- The Institute of Chemical Research of Catalonia (ICIQ), Tarragona, Spain
A self-assembled tetrahedral cage results from two C3-symmetry building blocks, namely, homooxacalix[3]arene tricarboxylate and uranyl cation, as demonstrated by X-ray crystallography. In the cage, four metals coordinate at the lower rim with the phenolic and ether oxygen atoms to shape the macrocycle with appropriate dihedral angles for tetrahedron formation, whereas four additional uranyl cations further coordinate at the upper-rim carboxylates to finalize the assembly. Counterions dictate the filling and porosity of the aggregates, whereas potassium induces highly porous structures, and tetrabutylammonium yields compact, densely packed frameworks. The tetrahedron metallo-cage complements our previous report (Pasquale et al., Nat. Commun., 2012, 3, 785) on uranyl–organic frameworks (UOFs) from calix[4]arene and calix[5]arene carboxylates (octahedral/cubic and icosahedral/dodecahedral giant cages, respectively) and completes the assembly of all five Platonic solids from just two chemical components.
Introduction
Metals have been key players since the birth of supramolecular chemistry, not only for their role as templates in the generation of macrocyclic compounds of different shapes and sizes but also for producing and being incorporated into a wide range of self-assembled structural motifs such as helicates or grids of increasing complexity and controlled topologies (i.e., molecules whose representation/graph based on atoms and bonds is non-planar), like catenanes, rotaxanes, knots, molecular muscles, and machines, many of them pioneered by Prof. Jean-Pierre Sauvage and thoroughly described in his Nobel Lecture (Sauvage, 2017). In the context of metallosupramolecular chemistry, metal-mediated self-assembled spheres or polyhedral cages have gained increased attention, owing to their nanoscale cavities. Work in this area has mostly focused on building their architectures and studying the properties and applications of their confined nanospaces. The topic has been extensively reviewed over the last four decades, and some recent studies include Pullen et al. (2021), McConnell (2022), and McTernan et al. (2022).
Design principles to construct high-symmetry cages, such as tetrahedra, cubes, and octahedra, were first discussed by Stang and co-workers (Chakrabarty et al., 2011) and are based on the shapes of the ligands employed and the coordination angles of the metals involved. The ample choice of available metal candidates for self-assembly must be balanced by the rigidity of their coordination requirements, much higher than other tools such as hydrogen bonds or hydrophobic forces. For example, in Fujita’s MnL2n (M = Pd2+) giant nanoscopic metallo-cages (Sun et al., 2011), subtle variations in the ligand (furan versus thiophene) favor either M12L24 or M24L48 rhombicuboctahedral constructs. In addition, the self-assembly process must be reversible to reach equilibrium, so the most stable structures result. Thus, kinetically labile octahedral (i.e., GaIII, FeII, CoII, ZnII, and NiII) (Caulder and Raymond, 1999) and square planar (PdII and PtII) metal ions (Sun et al., 2020) are usually employed. Among the larger metals, lanthanides (i.e., EuIII) have also been used for self-assembly, but their variable coordination numbers and geometries complicate rational designs (Yan et al., 2015). In the actinide series, uranyl cation UO22+ has been employed to assemble frameworks and cages, but most examples generally involve polyoxometalate-type clusters (Burns et al., 2005; Ling et al., 2010; Thuéry and Harrowfield, 2017).
Among the self-assembled cages, the chemical replica of Platonic solids, the five regular polyhedra with convex faces, have always attracted and fascinated synthetic chemists. While polyhedral structures based on sp3-carbon atoms are limited to tetrahedrane, cubane, and dodecahedrane, whose syntheses constituted milestone achievements decades ago (Eaton and Cole, 1964; Maier et al., 1978; Ternansky et al., 1982), coordination and supramolecular chemistry have opened access to structures of increased complexity (Seidel and Stang, 2002; Pluth and Raymond, 2007; Yoshizawa et al., 2009; Hardie, 2010; Jin et al., 2010; Smulders et al., 2013; Young and Hay, 2013), such as octahedra (MacGillivray and Atwood, 1997; Takeda et al., 1999; Ronson et al., 2007; Hiraoka et al., 2009), icosahedra (Orr et al., 1999; Bilbeisi et al., 2013), tetrahedra (Pluth et al., 2007; Mal et al., 2009; Granzhan et al., 2011; Clustelcean et al., 2012; Mahata et al., 2013; Mitra et al., 2013), or so-called Archimedean solids (Olenyuk et al., 1999; Sun et al., 2010; Liu et al., 2011; Wang et al., 2014).
We described, a decade ago, novel metallo-cages in the solid state arising from uranyl cation UO22+ and calixarene carboxylic acids (Pasquale et al., 2012). Uranyl easily coordinates reversibly with three carboxylates at its equatorial plane in a hexagonal bipyramidal fashion (Clark et al., 1995; Sather et al., 2010; Wang and Chen, 2011), providing an ideal C3-symmetry component (Figure 1A), whereas calix[4]arene and calix[5]arene carboxylates provide C4- and C5-symmetry elements, respectively, to build octahedral and icosahedral assemblies (with an inner cube or dodecahedron inscribed at the uranyl -yl oxygens, respectively) (Figures 1B, C). Indeed, polycarboxylates have been often employed as ligands for uranyl–organic frameworks (UOFs) (Thuéry et al., 1999; Thuéry et al., 2004; Liao et al., 2010; Wang and Chen, 2011; Li et al., 2016; Zhang et al., 2017; Hu et al., 2018) with a wide variety of resulting architectures.
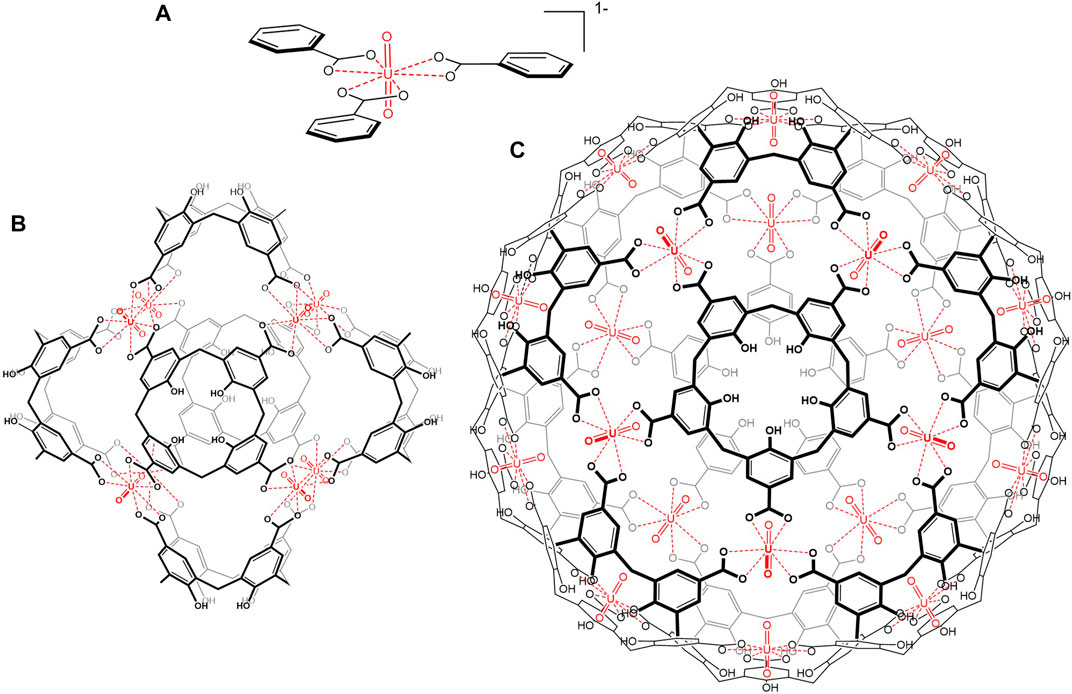
FIGURE 1. Hexagonal bipyramidal coordination of uranyl with carboxylates (A) and chemical structures of uranyl hexameric (B) and dodecameric (C) cages from calixarenes.
As a result, octahedral and icosahedral anionic metallo-cages of nanoscopic dimensions (estimated inner volumes from inscribed spheres ca. 940 and 7,200 Å3, respectively) were formed univocally with an unusually small number of components (Pasquale et al., 2012).
The assembly of a tetrahedron, the last Platonic solid from calixarene carboxylic acids and uranyl, requires two C3-symmetry components in a L4M4 stoichiometry, but the selection of an appropriate ligand with three carboxylates is by no means trivial. Calix[6]arenes substituted at alternate rings are unsuitable candidates, since these substituents are oriented almost parallel to each other in their conformations (van Duynhoven et al., 1994). An interesting alternative would be the use of O-unsubstituted homooxacalix[3]arenes, but they display wide, almost flat cone conformations (Tsubaki et al., 1998). Interestingly, however, the cavities can shrink upon uranyl coordination with the six oxygen atoms of the macrocycle (three phenols and three ether bridges) (Thuéry et al., 1999; Masci et al., 2002) into ideal angles and shapes for a tetrahedral assembly.
Based on these findings, a cage of L4M8 stoichiometry could be anticipated from homooxacalix[3]arene tricarboxylic acid 1 (Scheme 1), with four uranyl cations (in blue) at the lower rim of the ligands (shaping metals) and the other four metals (in red) acting as gluing elements.
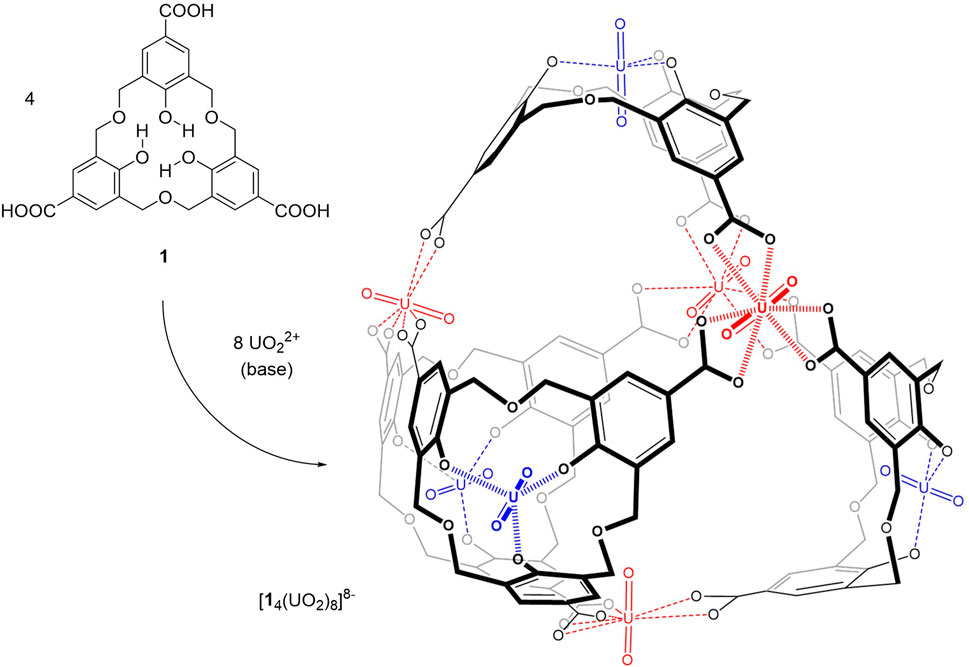
SCHEME 1. Assembly of a tetrahedral cage from homooxacalix[3]arene tricarboxylic acid (1) and uranyl.
Results and discussion
The predicted shaping of homooxacalix[3]arene–uranyl complexes into an ideal building block for tetrahedron formation was confirmed in the solid state from the triester precursor of triacid 1, namely, triethyl homooxacalix[3]arene tricarboxylate (2) (Zhong et al., 1999). Indeed, a single crystal (crystal C1) was grown from the slow diffusion of ethyl acetate into a mixture of 2, potassium tert-butoxide, and uranyl nitrate in a CHCl3–methanol–DMF solvent mixture. In the crystal, the uranyl is bound to all three phenol groups of the macrocycle, at the expected long U-O single-bond distances (ca. 2.22 Å) (Thuéry et al., 1999), forcing the calixarene skeleton to adopt a sharp conical bowl-shaped conformation (dihedral angles of benzene rings at ca. 85.3o) (Figure 2; Supplementary Figure S1). The remaining ether oxygen atoms of the macrocycle also coordinate at the uranyl equatorial plane, though in a much weaker manner (U-O distances ca. 3.19 Å).
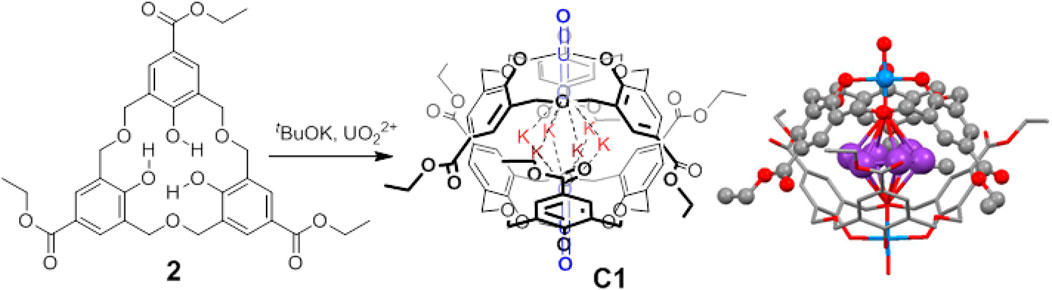
FIGURE 2. Synthesis and crystal structure of uranyl complex C1 from triethyl homooxacalix[3]arene tricarboxylate ester (2).
Interestingly, in the crystal packing, C1 forms a dimeric capsule, arising from two staggered, face-to-face oriented bowls and stabilized by the disordered potassium counterions of both units, bound to the inner -yl oxygen atoms of the uranyl moieties, and also stabilized by cation–π interactions with the neighboring benzene rings (Gokel et al., 2002).
Tricarboxylic acid 1 was readily synthesized by the hydrolysis of 2 with potassium hydroxide in an ethanol–water mixture. Slow diffusion of toluene into a solution of 1, potassium hydroxide, and uranyl nitrate in DMF resulted in a crystal showing the expected supramolecular assembly. The structure was resolved using a rotating anode with MoKa radiation, without requiring the use of a synchrotron light beam, as is usual for most giant assemblies (Sun et al., 2010; Sun et al., 2011).
The tetrahedral complex 14(UO2)8K8 (crystal C2) crystallizes in the body-centered cubic space group I-43m, showing a high degree of symmetry. Four uranyl residues are located at the center of the faces, whereas the remaining four metals lie at the corners (Figure 3). Unlike for crystal C1, the calixarene monomers are not fully symmetric, revealing a certain degree of distortion, as only two of the ether oxygen atoms bind to the uranyl (Supplementary Figure S2B). The inner -yl oxygens of the uranyl groups at the faces define a tetrahedron with two ca. 7.70 Å edges and four ca. 7.93 Å edges, whereas a larger tetrahedron (Figure 3B; Supplementary Figure S3) is defined by the prolongation of the lines along the edges of the assembly.
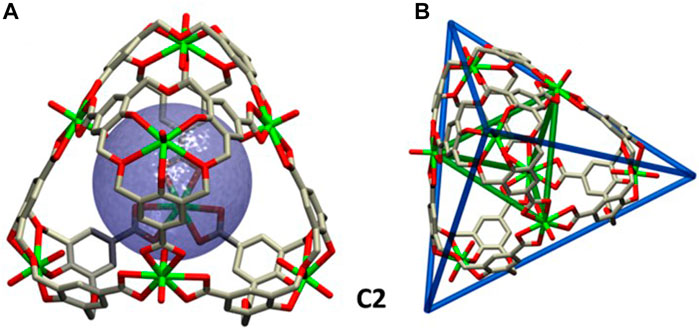
FIGURE 3. Crystal structure C2. (A) Tetrahedron frame with a ca. 382 Å3 sphere tangent to the -yl oxygens at the faces, representing the inner available volume. (B) Wireframe representation of the two distorted tetrahedrons defined by the inner -yl uranyl oxygens on the face (green lines) and by the outer tetrahedral surface (blue lines).
The crystal packing of C2 reveals a remarkable degree of porosity. Each tetrahedron requires eight cationic counterions to balance the overall negative charge (one negative charge per uranyl subunit). In crystal C2, only four disordered potassium cations have been assigned, located as a bridge connecting to three tetrahedra via the outer -yl uranyl oxygens at the corners (Figure 4A).
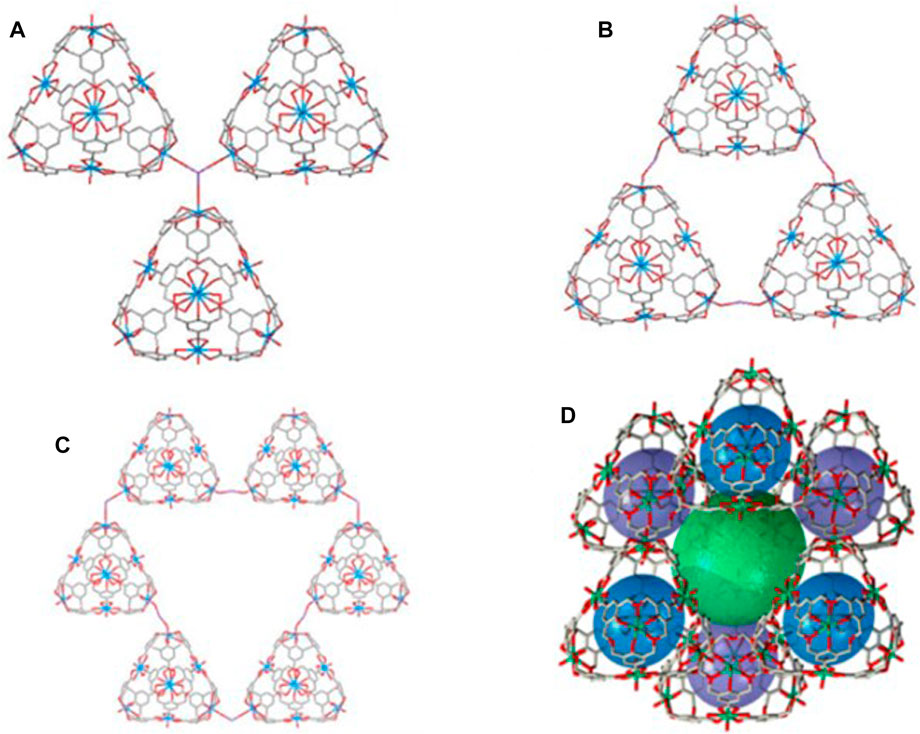
FIGURE 4. Stacking mode between tetrahedral cages and bridged potassium counterions in C2. (A) Each potassium bridges three tetrahedral cages. (B) Three tetrahedra form a circle via three bridged potassium cations. (C) Six tetrahedra in a circle via six bridged potassium atoms. (D) Self-assembled cavity (in green) formed by six tetrahedral cages with a ca. 882 Å3 volume.
The three-dimensional stacking of tetrahedra and potassium counterions in C2 is based on triple-tetrahedral subunits and a sextuple-tetrahedral subunit formed through potassium bridges (Figures 4A–C). Two triple-tetrahedral subunits are formed: one via a bridged potassium atom and the other as a circle that requires three bridged potassium counterions. Also, a larger, flat, cyclic subunit is formed by six tetrahedrons and six bridged potassium atoms. Stacking of both triple-tetrahedral assemblies produces a large tetrahedral cavity, whose potassium vertexes define 19.56 Å edges and an inner available volume of ca. 882 Å3 (Figure 4D; Supplementary Figure S4). The complexity further increases by the formation of a cage with 12 tetrahedra, in which the sextuple-tetrahedral assembly cage is embedded (Supplementary Figure S5). The 3D tetrahedron–potassium network in C2 can be displayed by using layer-by-layer stacking and mutually embedding patterns (Supplementary Figure S6).
When ethyl acetate is diffused into a mixture of 1, tetrabutylammonium (TBA) hydroxide, and uranyl nitrate, a homooxacalix[3]arene–uranyl tetrahedral complex (crystal C3) is also formed. The complex with TBA as a counterion crystalizes in space group P2 (1)/n, a lower symmetry than crystal C2, so that the tetrahedral cage lacks symmetry elements. In this case, all eight cationic TBA counterions are located. Two of them, together with three solvent molecules (DMF), fulfill the cavity (Figure 5A). The remaining six TBA counterions surround the tetrahedral cage in a tight packing, so a porous assembly is not present under these conditions (Figure 5B). This is rather unusual in other chemical replica of Platonic tetrahedra (Granzhan et al., 2011; Clustelcean et al., 2012).
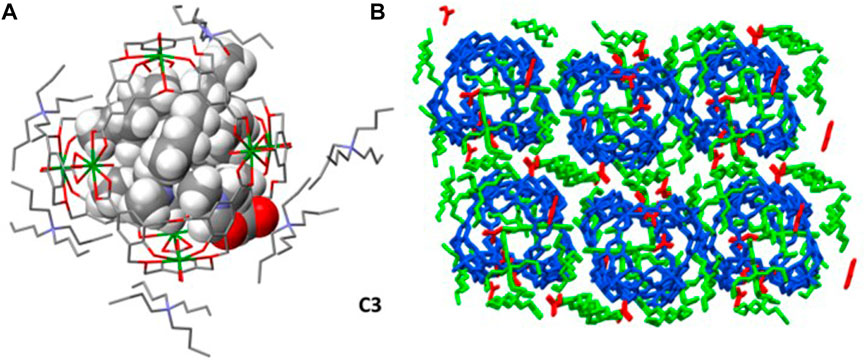
FIGURE 5. (A) Two TBAs and three DMFs filling the tetrahedral cage, whereas six TBAs surround the cage in C3. (B) Packing of tetrahedral cages, TBA counterion, and DMF solvent molecules.
Summary and outlook
In summary, all five Platonic solids can be easily assembled from just two components, namely, calixarene carboxylates and uranyl. The last one, the tetrahedron (representing fire in Plato’s conception of world), is described here. The assembly requires homooxacalix[3]arene tricarboxylate and eight uranyl moieties, four of them employed to shape the macrocycle into the appropriate conformation, while the remaining four are gluing elements to bridge the subunits by carboxylate–uranyl coordination. Counterions dictate the packing characteristics. Potassium creates porous materials, whereas tetrabutylammonium yields densely packed structures. As for the remaining uranyl cages, metals are centered in the faces, paving the way for the use of these novel UOFs as catalytic vessels (Hu et al., 2018), gas storage containers (Furukawa et al., 2010; Li et al., 2016), and in photoelectronic applications (Wang and Chen, 2011; Wang et al., 2012; Wang et al., 2013).
From a design point of view, our approach to Platonic polyhedral cages could be conceptually extended to reversible non-metallic motifs, such as imines, from calixarene aldehydes and planar C3-symmetry counterparts (i.e., benzene or 1,3,5-triazine triamines) (Rue et al., 2011; Lin et al., 2012), opening the way for a new family of self-assembled large capsules.
Data availability statement
The datasets presented in this study can be found in online repositories. The names of the repository/repositories and accession number(s) can be found below: http://www.crystallography.net/cod/-, 3000431, 3000432, and 3000433.
Author contributions
JdM conceived the project and wrote the original draft of the manuscript. J-CW performed the synthesis, purification, and characterization of the products. EE-A and MM-B realized the X-ray analysis and wrote the draft of the crystallographic data and analysis.
Funding
This work was supported by the Institute of Chemical Research of Catalonia (ICIQ), Centres de Recerca de Catalunya (CERCA), the Barcelona Institute of Science and Technology (BIST), the Ministry of Economy and Competitiveness of Spain (Project CTQ2011-28677), and Consolider Ingenio 2010 (Grant CSD2006-0003).
Conflict of interest
The authors declare that the research was conducted in the absence of any commercial or financial relationships that could be construed as a potential conflict of interest.
Publisher’s note
All claims expressed in this article are solely those of the authors and do not necessarily represent those of their affiliated organizations, or those of the publisher, the editors, and the reviewers. Any product that may be evaluated in this article, or claim that may be made by its manufacturer, is not guaranteed or endorsed by the publisher.
Supplementary material
The Supplementary Material for this article can be found online at: https://www.frontiersin.org/articles/10.3389/fchem.2023.1163178/full#supplementary-material
References
Bilbeisi, R. A., Ronson, T. K., and Nitschke, J. R. (2013). A self-assembled [FeII12L12] capsule with an icosahedral framework. Angew. Chem. Int. Ed. 52 (34), 9027–9030. doi:10.1002/anie.201302976
Burns, P. C., Kubatko, K.-A., Sigmon, G., Fryer, B. J., Gagnon, J. E., Antonio, M. R., et al. (2005). Actinyl peroxide nanospheres. Angew. Chem. Int. Ed. 44 (14), 2135–2139. doi:10.1002/anie.200462445
Caulder, D. L., and Raymond, K. N. (1999). Supermolecules by design. Acc. Chem. Res. 32 (11), 975–982. doi:10.1021/ar970224v
Chakrabarty, R., Mukherjee, P. S., and Stang, P. J. (2011). Supramolecular coordination: Self-assembly of finite two- and three-dimensional ensembles. Chem. Rev. 111 (11), 6810–6918. doi:10.1021/cr200077m
Clark, D. L., Hobart, D. E., and Neu, M. P. (1995). Actinide carbonte complexes and their importance in actinide environmental chemistry. Chem. Rev. 95 (1), 25–48. doi:10.1021/cr00033a002
Clustelcean, R., Bonnesen, P. V., Ducan, N. C., Zhang, X., Watson, L. A., Berkel, G. V., et al. (2012). Urea-functionalized M4L6 cage receptors: Anion-templated self-assembly and selective guest exchange in aqueous solutions. J. Am. Chem. Soc. 134 (20), 8525–8534. doi:10.1021/ja300677w
Eaton, P. E., and Cole, T. W. (1964). The cubane system. J. Am. Chem. Soc. 86 (5), 962–964. doi:10.1021/ja01059a072
Furukawa, H., Ko, N., Go, Y. B., Aratani, N., Choi, S. B., Choi, E., et al. (2010). Ultrahigh porosity in metal-organic frameworks. Science 329 (5990), 424–428. doi:10.1126/science.1192160
Gokel, G. W., Barbour, L. J., Ferdani, R., and Hu, J. (2002). Lariat ether receptor systems show experimental evidence for alkali metal Cation−π interactions. Acc. Chem. Res. 35 (10), 878–886. doi:10.1021/ar000093p
Granzhan, A., Schouwey, C., Riis-Johannessen, T., Scopelliti, R., and Severin, K. (2011). Connection of metallamacrocycles via dynamic covalent chemistry: A versatile method for the synthesis of molecular cages. J. Am. Chem. Soc. 133 (18), 7106–7115. doi:10.1021/ja200580x
Hardie, M. J. (2010). Recent advances in the chemistry of cyclotriveratrylene. Chem. Soc. Rev. 39, 516–527. doi:10.1039/b821019p
Hiraoka, S., Yamauchi, Y., Arakane, R., and Shionoya, M. (2009). Template-directed synthesis of a covalent organic capsule based on a 3 nm-sized metallocapsule. J. Am. Chem. Soc. 131 (33), 11646–11647. doi:10.1021/ja903324r
Hu, K.-Q., Huang, Z.-W., Zhang, Z.-H., Mei, L., Qian, B.-B., Yu, J.-P., et al. (2018). Actinide-based porphyrinic MOF as a dehydrogenation catalyst. Catal. Chem. Eur. J. 24 (63), 16766–16769. doi:10.1002/chem.201804284
Jin, P., Dalgarno, S. J., and Atwood, J. L. (2010). Mixed metal-organic nanocapsules. Coord. Chem. Rev. 254 (15-16), 1760–1768. doi:10.1016/j.ccr.2010.04.009
Li, P., Vermeulen, N. A., Gong, X., Malliakas, C. D., Stoddart, J. F., Hupp, J. T., et al. (2016). Design and synthesis of a water-stable anionic uranium-based metal–organic framework (MOF) with ultra large pores. Angew. Chem. Int. Ed. 55 (35), 10358–10362. doi:10.1002/anie.201605547
Liao, Z.-L., Li, G.-D., Wei, X., Yu, Y., and Chen, J.-S. (2010). Construction of three-dimensional uranyl - organic frameworks with benzenetricarboxylate ligands. Eur. J. Inorg. Chem. 2010, 3780–3788. doi:10.1002/ejic.201000298
Lin, Z., Sun, J., Efremovska, B., and Warmuth, R. (2012). Assembly of water-soluble, dynamic, covalent container molecules and their application in the room-temperature stabilization of protoadamantene. Chem. Eur. J. 18 (40), 12864–12872. doi:10.1002/chem.201200602
Ling, J., Qiu, J., Sigmon, G. E., Ward, M., Szymanowski, J. E. S., and Burns, P. C. (2010). Uranium pyrophosphate/methylenediphosphonate polyoxometalate cage clusters. J. Am. Chem. Soc. 132 (38), 13395–13402. doi:10.1021/ja1048219
Liu, Y., Hu, C., Comotti, A., and Ward, M. D. (2011). Supramolecular archimedean cages assembled with 72 hydrogen bonds. Science 333 (6041), 436–440. doi:10.1126/science.1204369
MacGillivray, L. R., and Atwood, J. L. (1997). A chiral spherical molecular assembly held together by 60 hydrogen bonds. Nature 389, 469–472. doi:10.1038/38985
Mahata, K., Frischmann, P. D., and Würthner, F. (2013). Giant electroactive M4L6 tetrahedral host self-assembled with Fe(II) vertices and perylene bisimide dye edges. J. Am. Chem. Soc. 135 (41), 15656–15661. doi:10.1021/ja4083039
Maier, G., Pfriem, S., Schäfer, U., and Matusch, R. (1978). Tetra-tert-butyltetrahedrane. Angew. Chem. Int. Ed. 17 (7), 520–521. doi:10.1002/anie.197805201
Mal, P., Breiner, B., Rissanen, K., and Nitschke, J. R. (2009). White phosphorus is air-stable within a self-assembled tetrahedral capsule. Science 324 (5935), 1697–1699. doi:10.1126/science.1175313
Masci, B., Nierlich, M., and Thuéry, P. (2002). Supramolecular assemblies from uranyl ion complexes of hexahomotrioxacalix[3]arenes and protonated [2.2.2]cryptand. New J. Chem. 26 (6), 766–774. doi:10.1039/b200734g
McConnell, A. J. (2022). Metallosupramolecular cages: From design principles and characterisation techniques to applications. Chem. Soc. Rev. 51 (8), 2957–2971. doi:10.1039/d1cs01143j
McTernan, C. T., Davies, J. A., and Nitschke, J. R. (2022). Beyond platonic: How to build metal-organic polyhedra capable of binding low-symmetry, information-rich molecular cargoes. Chem. Rev. 122 (11), 10393–10437. doi:10.1021/acs.chemrev.1c00763
Mitra, T., Jelfs, K. E., Schmidtman, M., Ahmed, A., Chong, S. Y., Adams, D. J., et al. (2013). Molecular shape sorting using molecular organic cages. Nat. Chem. 5, 276–281. doi:10.1038/nchem.1550
Olenyuk, B., Levin, M. D., Whiteford, J. A., Shield, J. E., and Stang, P. J. (1999). Self-assembly of nanoscopic dodecahedra from 50 predesigned components. J. Am. Chem. Soc. 121 (44), 10434–10435. doi:10.1021/ja9931933
Orr, G. W., Barbour, L. J., and Atwood, J. L. (1999). Controlling molecular self-organization: Formation of nanometer-scale spheres and tubules. Science 285 (5430), 1049–1052. doi:10.1126/science.285.5430.1049
Pasquale, S., Sattin, S., Escudero-Adán, E. C., Martínez-Belmonte, M., and de Mendoza, J. (2012). Giant regular polyhedra from calixarene carboxylates and uranyl. Nat. Commun. 3, 785. doi:10.1038/ncomms1793
Pluth, M. D., Bergman, R. G., and Raymond, K. N. (2007). Acid catalysis in basic solution: A supramolecular host promotes orthoformate hydrolysis. Science 316 (5821), 85–88. doi:10.1126/science.1138748
Pluth, M. D., and Raymond, K. N. (2007). Reversible guest exchange mechanisms in supramolecular host–guest assemblies. Chem. Soc. Rev. 36, 161–171. doi:10.1039/b603168b
Pullen, S., Tessarolo, J., and Clever, G. H. (2021). Increasing structural and functional complexity in self-assembled coordination cages. Chem. Sci. 12 (21), 7269–7293. doi:10.1039/d1sc01226f
Ronson, T. K., Fisher, J., Harding, L. P., and Hardie, M. J. (2007). Star-burst prisms with cyclotriveratrylene-type ligands: A [Pd6L8]12+ stella octangular structure. Angew. Chem. Int. Ed. 46 (47), 9086–9088. doi:10.1002/anie.200703903
Rue, N. M., Sun, J., and Warmuth, R. (2011). Polyimine container molecules and nanocapsules. Isr. J. Chem. 51 (7), 743–768. doi:10.1002/ijch.201100064
Sather, A. C., Berryman, O. B., and Rebek, J. (2010). Selective recognition and extraction of the uranyl ion. J. Am. Chem. Soc. 132 (39), 13572–13574. doi:10.1021/ja1035607
Sauvage, J.-P. (2017). From chemical topology to molecular machines (Nobel lecture). Angew. Chem. Int. Ed. 56 (37), 11080–11093. doi:10.1002/anie.201702992
Seidel, S. R., and Stang, P. J. (2002). High-symmetry coordination cages via self-assembly. Acc. Chem. Res. 35 (11), 972–983. doi:10.1021/ar010142d
Smulders, M. M. J., Riddell, I. A., Browne, C., and Nitschke, J. R. (2013). Building on architectural principles for three-dimensional metallosupramolecular construction. Chem. Soc. Rev. 42, 1728–1754. doi:10.1039/c2CS35254k
Sun, Q.-F., Iwasa, J., Ogawa, D., Ishido, Y., Sato, S., Ozeki, T., et al. (2010). Self-assembled M24L48 polyhedra and their sharp structural switch upon subtle ligand variation. Science 328 (5982), 1144–1147. doi:10.1126/science.1188605
Sun, Q.-F., Murase, T., Sato, S., and Fujita, M. (2011). A sphere-in-sphere complex by orthogonal self-assembly. Angew. Chem. Int. Ed. 50 (44), 10318–10321. doi:10.1002/anie.201104670
Sun, Y., Chen, C., Liu, J., and Stang, P. J. (2020). Recent developments in the construction and applications of platinum-based metallacycles and metallacages via coordination. Chem. Soc. Rev. 49 (12), 3889–3919. doi:10.1039/d0cs00038h
Takeda, N., Umemoto, K., Yamaguchi, K., and Fujita, M. (1999). A nanometre-sized hexahedral coordination capsule assembled from 24 components. Nature 398, 794–796. doi:10.1038/19734
Ternansky, R. J., Balogh, D. W., and Paquette, L. A. (1982). Dodecahedrane. J. Am. Chem. Soc. 104 (16), 4503–4504. doi:10.1021/ja00380a040
Thuéry, P., and Harrowfield, J. (2017). Tetrahedral and cuboidal clusters in complexes of uranyl and alkali or alkaline-earth metal ions with rac- and (1r,2r)-trans-1,2- cyclohexanedicarboxylate. Cryst. Growth Des. 17 (5), 2881–2892. doi:10.1021/acs.cgd.7b00448
Thuéry, P., Nierlich, M., Masci, B., Asfari, Z., and Vicens, J. (1999). An unprecedented trigonal coordination geometry for the uranyl ion in its complex with p-tert-butylhexahomotrioxacalix[3]arenep-tert-butylhexahomotrioxacalix[3]arene. J. Chem. Soc. Dalton Trans. 21, 3151–3152. doi:10.1039/a905939c
Thuéry, P., Villiers, C., Jaud, J., Ephritikhine, M., and Masci, B. (2004). Uranyl-based metallamacrocycles: Tri- and tetranuclear complexes with (2R,3R,4S,5S)-tetrahydrofurantetracarboxylic acid. J. Am. Chem. Soc. 126 (22), 6838–6839. doi:10.1021/ja0485964
Tsubaki, K., Otsubo, T., Tanaka, K., Fuji, K., and Kinoshita, T. (1998). Stepwise construction of some hexahomooxacalix[3]arenes and their conformations in solid state. J. Org. Chem. 63 (10), 3260–3265. doi:10.1021/jo971945a
van Duynhoven, J. P. M., Janssen, R. G., Verboom, W., Franken, S. M., Casnati, A. A., Pochini, A., et al. (1994). Control of calix[6]arene conformations by self-inclusion of 1,3,5-tri-O-alkyl substituents: Synthesis and NMR studies. J. Am. Chem. Soc. 116 (13), 5814–5822. doi:10.1021/ja00092a036
Wang, K. X., and Chen, J. S. (2011). Extended structures and physicochemical properties of uranyl–organic compounds. Acc. Chem. Res. 44 (7), 531–540. doi:10.1021/ar200042t
Wang, M., Wang, C., Hao, X.-Q., Li, X., Vaughn, T. J., Zhang, Y.-Y., et al. (2014). From trigonal bipyramidal to platonic solids: Self-assembly and self-sorting study of terpyridine-based 3D architectures. J. Am. Chem. Soc. 136 (29), 10499–10507. doi:10.1021/ja505414x
Wang, Q.-Q., Day, V. W., and Bowman-James, K. (2012). Supramolecular encapsulation of tetrahedrally hydrated guests in a tetrahedron host. Angew. Chem. Int. Ed. 51 (9), 2119–2123. doi:10.1002/anie.201106090
Wang, Z. J., Clary, K. N., Bergman, R. G., Raymond, K. N., Toste, F. D., Bergman, R. G., et al. (2013). A supramolecular approach to combining enzymatic and transition metal catalysis. Nat. Chem. 5, 100–103. doi:10.1038/nchem.1531
Yan, L.-L., Tan, C.-H., Zhang, G.-L., Zhou, L.-P., Bünzli, J.-C., and Sun, Q.-F. (2015). Stereocontrolled self-assembly and self-sorting of luminescent europium tetrahedral cages. J. Am. Chem. Soc. 137 (26), 8550–8555. doi:10.1021/jacs.5b03972
Yoshizawa, M., Klosterman, J. K., and Fujita, M. (2009). Functional molecular flasks: New properties and reactions within discrete, self-assembled hosts. Angew. Chem. Int. Ed. 48 (19), 3418–3438. doi:10.1002/anie.200805340
Young, N. J., and Hay, B. P. (2013). Structural design principles for self-assembled coordination polygons and polyhedra. Chem. Commun. 49, 1354–1379. doi:10.1039/c2CC37776d
Zhang, Z-H., Senchyk, G. A., Liu, Y., Spano-Franco, T., Szymanowski, J. E. S., and Burns, P. C. (2017). Porous uranium diphosphonate frameworks with trinuclear units templated by organic ammonium hydrolyzed from amine solvents. Inorg. Chem. 56 (21), 13249–13256. doi:10.1021/acs.inorgchem.7b02019
Keywords: uranyl–organic framework, metallo-cages, self-assembly, calixarenes, porous materials
Citation: Wu J-C, Escudero-Adán EC, Martínez-Belmonte M and de Mendoza J (2023) A tetrahedron from homooxacalix[3]arene, the fifth Platonic polyhedron from calixarenes and uranyl. Front. Chem. 11:1163178. doi: 10.3389/fchem.2023.1163178
Received: 10 February 2023; Accepted: 28 March 2023;
Published: 21 April 2023.
Edited by:
Severin Schneebeli, University of Vermont, United StatesReviewed by:
Gongrui Wang, Dalian Institute of Chemical Physics (CAS), ChinaWenbo Chen, Shanghai University of Electric Power, China
Copyright © 2023 Wu, Escudero-Adán, Martínez-Belmonte and de Mendoza. This is an open-access article distributed under the terms of the Creative Commons Attribution License (CC BY). The use, distribution or reproduction in other forums is permitted, provided the original author(s) and the copyright owner(s) are credited and that the original publication in this journal is cited, in accordance with accepted academic practice. No use, distribution or reproduction is permitted which does not comply with these terms.
*Correspondence: Javier de Mendoza, am1lbmRvemFAaWNpcS5lcw==