- 1National Institute of Materials Physics, Magurele, Romania
- 2Faculty of Physics, University of Bucharest, Magurele, Romania
- 3Ilie Murgulescu Institute of Physical Chemistry, Romanian Academy, Bucharest, Romania
Layered zinc hydroxynitrate (ZHN), with the chemical formula Zn5 (OH)8 (NO3)2·2H2O, exhibits a range of special properties such as anion-exchange and intercalation capacity, as well as biocompatibility, making it attractive for a large variety of applications in fields from nanotechnology to healthcare and agriculture. In this study nanocrystalline ZHN doped with 1,000 ppm Mn2+ was prepared by two synthesis methods (coprecipitation and solid state reaction) using similar environment-friendly precursors. The complex morpho-structural [X-ray diffraction, scanning and transmission electron microscopy, textural analysis] and spectroscopic [Fourier transform infrared and electron paramagnetic resonance (EPR)] characterization of the two ZHN nanopowders showed similar crystalline structures with Mn2+ ions localized in the nanocrystals volume, but with differences in their morphological and textural characteristics, as well as in the doping efficiency. ZHN obtained by coprecipitation consists of larger nanoplatelets with more than two times larger specific surface area and pore volume, as well as a dopant concentration than in the ZHN sample obtained by solid state reaction. The thermal stability and the on-set of the structural phase transformation have been investigated at atomic scale with high accuracy by EPR, using Mn2+ as paramagnetic probes. The on-set of the ZHN structural phase transformation toward ZnO was observed by EPR to take place at 110°C and 130°C for the samples prepared by coprecipitation and solid state reaction, respectively, evidencing a manganese induced local decrease of the transformation temperature. Our results contribute to the selection of the most appropriate ZHN synthesis method for specific applications and in the development of new green, cost-effective synthesis routes for Mn2+ doped nano-ZnO.
1 Introduction
After the discovery of polymeric nanocomposite materials containing mineral clays, a considerable increase in the scientific interest for layered compounds was recorded, especially for the layered double hydroxide salts (LDHS) (Braterman et al., 2004; Oh et al., 2009). Meanwhile, the structurally similar layered hydroxide salts (LHS) exhibit the same interesting properties such as anion-exchange and intercalation capacity, which enable their use as catalysts and matrices of functional nanocomposites with polymers. (Newman and Jones, 1999; Wypych et al., 2005; Biswick et al., 2006; Marangoni et al., 2009; Cursino et al., 2010; Machado et al., 2010; Reinoso et al., 2014; de Oliveira and Wypych, 2016; Ghotbi et al., 2018). Other applications in nanomedicine, agriculture and cosmetics are related to the biocompatibility of these layered materials, e.g., slow releasing drug delivery agents, biomolecule reservoirs, fertilizers, herbicides, sunscreens (Oh et al., 2009; Cursino et al., 2010; Li et al., 2012; Ruiz et al., 2020).
A typical representative of LHS is zinc hydroxynitrate, with molecular formula Zn5 (OH)8 (NO3)2·2H2O (ZHN). One of the problems limiting the large scale use of this compound for most of these applications is its thermal stability. The thermal stability of ZHN was investigated in the past five decades by several groups and several different mechanisms for the thermal decomposition to zinc oxide (ZnO) were proposed. However, most of the studies report the same two overlapping steps for the decomposition, below 200°C or between 200°C and 320°C, depending on the synthesis or post-synthesis conditions, steps that involve the formation of intermediates like anhydrous Zn5 (OH)8 (NO3)2 and Zn3 (OH)4 (NO3)2 (Stählin and Oswald, 1971; Biswick et al., 2007; Ahmadi et al., 2013).
It is expected that the morpho-structural properties affect material’s efficiency for specific applications. Therefore, it is important to establish performant and reproducible synthesis algorithms, resulting in materials with defined morpho-structural characteristics, in view of a larger scale use of ZHN. To our knowledge, there are few reports containing information on the morphology of the investigated ZHN (Hussein et al., 2009; Li et al., 2012; Reinoso et al., 2014; de Oliveira and Wypych, 2016; Ruiz et al., 2020), while the ZHN crystallinity degree and crystallite size are mentioned only by Hussein et al. (2009). No discussion is available in literature on the influence of the ZHN morpho-structural aspects on the material thermal stability and its structural phase transformation path.
Electron paramagnetic resonance (EPR) spectroscopy of lightly doped (nano)-crystals has been successfully used to investigate structural phase transformations at atomic scale using paramagnetic probing ions, resulting in a richer information at atomic level and higher accuracy than in the case of thermal analysis methods (Nistor et al., 2014). Mn2+ proved to be a good paramagnetic probe in zinc based compounds, due to the similar charge state and close ionic radii with the host Zn2+ ions (Nistor et al., 2013; Stefan et al., 2013; Ghica et al., 2014). In low concentrations (below 1% nominal concentration), the Mn2+ doping ions do not affect the host lattice and allow the determination with high sensitivity and accuracy of the on-set of the phase transformations (Ghica et al., 2011; Nistor et al., 2013; Ghica et al., 2019). Moreover, due to the EPR sensitivity which is much higher than that of the X-ray diffraction (XRD) technique, low concentrations of doped minority phases could be identified as well (Ghica et al., 2011; Nistor et al., 2013; Ghica et al., 2016).
In answer to all aspects and problems discussed above, we have synthesized nanostructured ZHN by two cost-effective methods, using the same environment-friendly precursors and investigated the morpho-structural properties and thermal stability of the resulting Mn-doped ZHN nanostructured powders, within an original approach. The localization of the low concentration Mn2+ ions and the doping efficiency in the two ZHN nano-systems, of particular interest for applications, were evaluated by EPR. The structural phase transformation was monitored at atomic scale by EPR with the Mn2+ probing ions, showing with high accuracy the on-set of the structural transformation toward ZnO and the differences in the two nano-systems.
The importance of this work is better evidenced in a larger context, as it opens the way to applications in a wide range of fields: i) green, cost-effective ZHN synthesis by different methods, for applications from nanotechnology to healthcare and agriculture; ii) developing new cost-effective synthesis routes for Mn2+ doped nano-ZnO. ZnO is one of the most intensively studied semiconductor with already a wide range of applications from nano- and/or optoelectronics to multiple bio-medical applications (Fortunato et al., 2009; Hoye et al., 2013; Verma et al., 2021). Furthermore, by doping it is possible to tailor or develop new material properties, extending the range of applications (Sharma et al., 2003; Dietl, 2010; Zhang et al., 2018; Pradhan, 2019; Popescu et al., 2020; Pradeep and Viswanatha, 2020).
2 Materials and methods
2.1 Materials synthesis
Zn5 (OH)8 (NO3)2·2H2O has been synthesized by two methods, using the same precursors without any further purification: zinc nitrate hexahydrate [Zn (NO3)2·6H2O, from Sigma Aldrich], manganese nitrate tetrahydrate [Mn (NO3)2·4H2O, from Alfa Aesar] and sodium hydroxide [NaOH, from Sigma Aldrich]. The preparation procedures for the two materials, labeled ZHNCOPP and ZHNSSR after the coprecipitation and solid state reaction methods used, are detailed below:
ZHNCOPP: Coprecipitation method—an aqueous solution containing zinc nitrate and manganese nitrate, in the corresponding amounts for 1,000 ppm Mn2+ nominal concentration, was slightly acidified with 250 mL HNO3 (1 M) and magnetically stirred for several minutes. Afterwards, this mixture was precipitated with a strong basic solution of NaOH 2.4 M (excess was used) added slowly with a peristaltic pump, at nearly 35°C, under continuous magnetic stirring. The suspension thus obtained was allowed to age for 1 hour at the same temperature, under continuous magnetic stirring. The precipitate was collected by centrifugation and washed several times with bi-distilled water and absolute ethanol, and air dried at 60°C.
ZHNSSR: Solid state reaction method—zinc nitrate hexahydrate, manganese nitrate tetrahydrate (the amount corresponding to a 1,000 ppm Mn2+ nominal concentration) and sodium hydroxide were thoroughly mixed and ground in an agate mortar, in a molar ratio 1:0.62, without any water or other solvents added, until a homogenous white paste was formed. The paste was collected in a beaker and was carefully washed with bi-distilled water several times. The white precipitate separated by centrifugation was air dried at 60°C.
2.2 Materials characterization
XRD patterns were recorded with a Bruker D8 Advance X-ray diffractometer with Cu anode and Ni filter (λ = 0.154184 nm), in Bragg-Brentano geometry. The structural parameters of the identified crystalline phases were determined by Rietveld refinement of the experimental XRD data with the Bruker Topas v. 3 software.
Morpho-structural investigations down to the nano-scale were performed by scanning electron microscopy (SEM) and transmission electron microscopy (TEM) using Tescan Lyra III FEG and JEM 2100 electron microscopes, respectively.
Nitrogen adsorption-desorption isotherms at −196°C were recorded on a Micromeritics ASAP 2020 apparatus. Both samples were degassed at 70°C for 15 h, under vacuum, prior to N2 adsorption. The analysis of the isotherms allowed us to determine the specific surface area (SBET), according to the Brunauer–Emmett–Teller (BET) equation, the total pore volume (Vtotal) from the amount adsorbed at the relative pressure of 0.995, and the mean pore diameter using Barrett–Joyner–Halenda (BJH) method.
Fourier transform infrared (FTIR) spectra were recorded with a Spectrum BX II (Perkin Elmer) spectrometer in absorbance mode, in the 4,000 cm−1–400 cm−1 spectral range, with 32 scans and a resolution of 4 cm−1. The samples were embedded in KBr pellets with a mass-ratio sample: KBr of 1:100.
EPR investigations were performed on weighted amounts of powders inserted in calibrated pure fused silica sample tubes of 2 mm inner diameter. X (9.8 GHz) and Q (34 GHz) -band EPR measurements were performed at room temperature (RT) with the Bruker ELEXSYS E580 and E500 spectrometers, respectively. The magnetic field calibration at the sample was made with a BDPA (alpha, gamma–bisdiphenylene-beta-phenyl allyl) reference sample from Bruker, exhibiting a single EPR line with g = 2.00276. The spin Hamiltonian (SH) parameters of the observed paramagnetic centers were determined with the EASYSPIN v.5.2.28 software (Stoll and Schweiger, 2006). Isochronal annealing experiments from 100°C up to 150°C were performed in air in steps of 10°C for 10 min at each temperature in order to study the thermal evolution of the samples inside the EPR tubes. The samples were sequentially annealed at successive temperatures in a temperature stabilized (±1°C) furnace and cooled down to RT for further measurements. Small amounts of samples extracted from the EPR tube after specific annealing treatments were deposited on a zero-diffraction Si wafer (MTI Corporation, USA) for XRD measurements.
3 Results and discussion
3.1 XRD investigation
Both ZHNCOPP and ZHNSSR samples (Figure 1A) exhibit X-ray diffractograms indexed as pure zinc hydroxynitrate hydrate–Zn5 (OH)8 (NO3)2·2H2O, monoclinic structure, space group C2/m, ICDD 04-011-5271. The Rietveld refinement of the experimental data resulted in similar structural parameters: a = 1.9480 nm, b = 0.6238 nm, c = 0.5517 nm, α = 90o, β = 93.28o, γ = 90o, and average crystallite size d = 25 nm ± 3 nm. A pronounced (200) texture is observed in the case of the ZHNCOPP sample, with a corresponding coherence distance on the [200] direction of 40 nm ± 3 nm. As the XRD texture could be associated with the shape anisotropy of the nanocrystals, this aspect has been investigated by electron microscopy in Section 3.2.
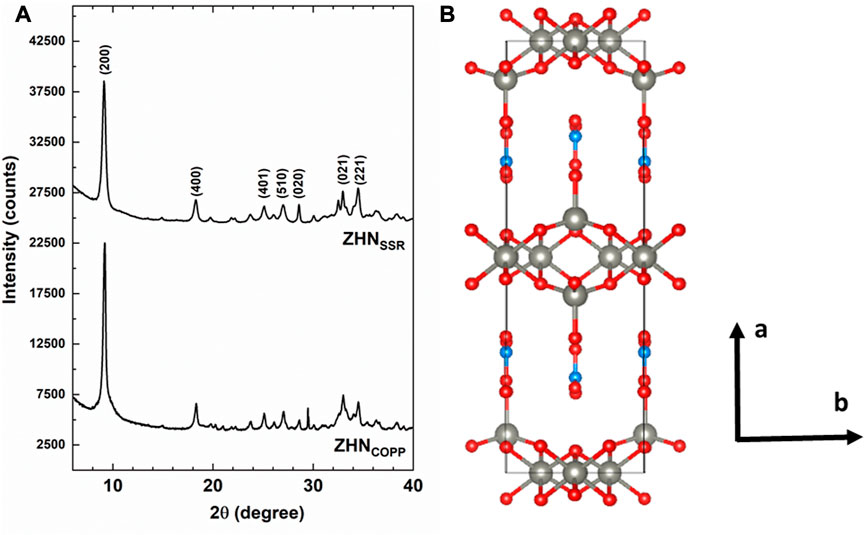
FIGURE 1. (A) XRD patterns of the as-prepared ZHN samples. Only the most intense peaks of the Zn5 (OH)8 (NO3)2 (H2O)2 structure have been marked. (B) Structural model along the c-axis with the following color codes for atoms: grey–Zn, red–oxygen, light blue–nitrogen.
Figure 1B presents a view along the c-axis of the structural model of the ZHN compound with the structural parameters from above. According to previous structural investigations (Stählin and Oswald, 1970; Stählin and Oswald, 1971; Chouillet et al., 2004; Biswick et al., 2007), the layered structure of Zn5 (OH)8 (NO3)2·2H2O consists of Zn (OH)2 layers with CdI2 type structure. In each such layer one-quarter of the zinc atoms are missing from the octahedral sites, while each occupied octahedron shares its edges with four occupied and two unoccupied octahedral sites. Tetrahedrally coordinated Zn2+ cations are located above and below the empty octahedra, coordinated in three corners of the tetrahedron by hydroxide ions from the brucite-like layers and in the fourth corner by a water molecule. The nitrate anions are not directly coordinated to the zinc ions, being located between the sheets and lying in a plane normal to them, preserving the D3h symmetry. The hydrogen bonds holding together the sheets bind the oxygen atoms of the nitrate groups with the water molecule and with two hydroxide ions of the sheet.
It should be mentioned that the presence of the Mn2+ ions with 0.1% nominal concentration in the host ZHN lattice would not be observable by XRD investigations. The Mn2+ ions have similar charge state and close ionic radii with the host Zn2+ ions (Shannon, 1976). The substitution of the tetrahedrally coordinated Zn2+ ions (crystal radius R = 0.074 nm) by the slightly larger Mn2+ ions (R = 0.080 nm) would produce a small expansion of the host lattice associated with lattice disorder. This would be evidenced by an increase of the lattice parameters and a decrease of the crystallite size, observed in XRD patterns as shifts in the peak positions and line broadening, respectively. However, at low dopant concentration this effect is reduced, and below 1% nominal concentration the Mn2+ doping ions would not affect the host lattice in a detectable way (Ghica et al., 2011; Nistor et al., 2013; Ghica et al., 2019), as confirmed by our XRD results. Instead, the localization of the Mn2+ ions and the annealing induced changes in their neighborhood/environment were investigated by the more sensitive EPR spectroscopy as presented in Section 3.5.
3.2 Electron microscopy investigations
SEM. SEM images of the ZHNCOPP and ZHNSSR samples are shown in Figures 2A, B. Both samples consist of agglomerated platelets shaped as irregular polygons, randomly oriented, but with much larger dimensions (up to about 10 μm) for the ZHNCOPP sample. Because of this dimensional difference, the two samples observation had to be performed with different microscope magnitudes, as seen in Figure 2. Both samples display the characteristic plate-like morphology of the layered materials, with nanometric-scale thick platelets, thinner than previously reported (Li et al., 2012; de Oliveira and Wypych, 2016; Ruiz et al., 2020). A comparison of Figures 2A, B evidences a more pronounced shape anisotropy in the case of the ZHNCOPP nanocrystals, which can indeed explain the observed texture in the XRD pattern of this sample.
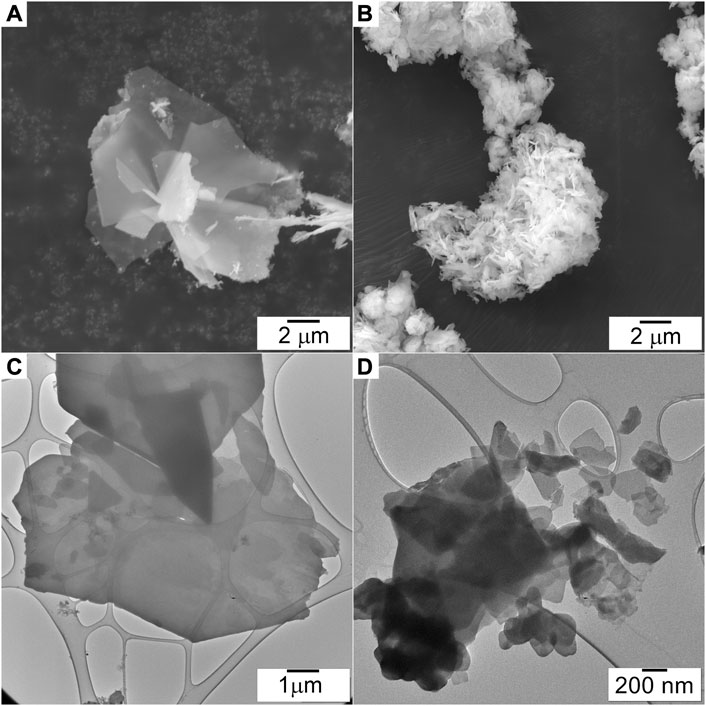
FIGURE 2. SEM images of the ZHNCOPP (A) and ZHNSSR (B) samples. TEM images of the ZHNCOPP (C) and ZHNSSR (D) samples.
TEM. Low magnification TEM images of the ZHNCOPP and ZHNSSR samples are shown in Figures 2C, D. The ZHNCOPP sample consists of platelets of large dimensions (few μm to above 10 μm) and much smaller thickness, proven by the fact that the carbon membrane on which particles are supported is still visible. The ZHNSSR sample also consists of platelets but of smaller size, in the range of a few hundred nanometers. Both samples were very sensitive to the electron beam, a fact that hindered the analysis at higher magnification, although damage prevention measures were taken (the accelerating voltage was set to 80 kV and the images were taken using low beam intensity and longer exposure times).
3.3 Textural analysis
The N2 adsorption–desorption isotherms of the samples are shown in Figure 3. Both isotherms can be classified as type IV according to IUPAC classification, with small hysteresis loops of H3 type (Sing et al., 1985). Both specific surface area and total pore volume are more than two times larger for the ZHNCOPP sample than for the ZHNSSR sample, as seen in Table 1. These values are in agreement with those reported in the literature for materials obtained by similar methods (Reinoso et al., 2014). However, the mean diameter of the pores is smaller for ZHNCOPP than for ZHNSSR. The notable differences in the specific surface area and total pore volume are correlated with the different size/morphology observed by electron microscopy for the two ZHN nanostructures. The appearance of the hysteresis loop at relative pressure values higher than 0.9 indicates the existence of relatively large pores, most likely coming from the interparticle voids.
To our knowledge, the results presented in Sections 3.1–3.3 represent the first comparative study of the morpho-structural properties of the ZHN compounds obtained by two different methods. By using two different synthesis methods, but keeping as many synthesis parameters similar as possible, i.e., the same precursors, Mn doping level, precipitate washing process and drying temperature and time, we have obtained ZHN nanostructures with notably different morphological properties. Up to now there are only a few reports on the morphology of the ZHN compounds (Hussein et al., 2009; Li et al., 2012; Reinoso et al., 2014; de Oliveira and Wypych, 2016; Ruiz et al., 2020), and no correlation was made with the synthesis method. It is generally known that the size and morphology at nanoscale are influenced by several factors, including the synthesis method itself, changes in the synthesis or post-synthesis parameters, doping, etc., and changes in just one parameter could result in a spectacular shape change (Yang et al., 2013; Ghica et al., 2016; Guria and Pradhan, 2016). In our case, it is possible that the mechanical stress due to the grinding and also the lack of any solvent (other than the water adsorbed from the atmosphere) affected the growth of the ZHNSSR platelets, impeding the development of highly anisotropic shapes, while for ZHNCOPP the preparation conditions were more suitable for the growth of well crystallized platelets, in the solvent presence. Despite intensive research in this field, growth mechanisms are still a difficult issue even for simple oxide, sulfide, selenide structures (ZnO, ZnS, ZnSe, CdSe, etc.) and further experimental and theoretical research is required (Yang et al., 2013; Guria and Pradhan, 2016).
3.4 FTIR results
As it can be observed in Figure 4, the two ZHN samples exhibit the same absorption bands previously reported for the Zn5 (OH)8 (NO3)2·2H2O compound (Stählin and Oswald, 1971; Chouillet et al., 2004). The sharp peak around 3,570 cm−1 and the intense peak at 3,400 cm−1 are assigned to various stretching vibrations of the O-H bonds in Zn5 (OH)8 (NO3)2·2H2O (Chouillet et al., 2004). The broad band around 3,300 cm−1 and the peak around 1,640 cm−1 indicate the presence of water molecules in the interlayer space or adsorbed on the surface. For both spectra shown in Figure 4, the most intense peak at about 1,380 cm−1 corresponds to the ν3 vibration (NO2 stretching mode of a free nitrate ion), while the weak peaks around 1,050 cm−1 correspond to the ν1 vibration (NO stretch of a free nitrate group), 840 cm−1 to the ν2 vibration (out of plane vibration) and 720 cm−1 to the ν4 vibration (NO2 bend), characteristic to various vibration modes of the nitrate group (Stählin and Oswald, 1971; Chouillet et al., 2004). It should be emphasized that, as observed by Chouillet et al. (2004), the 1,380 cm−1 absorption band is not split, in agreement with Zn5 (OH)8 (NO3)2·2H2O structure in which the nitrate ion is not coordinated (Louër et al., 1973). Moreover, the weak absorption band at 1,050 cm−1 is infrared forbidden and should not appear in a D3h symmetry, but it can be present when the symmetry is slightly distorted (Gatehouse et al., 1957). Below 1,000 cm−1 translation and bending vibration modes for Zn-OH, nitrate group and Zn-O bonds, with low intensity, can be observed in the spectra of both compounds (Jnido et al., 2021).
3.5 EPR investigation
The EPR spectra of the as-prepared samples in the X (9.86 GHz) and Q (34.12 GHz) frequency bands, normalized for differences in mass and spectrometer parameters, are presented in Figures 5A, B, respectively. For each sample the EPR spectrum consists of several overlapping individual spectra with a characteristic six-lines hyperfine structure (better observed in Q-band, Figure 5B), attributed to Mn2+ ions (S = 5/2, I = 5/2) localized in different host lattices. The EPR spectra of the two samples are quite similar, with close line widths values and the spectrum of the ZHNCOPP sample about three times more intense than the spectrum of the ZHNSSR sample. It can thus be inferred that the Mn2+ ions concentration is three times higher in the ZHNCOPP sample than in the ZHNSSR sample, pointing to an enhanced doping efficiency when the coprecipitation synthesis route is used. We associate this result to the enhanced shape anisotropy of the ZHNCOPP nanocrystals, in agreement with theoretical studies which show that the doping efficiency varies with the morphology and size of the nanocrystals (Erwin et al., 2005; Singh et al., 2010).
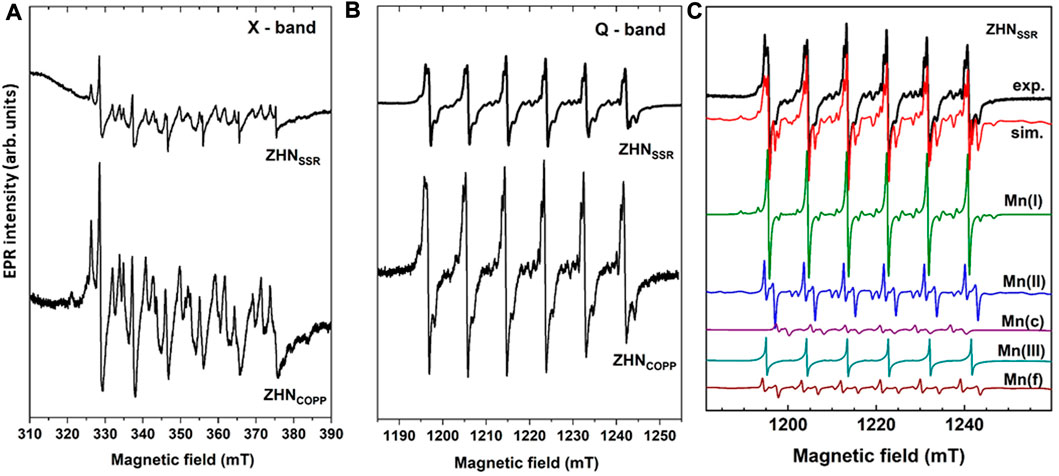
FIGURE 5. EPR spectra of the as-prepared samples measured at RT in X-band (9.86 GHz) (A), and Q-band (34.12 GHz) (B). (C) Experimental (exp.) and simulated (sim.) EPR spectrum in Q-band of the ZHNSSR sample. The component spectra of the indicated centers, calculated with the SH parameters from Table 2, are presented below.
The EPR spectra of the Mn2+ paramagnetic centers were analyzed using the following spin Hamiltonian (SH), where the first term is the electron Zeeman interaction of the S = 5/2 electron spin with the external magnetic field B and the second term is the hyperfine interaction of the electron spin with the I = 5/2 nuclear spin of the 55Mn (100% abundance) isotope, responsible for the characteristic six-lines hyperfine structure. The next two terms are zero-field-splitting (ZFS) terms describing the interaction of the electron spin with the local crystal field, while the last term is the nuclear Zeeman interaction (Abragam and Bleaney, 1970; Stefan et al., 2014):
For nanostructured materials, the contribution of random strains and lattice disorder is very important, producing a variation in the local crystal field at the paramagnetic ion sites, both in magnitude and orientation, reflected in the broadening of the individual EPR line width. This line broadening is reproduced in the lineshape simulation of the experimental spectra by including a statistical distribution of the axial ZFS-parameter value D, described by the corresponding standard deviation σ(D) (Stefan et al., 2013). The EPR parameters (g, A, D, a) of the Mn2+ centers, σ(D) and individual linewidth (ΔB), as determined by simulation and fitting of the Q-band spectrum of the ZHNSSR sample are presented in Table 2. The Mn(I) and Mn(II) centers correspond to Mn2+ ions in ZHN, while the Mn(III) centers are Mn2+ ions localized in a Zn3 (OH)4 (NO3)2 phase (Ghica et al., 2019). Mn(c) corresponds to Mn2+ ions in crystalline ZnO (Stefan et al., 2013), while Mn(f) is associated to traces of doped precursor. The simulated Q-band spectrum of the ZHNSSR sample with all the above mentioned contributions (Table 2) is presented in Figure 5C. The relative concentrations of the Mn2+ centers in the respective hosts, given in Table 2 with an accuracy of ±2%, are proportional to the integrated intensities of the associated spectra. Thus, in the ZHNSSR sample, the concentration of the Mn2+ ions in traces of ZnO and precursors is much lower than the concentration of the Mn2+ ions in ZHN. On the other hand, the EPR spectrum of the ZHNCOPP sample shows that the Mn2+ ions are localized substitutionally only in the two Zn2+ sites from ZHN.
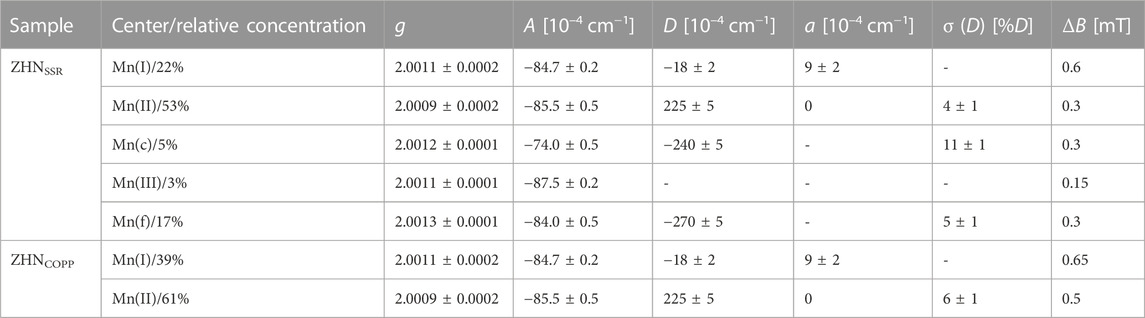
TABLE 2. Relative concentration, EPR parameters (g, A, D and a), broadening parameter σ (D) and individual peak-to-peak linewidth (ΔB) of the Mn2+ centers in the ZHN samples.
The observation of the Mn2+ ions in a crystalline phase of ZnO in the ZHNSSR EPR spectrum, with a relative concentration of 5%, lead us to check for the possible presence of ZnO peaks in the XRD patterns of both samples. This minority phase would not be distinguishable in diffractograms, as the corresponding XRD peaks with very low intensities are hidden under the main peaks of the ZHN phase. The Rietveld refinement performed by including ZnO alongside Zn5 (OH)8 (NO3)2·2H2O resulted in a better fit of the diffractograms and allowed the identification of traces (1%–2%) of ZnO hexagonal phase (space group P63mc, ICDD 01-070-8070) with average crystallite size of ∼15 nm in both samples.
The spectral parameters of the two Mn-related centers [Mn(I) and Mn(II)] in ZHN are similar in both samples and correspond to the ones reported by Ghica et al. (2019), indicating the presence of the Mn doping ions in the sample volume for both synthesis methods. Along with the structural investigations, this result shows similar structural quality at atomic scale of both ZHN nano-systems obtained by the two synthesis methods, even though ZHNSSR contains traces of precursor phases.
The thermal decomposition of both ZHN samples has been investigated by EPR using the Mn doping ions as paramagnetic probes. Their EPR spectra after isochronal annealing experiments up to 150°C are represented in Figures 6A, B, where only the first three hyperfine transitions at low-field are displayed for simplicity. At temperatures above 100°C the Mn(I) and Mn(II) EPR spectra become weaker, and new centers are formed. The thermal evolution of the Mn2+ centers reflects the evolution of their host material, namely, the decomposition of ZHN and the formation of new phases. A notable increase in the intensity of the EPR spectrum of Mn(c) (Mn2+ ions in ZnO nanocrystals) appears at 110°C for the ZHNCOPP sample, and at 130°C for the ZHNSSR sample. We should mention that a similar EPR investigation of the thermal decomposition of crystalline Zn(OH)2 showed the on-set of the formation of nanocrystallline ZnO at 120°C (Nistor et al., 2013b). We note that in the ZHNCOPP sample, the Mn(III) centers are formed only above 100°C. For both samples the Mn(III) centers decrease in intensity with the annealing temperature increase, being hardly visible at 150°C (Figures 6A, B). For an easier observation of the evolution of the Mn2+ spectra from Figure 6A, B we have marked the first hyperfine transition at low-field of the Mn2+ centers with a green rectangle for all zinc hydroxynitrate compounds [Mn(I) and Mn(II) in Zn5 (OH)8 (NO3)2·2H2O and Mn(III) in Zn3 (OH)4 (NO3)2], and with a dark red rectangle for ZnO.
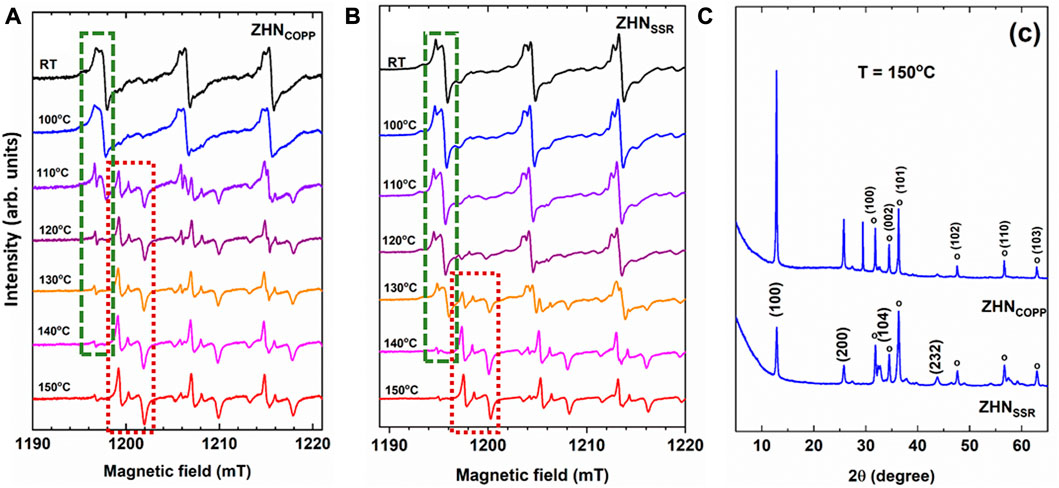
FIGURE 6. Detailed view of the EPR spectra of the Mn2+ ions in the ZHNCOPP (A), and ZHNSSR (B) samples, as-prepared (RT) and isochronally annealed (10 min at each indicated temperature). Only the first three hyperfine transitions at low-field are displayed for simplicity. Transformation of the Mn2+ centers in the zinc hydroxynitrate phases into Mn2+ centers in ZnO is evidenced with green and dark red dotted lines, respectively, only for the first hyperfine transition, where these centers do not overlap. (C) XRD patterns of both samples after isochronal annealing up to 150°C. The ZnO phase is marked with open circles and indexed in the upper pattern, while the Zn3 (OH)4 (NO3)2 phase is indexed in the lower pattern.
After isochronal annealing up to 150°C, the EPR spectra of both samples show mostly the Mn(c) paramagnetic center, meaning that almost all Mn2+ ions are localized in the volume of the ZnO phase. However, the corresponding XRD patterns of both samples consist of a mixture of two different crystalline phases: Zn3 (OH)4 (NO3)2 and ZnO (Figure 6C), with an enhanced transformation towards ZnO in the case of the ZHNSSR sample. It can thus be concluded that the transformation of ZHN into ZnO is initiated at lower temperatures in the sample regions containing manganese than in the pure ones, independent of the synthesis routes, as further explained. The doping process can be used to change or modulate the morpho-structural properties of nanostructured materials, as the doping ions could influence the nanocrystals growth, affecting both the size and morphology, and even induce structural phase transformations or decomposition toward different compounds (Erwin et al., 2005; Ghica et al., 2016; Guria and Pradhan, 2016; Pradhan, 2019). In a previous report, Ghica et al. (2016) have shown that in the case of the Zn (OH)2 compound, the minute addition of Mn2+ ions (1 ppm) re-directed the coprecipitation synthesis toward Mn-doped ZnO instead of Mn-doped Zn (OH)2. It was suggested that this effect could be driven by a coordinative hindrance suffered by Mn2+, which in most compounds assumes an octahedral coordination, when substituting for Zn2+ in a site tetrahedrally coordinated by four (OH)− ligands. As mentioned in Section 3.1, there are two cationic sites in the ZHN compound: One with Zn2+ ions octahedrally coordinated by six (OH)− groups and one with Zn2+ ions tetrahedrally coordinated by three (OH)− groups and one water molecule. It is possible that the Mn2+ ions substituting for Zn2+ ions in tetrahedral sites could favor the transformation into ZnO by a similar mechanism. The tetrahedrally coordinated sites could thus become nucleation sites for ZnO at a lower temperature than in the undoped material. In our previous study of ZnO nanostructures obtained by coprecipitation followed by annealing (Ghica et al., 2019), we have observed that the transformation of ZHN into ZnO was initiated at lower temperatures in the sample regions containing manganese than in the pure ones. As shown in the present work, the ZHN to ZnO transformation at a lower temperature at the Mn2+ sites takes place for both ZHN compounds with different morphologies obtained by two different synthesis methods. This effect is thus the result of the doping process itself, and not dependent on the synthesis method.
On the other hand, the difference in the onset temperature for the phase transformation in the two nanostructured ZHN samples (110°C for ZHNCOPP and 130°C for ZHNSSR) is related to their different size and morphology resulted from the specific synthesis method used. This correlation has been detailed in the last paragraph of Section 3.3, following the morpho-structural analysis. Although both ZHN samples have the same nominal doping level, the EPR study has shown that the concentration of the substitutional Mn2+ ions is higher in the ZHNCOPP sample, therefore providing more nucleation sites for ZnO in the ZHNCOPP sample than in the ZHNSSR sample. This result can also explain the lower onset temperature for the transformation into ZnO in the ZHNCOPP sample than in the ZHNSSR sample.
Our result points to a more convenient lower temperature synthesis of ZnO nanocrystals by thermal decomposition of ZHN obtained either by coprecipitation or solid state reaction, when ZHN is doped with Mn2+ ions. This subject deserves further investigation, towards the identification of new cost-effective synthesis routes of Mn2+ doped nano-ZnO. Doping in general and Mn2+ doping specifically in the case of nanostructured ZnO can modulate/enhance material properties for different applications (Sharma et al., 2003; Kittilstved and Gamelin, 2006; Dietl, 2010; Hoye et al., 2013; Jiang et al., 2018; Martínez-Carmona et al., 2018; Zhang et al., 2018; Pradhan, 2019; Popescu et al., 2020; Pradeep and Viswanatha, 2020) as a function of the dopant concentration and localization. However, the synthesis of nanostructured doped ZnO is not trivial, as shown by the still not-resolved issues regarding the control and uniformity of the dopant distribution and solubility, agglomeration and formation of minority phases, all depending on the synthesis method and procedures. Thermal decomposition at low temperatures of Mn2+ doped ZHN promises to be a good method to obtain uniformly doped ZnO nanocrystals.
To summarize, a comparison of the two synthesis routes for ZHN seems to incline the balance in favor of the coprecipitation route. The two samples have similar structure and crystallite size, the dopant Mn2+ ions are easily incorporated in the host lattices, but the coprecipitation sample has a higher doping efficiency. However, the solid state reaction method has the non-negligible advantage of a cleaner and simpler process, with less reactants involved, and a higher thermal stability of the as-prepared ZHN sample.
4 Conclusion
Nanocrystalline Zn5 (OH)8 (NO3)2·2H2O (ZHN), doped with a small amount of Mn2+ ions, was prepared by two different synthesis routes, coprecipitation and solid state reaction using the same environment-friendly reactants. The resulting nanocrystalline samples have distinct morpho-structural and textural properties, clearly influenced by the synthesis method. While in both cases the structure and crystallite size are similar, the sample obtained by coprecipitation exhibits much larger platelets with specific surface area and total pore volume more than two times larger than in the case of the sample obtained by solid state reaction. From the analysis of the EPR spectra we determined that the Mn2+ dopant ions are localized substitutionally in the Zn2+ sites of the host lattice in both samples. The concentration of the Mn2+ ions is three times higher in the coprecipitation sample, showing that this synthesis route ensures an enhanced doping efficiency. The on-set of the ZHN structural phase transformation toward ZnO was observed by EPR at 110°C and 130°C for the samples synthesized by coprecipitation and solid state reaction, respectively. A comparison of the EPR results with the structural and compositional XRD results showed that the Mn2+ dopant ions promote the local transformation of ZHN into ZnO at lower temperatures. This is the first detailed investigation of the decrease in the ZHN to ZnO transformation temperature initiated by the Mn2+ doping.
Our results provide a basis for the selection of the appropriate synthesis method for nanocrystalline ZHN suitable for the envisaged application in nanotechnology, healthcare or agriculture and for the development of new green, cost-effective synthesis routes of Mn2+ doped nano-ZnO.
Data availability statement
The original contributions presented in the study are included in the article/supplementary material, further inquiries can be directed to the corresponding author.
Author contributions
DG and IV conceptualized the project and were in charge of overall planning. All authors performed experiments, analyzed and interpreted data and contributed to discussions. DG, IV, and MS drafted the manuscript with support from all authors. All authors have read and agreed to the published version of the manuscript.
Funding
This work is funded by the Core Program of the National Institute of Materials Physics, granted by the Romanian Ministry of Research, Innovation and Digitalization through the Project PC1- PN23080101. The fee for open access publication was supported from the project 35PFE/2021, funded by the Romanian Ministry of Research, Innovation and Digitization.
Conflict of interest
The authors declare that the research was conducted in the absence of any commercial or financial relationships that could be construed as a potential conflict of interest.
Publisher’s note
All claims expressed in this article are solely those of the authors and do not necessarily represent those of their affiliated organizations, or those of the publisher, the editors and the reviewers. Any product that may be evaluated in this article, or claim that may be made by its manufacturer, is not guaranteed or endorsed by the publisher.
References
Abragam, A., and Bleaney, B. (1970). Electron paramagnetic resonance of transition ions. Oxford: Clarendon Press.
Ahmadi, S. J., Hosseinpour, M., Javadi, F., and Tayebee, R. (2013). Optimization study on formation and decomposition of zinc hydroxynitrates to pure zinc oxide nanoparticles in supercritical water. Ind. Eng. Chem. Res. 52, 1448–1454. doi:10.1021/ie3026218
Biswick, T., Jones, W., Pacuła, A., Serwicka, E., and Podobinski, J. (2007). The role of anhydrous zinc nitrate in the thermal decomposition of the zinc hydroxy nitrates Zn5(OH)8(NO3)2·2H2O and ZnOHNO3·H2O. J. Sol. State Chem. 180, 1171–1179. doi:10.1016/j.jssc.2007.01.012
Biswick, T., Jones, W., Pacuła, A., and Serwicka, E. (2006). Synthesis, characterisation and anion exchange properties of copper, magnesium, zinc and nickel hydroxy nitrates. J. Sol. State Chem. 179, 49–55. doi:10.1016/j.jssc.2005.09.040
Braterman, P. S., Xu, Z. P., and Yarberry, F. (2004). “Layered double hydroxide (LDHs),” in Handbook of layered materials. Editors S. M. Auerbach, K. A. Carrado, and P. K. Dutta (New York: Marcel Dekker), 373–474. doi:10.1201/9780203021354.ch8
Chouillet, C., Krafft, J. M., Louis, C., and Lauron-Pernot, H. (2004). Characterization of zinc hydroxynitrates by diffuse reflectance infrared spectroscopy – structural modifications during thermal treatment. Spectrochim. Acta, Part A 60, 505–511. doi:10.1016/S1386-1425(03)00255-5
Cursino, A. C. T., da Costa Gardolinski, J. E. F., and Wypych, F. (2010). Intercalation of anionic organic ultraviolet ray absorbers into layered zinc hydroxide nitrate. J. Colloid Interface Sci. 347, 49–55. doi:10.1016/j.jcis.2010.03.007
de Oliveira, H. B., and Wypych, F. (2016). Evaluation of layered zinc hydroxide nitrate and zinc/nickel double hydroxide salts in the removal of chromate ions from solutions. J. Solid State Chem. 243, 136–145. doi:10.1016/j.jssc.2016.08.019
Dietl, T. (2010). A ten-year perspective on dilute magnetic semiconductors and oxides. Nat. Mater 9, 965–974. doi:10.1038/nmat2898
Erwin, S. C., Zu, L., Haftel, M. I., Efros, A. L., Kennedy, T. A., and Norris, D. J. (2005). Doping semiconductor nanocrystals. Nature 436, 91–94. doi:10.1038/nature03832
Fortunato, E., Gonçalves, A., Pimentel, A., Barquinha, P., Gonçalves, G., Pereira, L., et al. (2009). Zinc oxide, a multifunctional material: From material to device applications. Appl. Phys. A Mat. Sci. Process. 96, 197–205. doi:10.1007/s00339-009-5086-5
Gatehouse, B. M., Livingstone, S. E., and Nyholm, R. S. (1957). 847. Infrared spectra of some nitrato-co-ordination complexes. J. Chem. Soc., 4222–4225. doi:10.1039/JR9570004222
Ghica, D., Nistor, S. V., Nistor, L. C., Stefan, M., and Mateescu, C. D. (2011). Structural phase transformations in annealed cubic ZnS nanocrystals. J. Nanopart. Res. 13 (9), 4325–4335. doi:10.1007/s11051-011-0379-y
Ghica, D., Stefan, M., Ghica, C., and Stan, G. E. (2014). Evaluation of the segregation of paramagnetic impurities at grain boundaries in nanostructured ZnO films. ACS Appl. Mat. Interfaces 6, 14231–14238. doi:10.1021/am5035329
Ghica, D., Vlaicu, I. D., Stefan, M., Maraloiu, V. A., Joita, A. C., and Ghica, C. (2019). Tailoring the dopant distribution in ZnO:Mn nanocrystals. Sci. Rep. 9, 6894. doi:10.1038/s41598-019-43388-z
Ghica, D., Vlaicu, I. D., Stefan, M., Nistor, L. C., and Nistor, S. V. (2016). On the agent role of Mn2+ in redirecting the synthesis of Zn(OH)2 towards nano-ZnO with variable morphology. RSC Adv. 6 (108), 106732–106741. doi:10.1039/c6ra23065b
Ghotbi, M. Y., Javanmard, A., and Soleimani, H. (2018). Three dimensional metal/N-doped nanoplate carbon catalysts for oxygen reduction, the reason for using a layered nanoreactor. Sci. Rep. 8, 3404. doi:10.1038/s41598-018-21782-3
Guria, A. K., and Pradhan, N. (2016). Doped or not doped: Ionic impurities for influencing the phase and growth of semiconductor nanocrystals. Chem. Mat. 28, 5224–5237. doi:10.1021/acs.chemmater.6b02009
Hoye, R. L. Z., Musselman, K. P., and MacManus-Driscoll, J. L. (2013). Research update: Doping ZnO and TiO2 for solar cells. Apl. Mater 1, 060701. doi:10.1063/1.4833475
Hussein, M. Z. b., Ghotbi, M. Y., Yahaya, A. H., and Abd Rahman, M. Z. (2009). The effect of polymers onto the size of zinc layered hydroxide salt and its calcined product. Solid State Sci. 11, 368–375. doi:10.1016/j.solidstatesciences.2008.06.006
Jiang, J., Pi, J., and Cai, J. (2018). The advancing of zinc oxide nanoparticles for biomedical applications. Bioinorg. Chem. Appl. 2018, 1–18. doi:10.1155/2018/1062562
Jnido, G., Ohms, G., and Viöl, W. (2021). Deposition of zinc oxide coatings on wood surfaces using the solution precursor plasma spraying process. Coatings 11 (2), 183. doi:10.3390/coatings11020183
Kittilstved, K. R., and Gamelin, D. R. (2006). Manipulating polar ferromagnetism in transition-metal-doped ZnO: Why manganese is different from cobalt (invited). J. Appl. Phys. 99, 08M112. doi:10.1063/1.2167638
Li, P., Xu, Z. P., Hampton, M. A., Vu, D. T., Huang, L., Rudolph, V., et al. (2012). Control preparation of zinc hydroxide nitrate nanocrystals and examination of the chemical and structural stability. J. Phys. Chem. C 116, 10325–10332. doi:10.1021/jp300045u
Louër, M., Louër, D., and Grandjean, D. (1973). Etude structurale des hydroxynitrates de nickel et de zinc. I. Classification structurale. Acta Cryst. B 29, 1696–1703. doi:10.1107/S0567740873005285
Machado, G. S., Arízaga, G. G. C., Wypych, F., and Nakagaki, S. (2010). Immobilization of anionic metalloporphyrins on zinc hydroxide nitrate and study of an unusual catalytic activity. J. Catal. 274, 130–141. doi:10.1016/j.jcat.2010.06.012
Marangoni, R., Ramos, L. P., and Wypych, P (2009). New multifunctional materials obtained by the intercalation of anionic dyes into layered zinc hydroxide nitrate followed by dispersion into poly(vinyl alcohol) (PVA). J. Colloid Interface Sci. 330, 303–309. doi:10.1016/j.jcis.2008.10.081
Martínez-Carmona, M., Gun’ko, Y., and Vallet-Regí, M. (2018). ZnO nanostructures for drug delivery and theranostic applications. Nanomaterials 8 (4), 268. doi:10.3390/nano8040268
Newman, S. P., and Jones, W. (1999). Comparative study of some layered hydroxide salts containing exchangeable interlayer anions. J. Solid State Chem. 148, 26–40. doi:10.1006/jssc.1999.8330
Nistor, S. V., Ghica, D., Stefan, M., and Nistor, L. C. (2013). Sequential thermal decomposition of the shell of cubic ZnS/Zn(OH)2 core-shell quantum dots observed with Mn2+ probing ions. J. Phys. Chem. C 117 (42), 22017–22028. doi:10.1021/jp4063093
Nistor, S. V., Ghica, D., Stefan, M., Vlaicu, I. D., Barascu, J. N., and Bartha, C. (2013b). Magnetic defects in crystalline Zn(OH)2 and nanocrystalline ZnO resulting from its thermal decomposition. J. Alloys Compd. 548, 222–227. doi:10.1016/j.jallcom.2012.09.016
Nistor, S. V., Stefan, M., and Ghica, D. (2014). Pulse annealing electron paramagnetic resonance with probing transition ions: Application to thermal formation and growth of nanoZnO. J. Therm. Anal. Calorim. 118, 1021–1031. doi:10.1007/s10973-014-3743-1
Oh, J. M., Biswick, T. T., and Choy, J. H. (2009). Layered nanomaterials for green materials. J. Mat. Chem. 19, 2553–2563. doi:10.1039/b819094a
Popescu, T., Matei, C. O., Vlaicu, I. D., Tivig, I., Kuncser, A. C., Stefan, M., et al. (2020). Influence of surfactant-tailored Mn-doped ZnO nanoparticles on ROS production and DNA damage induced in murine fibroblast cells. Sci. Rep. 10, 18062. doi:10.1038/s41598-020-74816-0
Pradeep, K. R., and Viswanatha, R. (2020). Mechanism of Mn emission: Energy transfer vs charge transfer dynamics in Mn-doped quantum dots. Apl. Mater 8, 020901. doi:10.1063/1.5140888
Pradhan, N. (2019). Mn-doped semiconductor nanocrystals: 25 Years and beyond. J. Phys. Chem. Lett. 10, 2574–2577. doi:10.1021/acs.jpclett.9b01107
Reinoso, D. M., Damiani, D. E., and Tonetto, G. M. (2014). Synthesis of biodiesel from soybean oil using zinc layered hydroxide salts as heterogeneous catalysts. Catal. Sci. Technol. 4, 1803–1812. doi:10.1039/c4cy00145a
Ruiz, C. V., Becerra, M. E., and Giraldo, O. (2020). Structural, thermal, and release properties of hybrid materials based on layered zinc hydroxide and caffeic acid. Nanomaterials 10, 163. doi:10.3390/nano10010163
Shannon, R. D. (1976). Revised effective ionic radii and systematic studies of interatomic distances in halides and chalcogenides. Acta Cryst. A 32, 751–767. doi:10.1107/s0567739476001551
Sharma, P., Gupta, A., Rao, K. V., Owens, F. J., Sharma, R., Ahuja, R., et al. (2003). Ferromagnetism above room temperature in bulk and transparent thin films of Mn-doped ZnO. Nat. Mater 2, 673–677. doi:10.1038/nmat984
Sing, K. S. W., Everett, D. H., Haul, R. A. W., Moscou, L., Pierotti, R. A., Rouquerol, J., et al. (1985). Reporting physisorption data for gas/solid systems with special reference to the determination of surface area and porosity (Recommendations 1984). Pure Appl. Chem. 57, 603–619. doi:10.1351/pac198557040603
Singh, T., Mountziaris, T. J., and Maroudas, D. (2010). On the transition-metal doping efficiency of zinc oxide nanocrystals. Appl. Phys. Lett. 97, 073120. doi:10.1063/1.3478216
Stählin, W., and Oswald, H. R. (1971). The infrared spectrum and thermal analysis of zinc hydroxide nitrate. J. Sol. State Chem. 2, 252–255. doi:10.1016/0022-4596(71)90037-5
Stählin, W., and Oswald, H. (1970). The crystal structure of zinc hydroxide nitrate, Zn5(OH)8(NO3)2·2H2O. Acta Cryst. B 26, 860–863. doi:10.1107/s0567740870003230
Stefan, M., Nistor, S. V., and Ghica, D. (2013). Correlation of lattice disorder with crystallite size and the growth kinetics of Mn2+ doped ZnO nanocrystals probed by electron paramagnetic resonance. Cryst. Growth Des. 13, 1350–1359. doi:10.1021/cg400037c
Stefan, M., Nistor, S. V., and Ghica, D. (2014). “ZnS and ZnO semiconductor nanoparticles doped with Mn2+ ions. Size effects investigated by EPR spectroscopy,” in Size effects in nanostructures. Basics and application. Editors V. Kuncser, and L. Miu (Germany: Springer-Verlag Berlin Heidelberg), 3–27.
Stoll, S., and Schweiger, A. (2006). EasySpin, a comprehensive software package for spectral simulation and analysis in EPR. J. Magn. Reson. 178, 42–55. doi:10.1016/j.jmr.2005.08.013
Verma, R., Pathak, S., Srivastava, A. K., Prawer, S., and Tomljenovic-Hanic, S. (2021). ZnO nanomaterials: Green synthesis, toxicity evaluation and new insights in biomedical applications. J. Alloys Compd. 876, 160175. doi:10.1016/j.jallcom.2021.160175
Wypych, F., Arízaga, G. G. C., and da Costa Gardolinski, J. E. F. (2005). Intercalation and functionalization of zinc hydroxide nitrate with mono- and dicarboxylic acids. J. Colloid Interface Sci. 283, 130–138. doi:10.1016/j.jcis.2004.08.125
Yang, Y., Li, Y., Zhu, L., He, H., Hu, L., Huang, J., et al. (2013). Shape control of colloidal Mn doped ZnO nanocrystals and their visible light photocatalytic properties. Nanoscale 5, 10461–10471. doi:10.1039/c3nr03160h
Keywords: zinc hydroxynitrate, nanocrystals, electron paramagnetic resonance, Mn2+ paramagnetic probe, morpho-structural analysis, structural phase transformation
Citation: Vlaicu ID, Stefan M, Radu C, Culita DC, Radu D and Ghica D (2023) Atomic scale insight into the decomposition of nanocrystalline zinc hydroxynitrate toward ZnO using Mn2+ paramagnetic probes. Front. Chem. 11:1154219. doi: 10.3389/fchem.2023.1154219
Received: 30 January 2023; Accepted: 28 March 2023;
Published: 06 April 2023.
Edited by:
Rajni Verma, The University of Melbourne, AustraliaReviewed by:
Sandeep Sharma, Guru Nanak Dev University, IndiaSaurabh Pathak, Seoul National University, Republic of Korea
Komal Jain, National Physical Laboratory (CSIR), India
Copyright © 2023 Vlaicu, Stefan, Radu, Culita, Radu and Ghica. This is an open-access article distributed under the terms of the Creative Commons Attribution License (CC BY). The use, distribution or reproduction in other forums is permitted, provided the original author(s) and the copyright owner(s) are credited and that the original publication in this journal is cited, in accordance with accepted academic practice. No use, distribution or reproduction is permitted which does not comply with these terms.
*Correspondence: Daniela Ghica, Z2hpY2FAaW5maW0ucm8=