- 1Department of Physics, Savitribai Phule Pune University, Pune, India
- 2Centre for Nanoscience and Nanotechnology, Amity University Maharashtra, Mumbai, India
The MoS2 nanobelts/Carbon hybrid nanostructure was synthesized by the simple hydrothermal method. The MoS2 nanobelts were distributed in the interlayers of Lemon grass-derived carbon (LG-C), provides the active sites and avoid restacking of the sheets. The structural and morphological characterization of MoS2/LG-C and LG-C were performed by Raman spectroscopy, X-ray diffraction, field emission scanning electron microscopy, transmission electron microscopy, and X-ray photoelectron spectroscopy. The electrochemical measurements were studied with cyclic voltammetry, the galvanostatic charge-discharge method, and electrochemical impedance spectroscopy. The specific capacitance of MoS2/LG-C and LG-C exhibits 77.5 F g−1 and 30.1 F g−1 at a current density of 0.5 A g−1. The MoS2/LG-C-based supercapacitor provided the maximum power density and energy density of 273.2 W kg−1 and 2.1 Wh kg−1, respectively. Furthermore, the cyclic stability of MoS2/LG-C was tested using charging-discharging up to 3,000 cycles, confirming only a 71.6% capacitance retention at a current density of 3 A g−1. The result showed that MoS2/LG-C is a superior low-cost electrode material that delivered a high electrochemical performance for the next generation of electrochemical energy storage.
1 Introduction
The development of energy storage devices, like rechargeable batteries, is important to produce environmentally friendly products (Huang et al., 2019; Shaqsi et al., 2020; Li et al., 2021). Nowadays, electrochemical energy storage devices such as supercapacitors and photovoltaic cells are commonly used (Kandasamy et al., 2021; Agrawal et al., 2022). Supercapacitors exhibit a quick charge storage property, which may contribute to their reduced charging times, greater cyclability, and therefore higher specific capacitance (Purkait et al., 2018; Narthana et al., 2021; Rajagopal et al., 2022; Rawat et al., 2022). The ongoing research effects focus on the production of a low-cost, long-life cycle, and high-specific capacitance material for the development of supercapacitors (Iro et al., 2016; Tomy et al., 2021). The selection of appropriate electrode material is important to improve the supercapacitor performance. Numerous materials have been used to store charges for electrochemical energy storage devices (Mohammed et al., 2019; Santoro et al., 2019). The store charges in supercapacitor devices are classified into three types, electrostatic double-layer capacitors, pseudocapacitors, and hybrid supercapacitors (Mathis et al., 2019; Atta and Fahim, 2021; Volfkovich, 2021). The double layer formed at the electrode surface is used for the storage of charges in electrostatic double-layer capacitors. In pseudocapacitors, charge storage occurs through a redox reaction (Fleischmann et al., 2022; Kour et al., 2022).
Biomass-derived carbon sources have natural renewable resources and are widely used because they are affordable, readily available, simple to prepare, and ecologically beneficial. Some examples of biomass that may be used as a resource for activated carbon for electrochemical energy storage include coconut oil, bamboo fiber, bean dregs, mango leaves, peanut shells, etc., (Madhu et al., 2014; Ruan et al., 2014; Gaddam et al., 2016; Ji et al., 2018; Bai et al., 2020) In contrast, activated carbons frequently exhibit less capacitive characteristics, such as low conductivity and restricted charge flow rates. Activated carbon exhibits poor conductivity and limited charge storage capacity (Gomes Ferreira de Paula et al., 2019; Mohammed et al., 2019; Santoro et al., 2019; Luo et al., 2021). To overcome these difficulties, it is necessary to develop structural modifications as well as hybrid materials (Khandare and Late, 2017; Tomboc et al., 2020).
The Lemon Grass (Cymbopogon citratus) plant is known for its long leaves, which are specially used for making oil and spices. In Asian countries, Lemon Grass (LG) is used to provide taste and flavor to drinks (including tea, coffee, etc.). Among them, it has been utilized as a biofertilizer and feedstock. LG consists mostly of cellulose, which is considered to be a carbon source material with high potential (Liakos et al., 2016; Thota et al., 2018). The production of carbon and inorganic-based composites is a successful method for enhancing electrochemical energy storage by combining various inorganic materials, including transition metal oxides, and transition metal dichalcogenides, with carbon-based materials (Wang et al., 2020; Kour et al., 2022).
Molybdenum (Mo) has a variable oxidation state that can vary from +2 to +6. Molybdenum disulfide (MoS2) has a layered structure that shows pseudocapacitive behaviour to obtain high specific capacitance (Cook et al., 2017). Mo has been stacked in between two sulfur atoms (S-Mo-S) by weak van der Waals forces, which allow the electrolyte ions to intercalate in MoS2 (Kukkar et al., 2016). The ion diffusion works with MoS2 to store the charge in its faradic capacitive nature, which helps to improve the chance of storing the charge (Mahmood et al., 2016).
In this work, we report the low-cost synthesis of MoS2/Lemon Grass-derived carbon (MoS2/LG-C) hybrid material for enhancing the electrochemical performance. In situ growth of MoS2 nanobelts on surfaces of the LG-C through a facile redox reaction between ammonium molybdate and thiourea with carbon. The morphology and structural properties of LG-C and MoS2/LG-C hybrid materials are examined. The electrochemical measurements performed with cyclic voltammetry, galvanostatic charge-discharge testing, and cyclic stability to obtain the superior charge storage capacity in supercapacitors are discussed.
2 Experimental
2.1 Materials
Ammonium heptamolybdate tetrahydrate ((NH4)6Mo7O24.4H2O), thiourea (NH2CSNH2), and potassium hydroxide (KOH) are procured from HPCL, India. All chemicals were AR-grade (99% purity) and used without further purification. Overall, double-distilled (DD) water was used in the experiments.
2.2 Preparation of LG-C
The lemon grass (LG) leaves were collected from the local market based in Pune, India. Collected LG leaves were washed with DD water and dried in the sunlight for at least 2 days. The dried leaves were crushed finely and subsequently treated in KOH (1:1 weight ratio of LG leaf powder to KOH) for impregnation. After impregnation, the pH of the powder has been adjusted to 7 (neutral) by using a 1M HCl solution. After adjusting the pH, the LG powder was dried for 12 h at 80°C. Finally, the powder was kept in tubular furnaces in an argon atmosphere at 800°C for 3 h. The temperature of the tubular furnace was increased slowly by 5°C per minute and cooled naturally to room temperature (RT).
2.3 Preparation of MoS2/LG-C
The preparation was initiated by dissolving 100 mg of LG-C in 50 mL of DD water. The ammonium heptamolybdate tetrahydride and thiourea in quantities of 151 mg and 200 mg were added to the above reaction mixture, and the mixture was stirred at room temperature for 2 h. The obtained mixture was poured into the Teflon link autoclave and treated hydrothermally at 220°C for 24 h before being allowed to cool down naturally to RT. The suspension was centrifuged and washed in deionized water and ethanol. The final product was dried in the oven for 12 h at 80°C.
2.4 Material characterization
The Raman spectra were recorded at a 532 nm He-Ne laser source using a Renishaw InVia Raman microscope. The crystalline phase and crystal structure of the pristine and hybrid materials were examined using X-ray diffraction (XRD) on a Bruker D8 Advance X-ray diffractometer using Cu Kα (= 1.5405 Å) irradiation. FESEM images were captured using the FEI Nova NANOSEM 450. X-ray photoelectron spectroscopy (XPS) was recorded on the PHI Versaprobe III by using Al Ka X-rays. Transmission electron microscopy (TEM), high resolution (HRTEM), and energy dispersive X-ray spectroscopy (EDS) were recorded using the FEI Talos F200S instrument at 200 kV.
2.5 Electrode preparation
The electrodes were prepared on carbon paper as a collector. A 1 × 1 cm2 area of carbon paper was coated with a slurry of active electrode material, carbon acetylene, and polyvinylidene fluoride (PVDF) in ratio of 85:10:5, respectively. PVDF is used as a binder in dimethylformamide (DMF). The electrodes were dried in a hot air oven for 12 h at 120°C. The active material in the quantity of 1 mg was used for the supercapacitor electrode fabrication.
2.6 Electrochemical measurement
All electrochemical measurements were carried out at the Biologic SP-300 potentiostat/galvanostat using a three-electrode set-up and symmetric system in 1M Li2SO4 electrolyte. Ag/AgCl, platinum and LG-C, and MoS2/LG-C were used as reference, counter, and working electrodes, respectively. Cyclic voltammetry (CV), the galvanostatic charge-discharge (GCD) test, electrochemical impedance spectroscopy (EIS), and cyclic stability using the GCD test were examined to study the various supercapacitor parameters.
3 Results and discussion
The one-step hydrothermal method was used to produce the MoS2/LG-C hybrid material. Figure 1 illustrates a schematic representation of the synthesis process of LG-C and MoS2/LG-C. LG-C nanosheets were associated with the formation of MoS2 nanobelts on the surfaces of LG-C. The structural information of MoS2 and the LG carbon was carried out using Raman spectroscopy and XRD analysis. Figure 2A shows the Raman spectra of LG-C and MoS2-LG-C. The two strong peaks appeared at about 1,344.3 and 1,592.8 cm−1 identified to the D and G vibrational modes of LG-C. The D band is associated with defects and disorders induced in sp2 carbon (Medhat et al., 2021). The Raman spectra of MoS2/LG-C show the E12g and A1g vibrational modes, which conform to the layered 2H-MoS2 structure (Dinh et al., 2021). The peak observed at 376.2 and 404.4 cm−1 shown in the inset of Figure 2A corresponds to the E12g and A1g modes of vibration containing in-plane and the plane-symmetric modes of Mo and S. The MoS2/LG-C Raman spectra revealed a shift in D and G vibrating modes occurring at 1,337.9 and 1,594.7 cm−1 which can be related to the stress caused in LG-C by the growth of MoS2 nanobelts. The intensity ratio of ID to IG is widely recognised to characterise the graphitization and amorphous nature of LG-C. In MoS2/LG-C, it was observed that the ID/IG ratio was approximately similar to 1:1 (Kishore et al., 2014). This indicates that higher disorder and more active sites in MoS2 allowed for the formation of amorphous carbon and defective sites in MoS2/LG-C (Yu et al., 2020).
Figure 2B shows the XRD patterns of the as-synthesized LG-C and MoS2/LG-C hybrid materials. The diffraction peaks observed in MoS2/LG-C at 2θ values of 14.4°, 39.2°, and 49.0° have indexed the planes (002), (103), and (105) respectively, corresponding to the 2H hexagonal phase of MoS2 (JCPDS no. 37–1492). The pristine LG-C XRD pattern revealed two peaks at 26.0° and 42.7°. The broad XRD peak was obtained for the pristine LG-C, while in MoS2/LG-C, these peaks were found to be slightly shifted to 25.8° and 41.9° due to the presence of MoS2 on the surface of LG-C (Shapira and Zucker, 2022).
FESEM images were taken at different magnifications, consisting of the micro-as well as nano-size surface morphology of pristine LG-C before and after the formation of MoS2. FESEM images of pristine LG-C, (Figures 3A–C) showed a thick sheet with a scattered layer appearance. LG-C sheets revealed a rough surface with the ordered stacking of the sheets. MoS2 nanobelts distributed in the interlayer of the LG-C materials (Figures 3D–F) produce nanobelts-like morphology. MoS2 has several precise nanobelts of sizes ranging from 2 to 3 μm in length with smooth and even surfaces.
Further, the surface morphology was investigated by TEM and HR-TEM images. The TEM images shown in Figures 4A–E further conform the size of nanobelts is not uniform, which varies from 90 to 190 nm. A clear dispersion of the MoS2 nanobelts in the LG-C matrix can be seen. The HR-TEM image (Figure 4F) shows a lattice spacing of ∼0.18 nm, which corresponds to the (105) plane of the hexagonal crystal structure of MoS2, which matches with the XRD results. The selected-area electron diffraction (SAED) pattern of the MoS2/LG-C is shown in the inset of Figure 4F. SAED pattern exhibited the polycrystalline nature of MoS2/LG-C hybrid material is revealed. Some of the prominent reflections (105) are shown in SAED. The elemental composition determined with the help of EDS analysis is given in (Supplementary Figure S1). The observed composition of Mo and S was found to close to the ideal conditions (1:2).
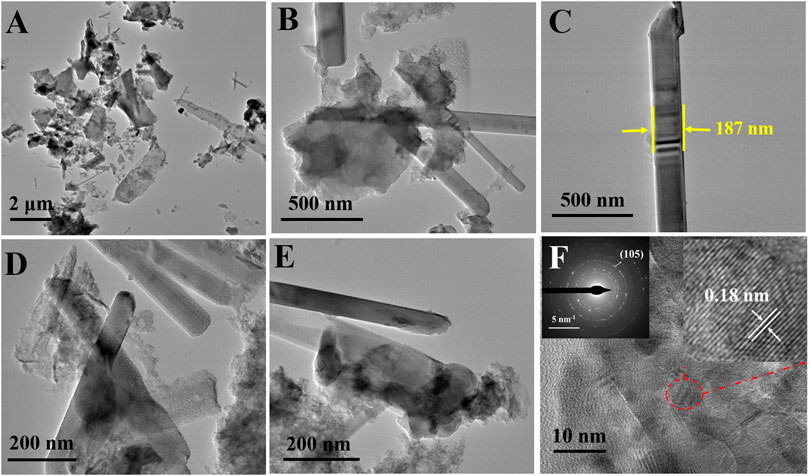
FIGURE 4. MoS2/LG-C hybrid material: (A–E) TEM images (F) HR-TEM image, inset of (F) showing SAED pattern and selected portion of lattice fringes.
The XPS spectrum of the MoS2/LG-C hybrid material is depicted in Figure 5. Figure 5A shows the survey scan for four peaks conforming to Mo 3d, S 2p, C 1s, and O 1s, suggesting that the MoS2/LG-C hybrid material was successfully formed. Apart from them, the peaks were obtained at 186.0 and 253.4 eV called plasmon loss peaks of S and Mo respectively. These peaks occur may be a higher probability of losing a specified amount of energy as a result of the photoelectron’s interaction with other electrons (Stevie and Donley, 2020). The doublet obtained 394.8 and 412.6 eV corresponds to Mo 3p3/2 and Mo 3p½ respectively. The Mo 3d XPS resolution scan (Figure 5B) shows the two primary peaks were obtained at 228.6 and 231.7 eV, corresponding to Mo4+ 3d5/2 and Mo4+ 3d3/2, respectively (Feng et al., 2019). The peaks obtained at 232.6 eV and 236.2 eV correspond to Mo6+ 3d5/2 and Mo6+ 3d3/2, respectively. The existence of sulphur atom level 2s in the MoS2/LG-C was linked to the peak at 225.7 eV (Xiong et al., 2015). Figure 5C shows the high-resolution S 2p XPS spectra, indicating the presence of peaks conforming to the S 2p3/2 and S 2p1/2 situated at 161.5 and 162.5 eV. The peak attributed to 168.9 eV could be the presence of the sulphate group (Gnanasekar et al., 2020). The C1s XPS spectra in Figure 5D revealed three major peaks with binding energies of about 284.3, 284.8, and 286.1, eV, corresponding to the functional groups C-C, C-C, and C-O, respectively (Ji et al., 2018).
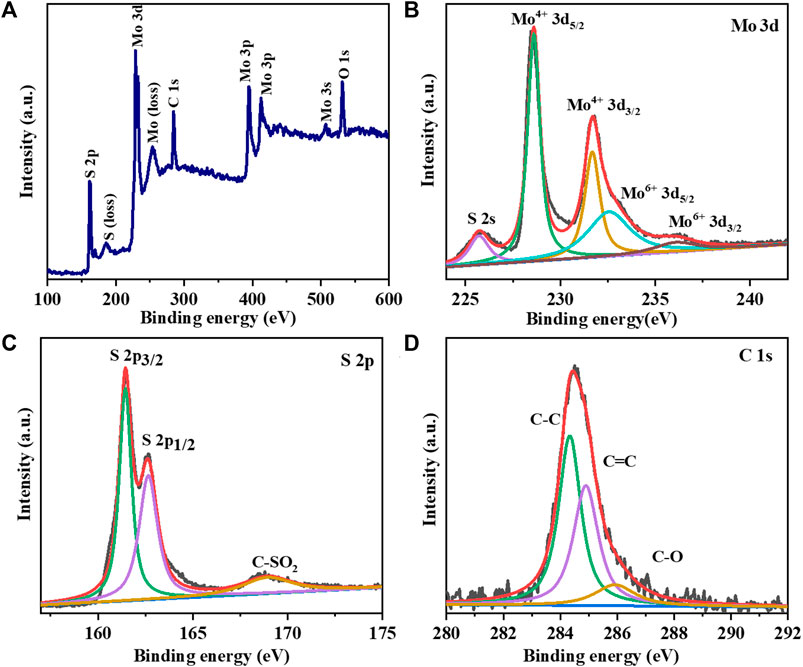
FIGURE 5. XPS analysis of MoS2/LG-C hybrid material: (A) Survey scan; High resolution XPS spectrum of (B) Mo 3d (C) S 2p (D) C 1s.
In Figure 6, all CV and GCD measurements were carried out using a 3-electrode system. Figures 6A, B shows the CV curves of LG-C and MoS2/LG-C in 1 M Li2SO4 at scan rates of 5, 10, 20, 50, and, 100 mV s−1. The CV curve of the LG-C electrode (Figure 6A), shows an almost rectangular nature with no identifiable redox peaks in the operating voltage range of 0.0–1.0 V. The rectangular nature of the CV curve of LG-C exhibits ideal behavior, showing the electric double-layer capacitor (Luo et al., 2015). MoS2/LG-C hybrid material in Figure 6B has a dome-like quasi-rectangular shape that exhibits both electric double-layer capacitor behaviour as well as Faradic pseudocapacitance behaviour (Xu et al., 2018). MoS2 nanobelts distributed in the interlayer of the LG-C sheets increased the entire area of the CV as well as peak current density with improved conductivity, which helps facilitate ion transport towards the electrochemical charge storage (Gopalakrishnan et al., 2020; Mahajan et al., 2022).
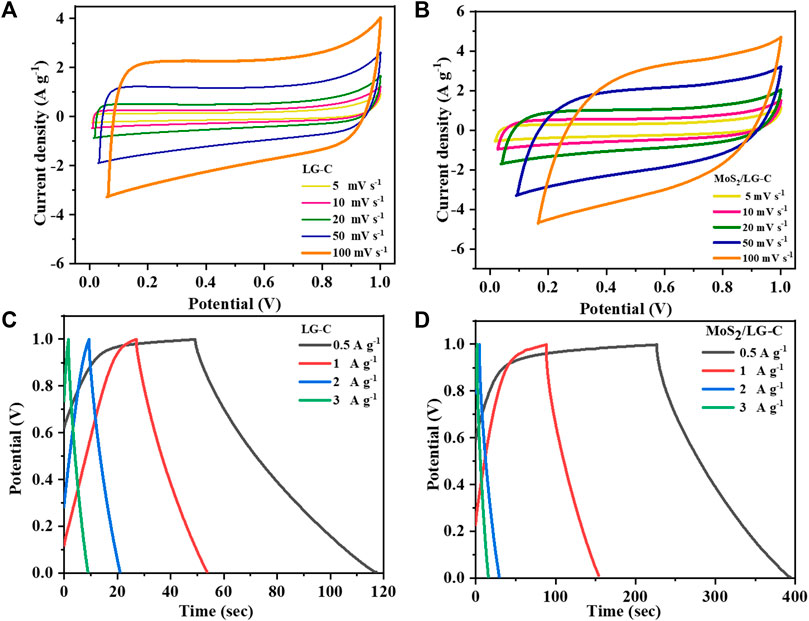
FIGURE 6. CV curves of (A) LG-C, (B) MoS2/LG-C; GCD curves of (C) LG-C, (D) MoS2/LG-C obtained by 3-electrode system.
Figures 6C, D show the GCD curves of LG-C and MoS2/LG-C at current densities of 0.5, 1, 2, and 3 A g−1 with the applied potential voltage between 0.0 and 1.0 V. The specific capacitance (Cs in F g−1) was calculated at various current densities using the following Eq. 1 (Lin and Zhang, 2015):
where
The Cs of LG-C and MoS2/LG-C calculate to be 30.5 F g−1 and 77.5 F g−1 at a current density of 0.5 A g−1, respectively. MoS2/LG-C helps to increase the specific capacitance value of the hybrid electrode material due to the synergistic effects of MoS2 as a conducting material with LG-C. Mo4+ in MoS2 supplied the more active sites for charge transfer in MoS2/LG-C, which provided a more interactive area between MoS2/LG-C and the electrolyte for ion diffusion and increased the discharge time (Feng et al., 2019). The comparative CV and GCD curves of LG-C and MoS2/LG-C are shown in Supplementary Figures S2A, B at a scan rate of 20 mV s−1 and a current density of 0.5 A g−1. Electrochemical charge storage is obtained due to ions present in an electrolyte (Li+) interaction through MoS2 and carbon sheets.
Figure 7A illustrates the plot of Cs versus the different current densities. At higher current densities, Cs were found to decrease, which could be due to the reduction in various activities at the electrode surface. The capacitance improvement occurs due to the favourable synergistic effect of MoS2 as an active-site hybrid material, which helps to avoid the stacking of LG-C sheets and increases the surface area of MoS2/LG-C. The increased surface area provided the path for electrochemical charge transformation and helped store the energy in the supercapacitor (Bi et al., 2019). The comparative data of different composite nanostructure materials with MoS2/LG-C in the literature related to Cs and various electrolytes are shown in Table 1. (Brousse et al., 2007; Aradilla et al., 2014; Ho et al., 2014; Masikhwa et al., 2017; Choi et al., 2020; Bello et al., 2022). The EIS measurements of LG-C and MoS2/LG-C were performed to study the transportation of ions in electrolytes with their electrochemical properties, equivalent series resistance (Rs), and charge transfer resistance (Rct) (Dulyaseree et al., 2017). Figure 7B shows the Nyquist plot of LG-C and MoS2/LG-C hybrid materials with an imaginary axis performed at a frequency range of 0.1 MHz–100 mHz. LG-C and MoS2/LG-C achieved similar series resistance (Rs) for supercapacitors of about 12.09 Ω and 6.26 Ω which shows the MoS2/LG-C has good electrical conductivity. In the high-frequency region, a clear semicircle was not observed, which indicates it may have a low Rct (Niaz et al., 2020). MoS2/LG-C has increased the conductivity of hybrid material because MoS2 provided a more active site for LG-C, which helps improve electrochemical properties. The cyclic stability of LG-C and MoS2/LG-C (Figure 7C was carried out using a GCD test in 1M Li2SO4 electrolyte at the current density of 3 A g−1. MoS2/LG-C exhibited superior cyclic stability and, 71.6% capacitance retaliation, and only 28.4% capacity degradation occurs up to 3,000 cycles at a current density of 3 A g−1. In LG-C, 46.2% of the capacitance was retained up to 3,000 cycles. In MoS2/LGC hybrid materials, sulphur bonded with MoS2 forms a layer that is collected on the carbon surface and connected to the carbon structure covalently, which helps to provide excellent cyclic stability. The abrupt decrease in capacity observed in cyclic stability plot due to the measurement might be related to active material dissolving in the electrolyte, as well as difficulties with electrodes which include expansion and active material loss owing to poor binding.
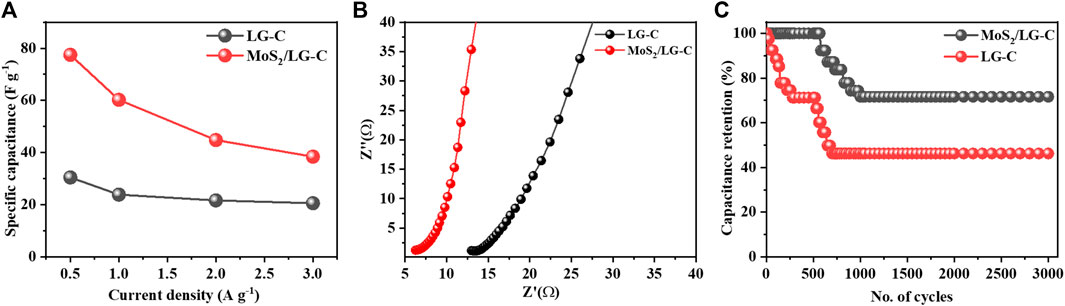
FIGURE 7. (A) Comparative plot of Cs at different current densities, (B) Nyquist plot of LG-C and MoS2/LG-C at the frequency range from 0.1 MHz to 100 mHz, (C) cyclic stability plot of LG-C and MoS2/LG-C hybrid material at current density 3 A g−1.
The electrochemical behaviour of the LG-C and MoS2/LG-C hybrid nanomaterials were also studied by symmetric supercapacitor devices in 1M Li2SO4 electrolyte. The CV curves of LG-C and MoS2/LG-C (Figures 8A, B) were measured at the potential window of 0.0–0.5 V at scan rates between 5 and 100 mVs−1. All CV curves of LG-C have a quasi-rectangular shape, showing a good electric double-layer capacitor for fast ion transfer. While CV curves variations from an ideal rectangle with increasing the current density. The GCD measurements of LG-C and MoS2/LG-C exhibit minor MoS2/LG-C were carried out at different current densities are shown in Figures 8C, D, respectively. The charge-discharge durations of the MoS2/LG-C hybrid material has longer than those of pure LG-C. The MoS2/LG-C hybrid material has higher specific capacitance than pure LG-C. The specific capacitance values were calculated using Eq. 2 for symmetric supercapacitor devices.
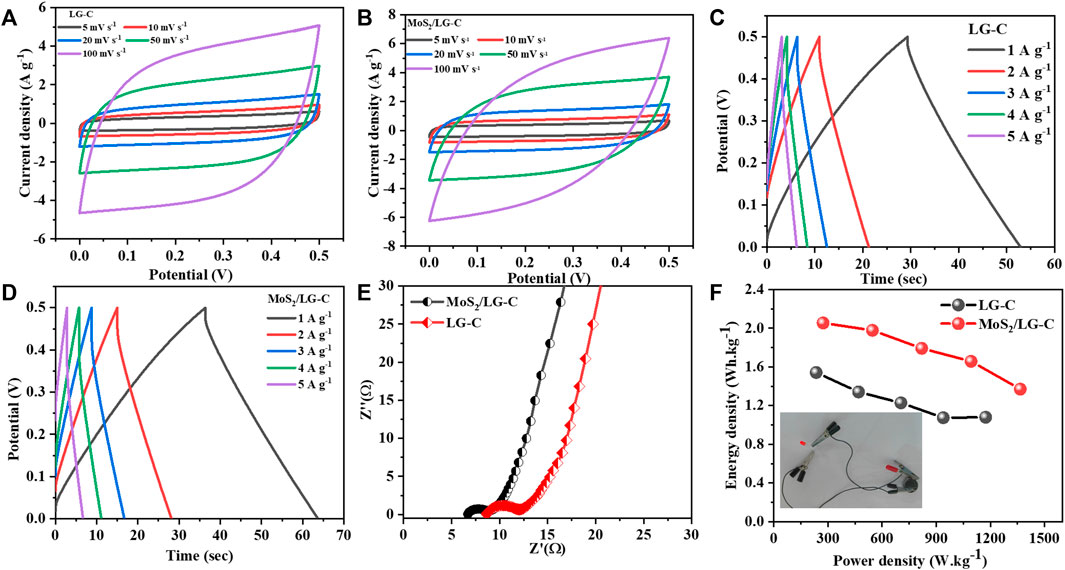
FIGURE 8. Symmetric supercapacitor device electrochemical measurements: (A) CV curves of LG-C, (B) CV curves of MoS2/LG-C, (C) GCD curves of LG-C, (D) GCD curves of MoS2/LG-C, (E) Nyquist plot of LG-C and MoS2/LG-C, (F) Ragone plot of LG-C and MoS2/LG-C, Inset of (F) shows the operating red light emitting diode for symmetric supercapacitor devices.
The MoS2/LG-C hybrid material and LG-C show a specific capacitance of 59.2 F g−1and 44.4 F g−1, respectively at a current density of 1 A g−1.
Furthermore, EIS measurements of MoS2/LG-C hybrid material and LG-C (Figure 8E) were carried out in the frequency range of 100 kHz to 10 mHz. The Nyquist plot corresponding series resistance (Rs) and charge transfer resistance (Rct) values of LG-C are 8.5 and 3.4 Ω respectively. In the MoS2/LG-C Nyquist plot, Rs and Rct were determined to be 6.6 and 2.2 Ω, respectively. The lower Rs value implies the internal resistance, electrolyte ionic resistance, and contact resistance of active electrode materials of the MoS2/LG-C electrode is more conductive than the LG-C electrode. The Rct may be ascribed to the MoS2/LG-C electrode’s improved electron transportation and high surface area, which allows for quick redox reactions at the electrode/electrolyte interface.
The Ragone plot of LG-C and MoS2/LG-C obtained at various current densities is depicted in Figure 8F. The relation between energy density (E in Wh kg−1) and power density (P in W kg−1) is an important parameter to examine energy devices. Energy density and power density are calculated from the following equations: 3 and 4, respectively (Raghu et al., 2018).
The MoS2/LG-C-based supercapacitor performance measured 273.2 W kg−1 power density and 2.1 Wh kg−1 energy density, whereas the LG-C sheets-based supercapacitor exhibited 234.7 W kg−1 power density and 1.5 Wh kg−1 energy density at a current density of 1 A g−1. P and E were found to increase with increasing current density, and the maximum power density, 1,366.1 W kg−1, and energy density, 1.3 Wh kg−1 were obtained at a current density of 5 A g−1 for MoS2/LG-C symmetric device. The inset of Figure 8F shows the operating red light-emitting diode for symmetric supercapacitor devices.
Conclusion
In conclusion, we successfully synthesized the MoS2 Nanobelts/LG-C nanohybrid structures by simple hydrothermal method. The low-cost and biodegradable LG leaves were used to form the nano-carbon by the pyrolysis method. The MoS2 nanobelts help to provide pathways for ion transportation due to the interaction between active electrode materials and electrolytes. The specific capacitances of MoS2/LG-C and LG-C were found to be 77.5 and 30.1 F g−1, respectively, at a current density of 0.5 A g−1. The cyclic stability of MoS2/LG-C and LG-C was carried out by the GCD test, and 71.6% capacitance retention occurs up to 3,000 cycles at the current density of 3 A g−1. The symmetric supercapacitor (MoS2/LG-C//MoS2/LG-C), the operating red light-emitting diode is illuminated. This method is favourable for the large-scale production of MoS2/LGC as active electrode hybrid materials for electrochemical energy storage devices.
Data availability statement
The original contributions presented in the study are included in the article/Supplementary Material.
Author contributions
LNK designed and performed the experiments as, characterizations and analyzed the data. DJL and NBC contributed to the analysis of the results and to the writing of the manuscript. All authors contributed to the article and approved the submitted version.
Acknowledgments
The authors are thankful to Savitribai Phule Pune University Pune, India for the financial support under the postdoctoral scheme. Authors like to thank Dr. H. S. S. Ramakrishna Matte and Dr. B. L. V. Prasad (Director), Centre for Nano and Soft Matter Sciences, Bangalore for TEM analysis work.
Conflict of interest
The authors declare that the research was conducted in the absence of any commercial or financial relationships that could be construed as a potential conflict of interest.
Publisher’s note
All claims expressed in this article are solely those of the authors and do not necessarily represent those of their affiliated organizations, or those of the publisher, the editors and the reviewers. Any product that may be evaluated in this article, or claim that may be made by its manufacturer, is not guaranteed or endorsed by the publisher.
Supplementary material
The Supplementary Material for this article can be found online at: https://www.frontiersin.org/articles/10.3389/fchem.2023.1166544/full#supplementary-material
References
Agrawal, A., Siddiqui, S. A., Soni, A., and Sharma, G. D. (2022). Advancements, frontiers and analysis of metal oxide semiconductor, dye, electrolyte and counter electrode of dye sensitized solar cell. Sol. Energy 233. doi:10.1016/j.solener.2022.01.027
Aradilla, D., Bidan, G., Gentile, P., Weathers, P., Thissandier, F., Ruiz, V., et al. (2014). Novel hybrid micro-supercapacitor based on conducting polymer coated silicon nanowires for electrochemical energy storage. Rsc Adv. 4. doi:10.1039/c4ra03192j
Atta, M. M., and Fahim, R. A. (2021). Flexible and wearable supercapacitors: A short review. J. Energy Storage 44, 103475. doi:10.1016/j.est.2021.103475
Bai, S., Wang, T., Tian, Z., Cao, K., and Li, J. (2020). Facile preparation of porous biomass charcoal from peanut shell as adsorbent. Sci. Rep. 10. doi:10.1038/s41598-020-72721-0
Bello, I. T., Otun, K. O., Nyongombe, G., Adedokun, O., Kabongo, G. L., and Dhlamini, M. S. (2022). Synthesis, characterization, and supercapacitor performance of a mixed-phase Mn-doped MoS2 nanoflower. Nanomaterials 12, 490. doi:10.3390/nano12030490
Bi, Z., Kong, Q., Cao, Y., Sun, G., Su, F., Wei, X., et al. (2019). Biomass-derived porous carbon materials with different dimensions for supercapacitor electrodes: A review. J. Mat. Chem. a 7. doi:10.1039/c9ta04436a
Brousse, T., Taberna, P.-L., Crosnier, O., Dugas, R., Guillemet, P., Scudeller, Y., et al. (2007). Long-term cycling behavior of asymmetric activated carbon/MnO2 aqueous electrochemical supercapacitor. J. Power Sources 173. doi:10.1016/j.jpowsour.2007.04.074
Choi, J. R., Lee, J. W., Yang, G., Heo, Y.-J., and Park, S.-J. (2020). Activated carbon/MnO2 composites as electrode for high performance supercapacitors. Catalysts 10, 256. doi:10.3390/catal10020256
Cook, J. B., Kim, H., Lin, T. C., Lai, C., Dunn, B., and Tolbert, S. H. (2017). Pseudocapacitive charge storage in thick composite MoS2 nanocrystal-based electrodes. Adv. Energy Mat. 7, 1601283. doi:10.1002/aenm.201601283
Dinh, D. A., Nguyen, T. L., Cuong, T. V., Hui, K. S., Bui, T. H., Wu, S., et al. (2021). Defect-free MoS2-flakes/amorphous-carbon hybrid as an advanced anode for lithium-ion batteries. Energy & Fuels 35. doi:10.1021/acs.energyfuels.0c03896
Dulyaseree, P., Fujishige, M., Yoshida, I., Toya, Y., Banba, Y., Tanaka, Y., et al. (2017). Nitrogen-rich green leaves of papaya and Coccinia grandis as precursors of activated carbon and their electrochemical properties. RSC Adv. 7. doi:10.1039/c7ra06048c,
Feng, N., Meng, R., Zu, L., Feng, Y., Peng, C., Huang, J., et al. (2019). A polymer-direct-intercalation strategy for MoS2/carbon-derived heteroaerogels with ultrahigh pseudocapacitance. Nat. Commun. 10. doi:10.1038/s41467-019-09384-7
Fleischmann, S., Zhang, Y., Wang, X., Cummings, P. T., Wu, J., Simon, P., et al. (2022). Continuous transition from double-layer to Faradaic charge storage in confined electrolytes. Nat. Energy 7. doi:10.1038/s41560-022-00993-z
Gaddam, R. R., Yang, D., Narayan, R., Raju, K., Kumar, N. A., and Zhao, X. S. (2016). Biomass derived carbon nanoparticle as anodes for high performance sodium and lithium ion batteries. Nano energy 26. doi:10.1016/j.nanoen.2016.05.047
Gnanasekar, P., Ranjith, K. S., Manivel, P., Han, Y.-K., and Kulandaivel, J. (2020). Hierarchical NbS2/MoS2-carbon nanofiber electrode for highly efficient and stable hydrogen evolution reaction at all ranges of pH. ACS Appl. Energy Mat. 3. doi:10.1021/acsaem.0c00856
Gomes Ferreira de Paula, F., Campello-Gómez, I., Ortega, P. F. R., Rodríguez-Reinoso, F., Martínez-Escandell, M., and Silvestre-Albero, J. (2019). Structural flexibility in activated carbon materials prepared under harsh activation conditions. Mater. (Basel) 12, 1988. doi:10.3390/ma12121988
Gopalakrishnan, A., Yu, A., and Badhulika, S. (2020). Facile synthesis of highly porous N-doped carbon nanosheets with silica nanoparticles for ultrahigh capacitance supercapacitors. Energy & Fuels 34. doi:10.1021/acs.energyfuels.0c02078
Ho, M. Y., Khiew, P. S., Isa, D., Tan, T. K., Chiu, W. S., Chia, C. H., et al. (2014). Nano Fe3O4-activated carbon composites for aqueous supercapacitors. Sains Malays. 43, 885–894.
Huang, S., Zhu, X., Sarkar, S., and Zhao, Y. (2019). Challenges and opportunities for supercapacitors. Apl. Mater 7, 100901. doi:10.1063/1.5116146
Iro, Z. S., Subramani, C., and Dash, S. S. (2016). A brief review on electrode materials for supercapacitor. Int. J. Electrochem. Sci. 11. doi:10.20964/2016.12.50
Ji, H., Hu, S., Shi, S., Guo, B., Hou, W., and Yang, G. (2018). Rapid microwave-hydrothermal preparation of few-layer MoS2/C nanocomposite as anode for highly reversible lithium storage properties. J. Mat. Sci. 53. doi:10.1007/s10853-018-2631-7
Kandasamy, M., Sahoo, S., Nayak, S. K., Chakraborty, B., and Rout, C. S. (2021). Recent advances in engineered metal oxide nanostructures for supercapacitor applications: Experimental and theoretical aspects. J. Mat. Chem. A 9. doi:10.1039/d1ta03857e
Khandare, L., and Late, D. J. (2017). MoO3-rGO nanocomposites for electrochemical energy storage. Appl. Surf. Sci. 418. doi:10.1016/j.apsusc.2016.11.199
Kishore, B., Shanmughasundaram, D., Penki, T. R., and Munichandraiah, N. (2014). Coconut kernel-derived activated carbon as electrode material for electrical double-layer capacitors. J. Appl. Electrochem. 44. doi:10.1007/s10800-014-0708-9
Kour, S., Tanwar, S., and Sharma, A. L. (2022). MnO2 nanorod loaded activated carbon for high-performance supercapacitors. J. Alloys Compd. 910, 164834. doi:10.1016/j.jallcom.2022.164834
Kukkar, M., Tuteja, S. K., Sharma, A. L., Kumar, V., Paul, A. K., Kim, K.-H., et al. (2016). A new electrolytic synthesis method for few-layered MoS2 nanosheets and their robust biointerfacing with reduced antibodies. ACS Appl. Mat. Interfaces 8. doi:10.1021/acsami.6b03079
Li, X., Zhang, J., Liu, B., and Su, Z. (2021). A critical review on the application and recent developments of post-modified biochar in supercapacitors. J. Clean. Prod. 310, 127428. doi:10.1016/j.jclepro.2021.127428
Liakos, I. L., D’autilia, F., Garzoni, A., Bonferoni, C., Scarpellini, A., Brunetti, V., et al. (2016). All natural cellulose acetate—lemongrass essential oil antimicrobial nanocapsules. Int. J. Pharm. 510. doi:10.1016/j.ijpharm.2016.01.060
Lin, S.-Y., and Zhang, X. (2015). Two-dimensional titanium carbide electrode with large mass loading for supercapacitor. J. Power Sources 294. doi:10.1016/j.jpowsour.2015.06.082
Luo, Q.-P., Huang, L., Gao, X., Cheng, Y., Yao, B., Hu, Z., et al. (2015). Activated carbon derived from melaleuca barks for outstanding high-rate supercapacitors. Nanotechnology 26, 304004. doi:10.1088/0957-4484/26/30/304004
Luo, X., Chen, S., Hu, T., Chen, Y., and Li, F. (2021). Renewable biomass-derived carbons for electrochemical capacitor applications. SusMat 1. doi:10.1002/sus2.8
Madhu, R., Sankar, K. V., Chen, S.-M., and Selvan, R. K. (2014). Eco-friendly synthesis of activated carbon from dead mango leaves for the ultrahigh sensitive detection of toxic heavy metal ions and energy storage applications. Rsc Adv. 4. doi:10.1039/c3ra45089a,
Mahajan, H., Mohanan, K. U., and Cho, S. (2022). Facile synthesis of biocarbon-based MoS2 composite for high-performance supercapacitor application. Nano Lett. 22. doi:10.1021/acs.nanolett.2c02595,
Mahmood, Q., Park, S. K., Kwon, K. D., Chang, S., Hong, J., Shen, G., et al. (2016). Transition from diffusion-controlled intercalation into extrinsically pseudocapacitive charge storage of MoS2 by nanoscale heterostructuring. Adv. Energy Mat. 6, 1501115. doi:10.1002/aenm.201501115
Masikhwa, T. M., Madito, M. J., Bello, A., Dangbegnon, J. K., and Manyala, N. (2017). High performance asymmetric supercapacitor based on molybdenum disulphide/graphene foam and activated carbon from expanded graphite. J. Colloid Interface Sci. 488. doi:10.1016/j.jcis.2016.10.095
Mathis, T. S., Kurra, N., Wang, X., Pinto, D., Simon, P., and Gogotsi, Y. (2019). Energy storage data reporting in perspective—Guidelines for interpreting the performance of electrochemical energy storage systems. Adv. Energy Mat. 9, 1902007. doi:10.1002/aenm.201902007
Medhat, A., El-Maghrabi, H. H., Abdelghany, A., Menem, N. M. A., Raynaud, P., Moustafa, Y. M., et al. (2021). Efficiently activated carbons from corn cob for methylene blue adsorption. Appl. Surf. Sci. Adv. 3, 100037. doi:10.1016/j.apsadv.2020.100037
Mohammed, A. A., Chen, C., and Zhu, Z. (2019). Low-cost, high-performance supercapacitor based on activated carbon electrode materials derived from baobab fruit shells. J. Colloid Interface Sci. 538. doi:10.1016/j.jcis.2018.11.103
Narthana, K., Durai, G., Kuppusami, P., Theerthagiri, J., Sujatha, S., Lee, S. J., et al. (2021). One-step synthesis of hierarchical structured nickel copper sulfide nanorods with improved electrochemical supercapacitor properties. Int. J. Energy Res. 45. doi:10.1002/er.6492
Niaz, N. A., Shakoor, A., Imran, M., Khalid, N. R., Hussain, F., Kanwal, H., et al. (2020). Enhanced electrochemical performance of MoS2/PPy nanocomposite as electrodes material for supercapacitor applications. J. Mat. Sci. Mat. Electron. 31. doi:10.1007/s10854-020-03682-3
Purkait, T., Singh, G., Kumar, D., Singh, M., and Dey, R. S. (2018). High-performance flexible supercapacitors based on electrochemically tailored three-dimensional reduced graphene oxide networks. Sci. Rep. 8. doi:10.1038/s41598-017-18593-3
Raghu, M. S., Kumar, K. Y., Rao, S., Aravinda, T., Prasanna, B. P., and Prashanth, M. K. (2018). Fabrication of polyaniline–few-layer MoS2 nanocomposite for high energy density supercapacitors. Polym. Bull. 75. doi:10.1007/s00289-017-2267-9
Rajagopal, S., Pulapparambil Vallikkattil, R., Mohamed Ibrahim, M., and Velev, D. G. (2022). Electrode materials for supercapacitors in hybrid electric vehicles: Challenges and current progress. Condens. Matter 7, 6. doi:10.3390/condmat7010006
Rawat, S., Mishra, R. K., and Bhaskar, T. (2022). Biomass derived functional carbon materials for supercapacitor applications. Chemosphere 286, 131961. doi:10.1016/j.chemosphere.2021.131961
Ruan, C., Ai, K., and Lu, L. (2014). Biomass-derived carbon materials for high-performance supercapacitor electrodes. Rsc Adv. 4. doi:10.1039/c4ra04470c,
Santoro, C., Winfield, J., Theodosiou, P., and Ieropoulos, I. (2019). Supercapacitive paper based microbial fuel cell: High current/power production within a low cost design. Bioresour. Technol. Rep. 7, 100297. doi:10.1016/j.biteb.2019.100297
Shapira, K., and Zucker, I. (2022). Emerging investigator series: Molybdenum disulfide-enabled activated carbon-a multifunctional adsorbent for practical water treatment applications. Environ. Sci. Nano 9. doi:10.1039/d1en00897h
Shaqsi, A. Z. A. L., Sopian, K., and Al-Hinai, A. (2020). Review of energy storage services, applications, limitations, and benefits. Energy Rep. 6. doi:10.1016/j.egyr.2020.07.028
Stevie, F. A., and Donley, C. L. (2020). Introduction to x-ray photoelectron spectroscopy. J. Vac. Sci. Technol. A Vac. Surfaces, Film. 38, 063204. doi:10.1116/6.0000412
Thota, S. P., Thota, S. M., Srimadh Bhagavatham, S., Sai Manoj, K., Sai Muthukumar, V. S., Venketesh, S., et al. (2018). Facile one-pot hydrothermal synthesis of stable and biocompatible fluorescent carbon dots from lemon grass herb. IET Nanobiotechnology 12. doi:10.1049/iet-nbt.2017.0038
Tomboc, G. M., Tesfaye Gadisa, B., Jun, M., Chaudhari, N. K., Kim, H., and Lee, K. (2020). Carbon transition-metal oxide electrodes: Understanding the role of surface engineering for high energy density supercapacitors. Chem. Asian J. 15. doi:10.1002/asia.202000324
Tomy, M., Ambika Rajappan, A., Vm, V., and Thankappan Suryabai, X. (2021). Emergence of novel 2D materials for high-performance supercapacitor electrode applications: A brief review. Energy & Fuels 35. doi:10.1021/acs.energyfuels.1c02743
Volfkovich, Y. M. (2021). Electrochemical supercapacitors (a review). Russ. J. Electrochem. 57. doi:10.1134/s1023193521040108
Wang, F., Ma, J., Zhou, K., and Li, X. (2020). MoS2/corncob-derived activated carbon for supercapacitor application. Mat. Chem. Phys. 244, 122215. doi:10.1016/j.matchemphys.2019.122215
Xiong, X., Luo, W., Hu, X., Chen, C., Qie, L., Hou, D., et al. (2015). Flexible membranes of MoS2/C nanofibers by electrospinning as binder-free anodes for high-performance sodium-ion batteries. Sci. Rep. 5. doi:10.1038/srep09254
Xu, W., Mu, B., and Wang, A. (2018). All-solid-state high-energy asymmetric supercapacitor based on natural tubular fibers. J. Mat. Sci. 53. doi:10.1007/s10853-018-2418-x
Keywords: lemon grass-derived carbon, MoS2, nanobelts, hybrid material, supercapacitor
Citation: Khandare LN, Late DJ and Chaure NB (2023) MoS2 nanobelts-carbon hybrid material for supercapacitor applications. Front. Chem. 11:1166544. doi: 10.3389/fchem.2023.1166544
Received: 15 February 2023; Accepted: 28 July 2023;
Published: 22 August 2023.
Edited by:
Umesha Mogera, University of Pennsylvania, United StatesReviewed by:
Kiruthika Shanmuga Sundaram, SASTRA University, IndiaGopalakrishnan Kothandam, University of Melbourne, Australia
Copyright © 2023 Khandare, Late and Chaure. This is an open-access article distributed under the terms of the Creative Commons Attribution License (CC BY). The use, distribution or reproduction in other forums is permitted, provided the original author(s) and the copyright owner(s) are credited and that the original publication in this journal is cited, in accordance with accepted academic practice. No use, distribution or reproduction is permitted which does not comply with these terms.
*Correspondence: Lina N. Khandare, bGluYWtoYW5kYXJlQGdtYWlsLmNvbQ==; Dattatray J. Late, ZGF0dGEwOTlAZ21haWwuY29t; Nandu B. Chaure, bi5jaGF1cmVAcGh5c2ljcy51bmlwdW5lLmFjLmlu
†ORCID ID: Lina N. Khandare, orcid.org/0000-0002-9978-3660; Dattatray J. Late, orcid.org/0000-0003-3007-7220; Nandu B. Chaure, orcid.org/0000-0002-4134-5019