- Laboratory of Organic Chemistry, Wageningen University, Wageningen, Netherlands
Boronic esters prepared by condensation of boronic acids and diols have been widely used as dynamic covalent bonds in the synthesis of both discrete assemblies and polymer networks. In this study we investigate the potential of a new dynamic-covalent motif, derived from TetraAzaADamantanes (TAADs), with their adamantane-like triol structure, in boronic ester-based covalent adaptable networks (CANs). The TetraAzaADamantane-boronic ester linkage has recently been reported as a more hydrolytically stable boronic ester variant, while still having a dynamic pH response: small-molecule studies found little exchange at neutral pH, while fast exchange occurred at pH 3.8. In this work, bi- and trifunctional TetraAzaADamantane linkers were synthesised and crosslinked with boronic acids to form rubber-like materials, with a Young’s modulus of 1.75 MPa. The dynamic nature of the TetraAzaADamantane networks was confirmed by stress relaxation experiments, revealing Arrhenius-like behaviour, with a corresponding activation energy of 142 ± 10 kJ/mol. Increasing the crosslinking density of the material from 10% to 33% resulted in reduced relaxation times, as is consistent with a higher degree of crosslinking within the dynamic networks. In contrast to the reported accelerating effect of acid addition to small-molecule TetraAzaADamantane complexes, within the polymer network the addition of acid increased relaxation times, suggesting unanticipated interactions between the acid and the polymer that cannot occur in the corresponding small-molecules analogues. The obtained boronate-TetraAzaADamantane materials were thermally stable up to 150°C. This thermal stability, in combination with the intrinsically dynamic bonds inside the polymer network, allowed these materials to be reprocessed and healed after damage by hot-pressing.
Introduction
The poor recyclability of most modern plastics, in particular of thermoset materials, has created a focus on making more sustainable and better recyclable materials. This recyclability issue can be tackled by incorporating dynamic covalent bonds (Rowan et al., 2002) inside crosslinked polymer materials to create so-called covalent adaptable networks (CANs) (Kloxin and Bowman, 2013; Winne et al., 2019; Bowman et al., 2020; Zheng et al., 2021). While dynamic covalent bonds have proven very successful in the creation of dynamic combinatorial libraries (Hamieh et al., 2013; Mondal and Hirsch, 2015), discrete cage(-like) assemblies (Mastalerz, 2010), or covalent organic frameworks (Hu et al., 2020), their use in polymer networks has only more recently been acknowledged (Kloxin et al., 2010). Instead, polymer chemists previously focussed primarily on reversible, non-covalent interactions (notably hydrogen bonds) (Yang et al., 2015). However, by utilising dynamic covalent bonds, a more robust network can be created with crosslinks that can be made to exchange under specific conditions (e.g., by application of heat or light), thus allowing for reprocessing and recycling. The dynamicity of the introduced reversible crosslinks also means that the polymer material can undergo self-healing, which can extend the lifetime of the material (Kissounko et al., 2018; Zhang et al., 2018; Hayashi, 2020). Crucially, the use of dynamic bonds that are covalent in nature means that while these CANs can be recycled and/or demonstrate self-healing behaviour, at their operating temperature they still form a robust network–as a direct consequence of these covalent crosslinks–that impart mechanical strength to the material. Over the last few decades various dynamic bonds have been explored for use in CANs; these include the Diels-Alder reaction (Briou et al., 2021; Gaina et al., 2021), imines (Taynton et al., 2014; Schoustra et al., 2020), esters (Montarnal et al., 2011; Liu et al., 2020) and vinylogous urethanes (Denissen et al., 2015). Also, boronic acids (Bapat et al., 2020; Gosecki and Gosecka, 2022) have received an increasing amount of interest, due to their dynamic reversible condensation reaction with diols and general biocompatibility (Yang et al., 2005; Cambre and Sumerlin, 2011; Banach et al., 2021). Coupled with the affinity of boronic acids towards catechols (He et al., 2011) and sugars (Cai et al., 2017; Geethanjali et al., 2017), this has led to various applications to include boronic acids in medical applications, such as drugs (Plescia and Moitessier, 2020) and medical gels (Yesilyurt et al., 2016; Marco-Dufort and Tibbitt, 2019; Amaral et al., 2020), and biodegradable materials (Li et al., 2013; Zych et al., 2021). Boronic esters have also been used to form dynamic assemblies such as micelles (Zhao et al., 2014; Zhang et al., 2020a) or cage structures (Takata et al., 2020; Giraldi et al., 2021). Even a dynamically self-assembled nanoscale boronate ester ladder has been reported (Drogkaris and Northrop, 2020).
Typically, bidentate boronic acid linkages are used via reactions with diols or poly-ols, which usually result in the formation of soft (hydro)gels (Roberts et al., 2007; Wang et al., 2018; Perera and Ayres, 2020; Yang et al., 2021). However an interesting moiety (Semakin et al., 2009) was found to be able to consistently give rigid tridentate complexes of boronic acids, due to its well defined adamantane-like structure and conformation. This triol was named TetraAzaADamantane (TAAD) and is shown in Figure 1. TAAD can be obtained from the precursor tris-oxime named TRISOXH3 (Golovanov et al., 2018), which is prepared in a one-pot synthesis, after which an acid is used to catalyse the conversion into the final adamantane-like conformation (see Figure 1).
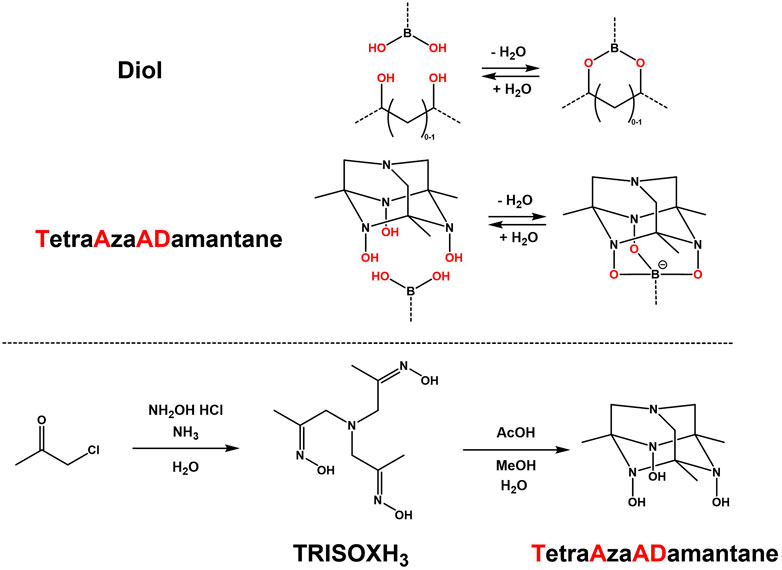
FIGURE 1. Comparison between (conventional) bidentate complexation of a boronic acid with a diol, and novel tridentate complexation by TetraAzaADamantane. Below, the synthesis pathway of tris-oxime TRISOXH3 and subsequent conversion into TetraAzaADamantane as reported (Semakin et al., 2009).
This TAAD moiety was then further investigated (Golovanov et al., 2015; Golovanov et al., 2018). It was found that the tridentate boronate complexation was stable at neutral to high pH with little dynamic exchange. However, around pH 3-4 dynamic exchange of the boronate groups was triggered, while at even lower pH complete disassembly of the boronate linkages was observed (Golovanov et al., 2018). Coupled with a quick and simple synthesis, and the more rigid multicyclic TAAD structure, we envisioned that this dynamic response makes the TAAD moiety an interesting candidate for use in CANs to create novel boronic acid-based materials.
In this work, we investigate the potential of the TAAD moiety in dynamic CANs. To this end, bifunctional boronic acid linkers and bi- and trifunctional TAAD linkers were designed and synthesised, as shown in Figure 2. These linkers were then reacted to form a crosslinked dynamic network. We found that the TAAD moieties can be successfully used for the formation of CANs. By using a small molecule network approach soft, rubber-like materials were prepared that were suitable for thermal reprocessing without the need for additional solvent and that could undergo stress relaxation.
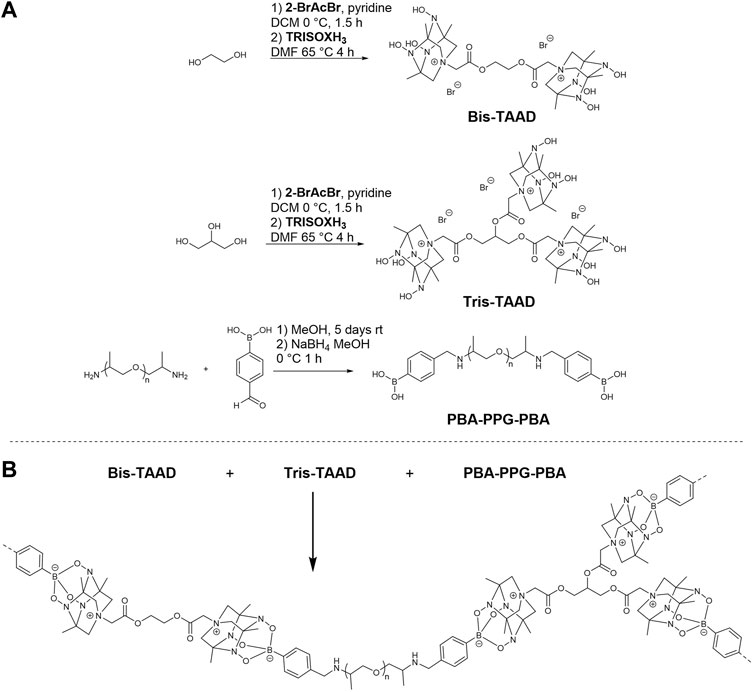
FIGURE 2. Synthesis and structure of the phenylboronic acid and TAAD linkers used in the boronate network (A), and synthesis of the TAAD-based polymer networks (B). For clarity, the counter anions have been omitted in the bottom figure.
Results and discussion
To incorporate TetraAzaADamantane (TAAD) groups in a boronate ester network, different linker molecules were synthesised. Both a linear bifunctional boronic acid and TAAD linker were prepared, as well as a trifunctional TAAD linker to enable crosslinking. For the linear boronic acid crosslinker it was chosen to react bis-amine terminated PPG2000 with 4-formylphenylboronic acid and subsequently reducing the formed imine bond (Bao et al., 2018). For the bi- and trifunctional TAAD linkers Bis-TAAD and Tris-TAAD, ethylene glycol and glycerol, respectively, were reacted with bromoacetyl bromide. After a short work up, TRISOXH3 was attached via nitrogen quaternisation and converted to the desired TAAD-containing building blocks (see Supplementary Material for full details of the synthetic procedures and characterisation).
To show the reversibility and exchange of the boronate-TAAD bond a small series of exchange studies was performed using differently substituted boronic acids, expanding on the existing exchange experiment involving only 4-bromophenylboronic acid (Golovanov et al., 2018). A model TAAD molecule coupled to a phenylboronic acid, resembling the boronate-TAAD linkages used in the materials, was synthesised and exchanged with three different para-substituted phenylboronic acids (see Supplementary Material). This exchange was followed using 1H-NMR spectroscopy, where both the disappearance of the signals corresponding to starting boronate-TAAD and the appearance of signals of the exchanged product were monitored in time. All showed slow exchange over the course of 3 days, resulting in only ∼20% conversion. Subsequently, the pH was adjusted to 3.8 to enter the dynamic pH regime and the exchange reaction was followed for 5 h. All three substituted phenylboronic acids showed an increase in exchange rate as expected (Supplementary Figures S35–S38), reaching 40%–60% conversion after only 5 h. This shows that the boronate-TAAD linkages that are to be incorporated in the network can undergo exchange reactions.
Next, polymer materials were prepared by dissolving the Bis-TAAD, Tris-TAAD, and PBA-PPG-PBA in separate methanol stock solutions. These stock solutions were then combined using a stoichiometry TAAD:boronic acid of 1:1, and initially with 10% of the crosslinker Tris-TAAD. The resulting mixture was vortexed, then cast in a silicone mould and left to dry in the fume hood for 2 days. Using this method, a soft rubber-like material was obtained that was slightly yellowish in colour. Initial characterisation by thermal gravimetric analysis (TGA, Supplementary Figure S65) showed that the material was thermally stable up to 153°C with only 5% weight loss. This thermal stability suggests that (re)processing of the material at elevated temperatures (e.g., by hot-pressing) can be explored up to temperatures of approximately 150°C. Below, we will further discuss the thermal (re)processing by hot-pressing.
The obtained polymer was then further characterised by rheometry. A frequency sweep of the material, as shown in Figure 3A, yielded a storage modulus (G′) and loss modulus (G″) between 0.1 and 1 MPa over the frequency domain of 0.01–100 rad/s. Extensional DMA showed that the material had a Young’s modulus of 1.75 MPa, corresponding to a soft rubber. The temperature sweep from 4°C to 170°C (Figure 3B) of the formed network showed a typical rubbery plateau at a GP of 1 MPa between 70°C and 125°C suggesting an entangled or crosslinked network, after which the material started to flow. The glassy state was not observed, but could likely be observed at lower temperatures.
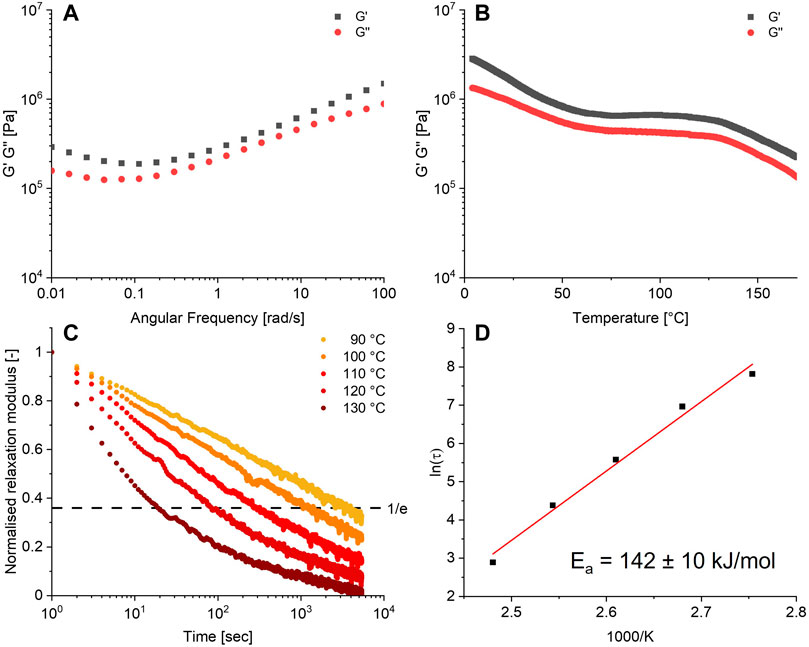
FIGURE 3. (A) Frequency sweep at 0.5% strain, 25°C. (B) Temperature sweep at 0.5% strain, 6.28 rad/s. (C) Relaxation series at 0.5% strain, 90°C–130°C. (D) Arrhenius plot obtained from the relaxation data. All data were obtained from the 10% crosslinked material.
A defining feature of CANs is their ability to undergo stress relaxation by dynamic bond exchange. The time for the material to relax imposed stress generally shortens with an increase in temperature, since the dynamic bond exchange becomes faster. This allows faster network rearrangement and thus faster stress relaxation. The Arrhenius equation can be applied to the dependence of the relaxation time (τ) with temperature, which will yield the activation energy Ea of relaxation process due to bond exchange. To this end, the relaxation behaviour of the boronate-TAAD network was investigated in the temperature range of 90°C–130°C. The observed relaxation time τ decreased from 2,478 s at 90°C to 18 s at 130°C, as can be seen in Figure 3C. From the corresponding Arrhenius plot (Figure 3D) for the boronate-TAAD exchange reaction an activation energy of 142 ± 10 kJ/mol could be determined, which is quite high compared to previously reported materials based on boronic esters (Cromwell et al., 2015; Röttger et al., 2017; Zhang et al., 2020b; Kang and Kalow, 2022) and more in the upper range of diethanolamine esters (Zhang et al., 2020b; Gosecki and Gosecka, 2022). However, it is important to note that most reported boronic ester materials were prepared as a (hydro)gel, which can heavily influence the determined activation energy. Remarkably, in contrast to the pH range of 3-4 necessary for dynamic exchange in the reactions (Golovanov et al., 2018), within this dynamic network, (stress) relaxation already manifested itself at neutral pH.
Having established that a TAAD-based CAN could be prepared with a 10% crosslinker content, the effect of the crosslink density on the CAN’s material properties was investigated. Additional CANs with 20% and 33% crosslinks were prepared and compared. Frequency sweeps (Supplementary Figures S39, S42) did not show a significant change to the moduli as G′ and G″ of the different materials were in the range of 0.1–1 MPa. Temperature sweeps (Supplementary Figures S41, S44) did show that increasing the crosslinking density shifted the onset of the rubbery plateau from around 50 °C to around 120°C–130 °C, which can be explained by considering the higher crosslink density. Next to this, the relaxation time (τ) of the material increased by one order of magnitude with increasing crosslink density from 10% to 33%, as can be seen in Figure 4A. The added crosslinks reduce network mobility, thus slowing the material relaxation process (Hajj et al., 2020). This shows that it is possible to tune material properties by varying the crosslink density of these networks.
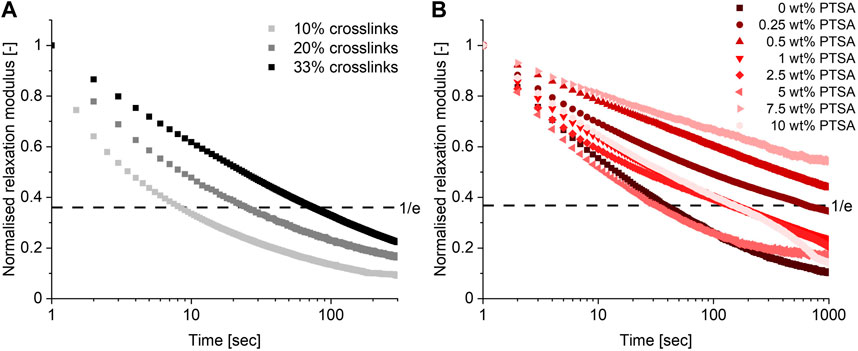
FIGURE 4. Relaxation curves (at 0.1% strain, 25°C) of materials with varying crosslink densities (A) and the effect of acid addition (at 0.1% strain, 25°C) on the material properties (B).
Since the boronate-TAAD network features pH-dependent bonds (Golovanov et al., 2018), also the effect of acid addition to the material was investigated. Previously, researchers showed that addition of acid can accelerate the dynamic bond exchange processes inside a CAN (Denissen et al., 2017; Self et al., 2018; Spiesschaert et al., 2020). In these reports, the pKa of the added acid was found to correlate with the shortening of the material relaxation time. Combined with the reported findings (Golovanov et al., 2018) of the different pH regimes of the boronate-TAAD linkages, we anticipated that addition of acid in the material would have an effect by reducing the relaxation time of the material, via transesterification catalysis.
To investigate the effect of acid on this boronate-TAAD network a series of materials was prepared by mixing in different weight percentages of p-toluenesulfonic acid (PTSA), ranging from 0.25 wt% to 10 wt%. The relaxation behaviour of these materials was then measured and evaluated, as shown in Figure 4B. The observed relaxation behaviour was more complex than initially anticipated: i.e., the addition of acid did not simply lead to faster relaxation. In contrast, at low PTSA content (0.25–0.5 wt%) an increase in the relaxation time of the material compared to the material without PTSA was observed. These materials displayed a relaxation time that is about one order of magnitude longer than the original acid-free material. At moderate PTSA content (1–5 wt%) the relaxation time is still generally longer than without PTSA, but it started to decrease until at 5 wt% PTSA the material had a relaxation time similar to the control without PTSA. At high PTSA content (5–10 wt%) again an increase in relaxation time was observed. While the precise underlying mechanism of these trends remains unclear, one possible explanation might be that the PTSA is partially excluded from the network due to unfavourable interactions between the apolar aromatic ring of the acid and the polar building blocks, thus reducing its effectiveness as a catalyst while possibly introducing crystalline regions in the matrix. Another possible explanation could be an interaction with the secondary amines of the PBA-PPG-PBA linker. PTSA could protonate the secondary amines of this linker, resulting in the formation of charged nitrogen species similar to polyelectrolyte complexes, which often become stiff and brittle upon drying (Fares et al., 2019; Krishna et al., 2022). This unexpected acid response in the material relaxation shows that, although the characteristics of this boronate-TAAD network can be tuned through acid addition, predicting the resulting material behaviour might prove complex. Fortunately, since the control material was already quite dynamic even at neutral pH there was little need for additional acid catalysis. More generally, it also serves as a reminder that translating molecular processes (i.e., acid-catalysed transesterification) to the macroscopic material level, is not always straightforwardly possible, as other macroscopic phenomena, such as crystallisation, can affect the process.
Another interesting observation could be seen in the temperature sweeps of these materials, where higher PTSA weight percentages resulted in the material melting at high temperatures, as shown by the loss factor steeply increasing (Supplementary Figure S64) This was not observed for the control material without PTSA.
The self-healing behaviour and recyclability of the material, as might be enabled by the dynamic bond exchange within the network, was also investigated using extensional DMA. The strain at which material breakage occurred was measured by linearly increasing the extensional stress of a CAN with 10% crosslinker. After breakage the material was cut in fine pieces and hot-pressed for 1 h at 80°C to allow the material to heal. As can be seen in Figure 5, the material is able to completely heal after multiple reprocessing cycles. The strengthening of the last few healing cycles seems to suggest that the network configuration is optimised during the applied thermal treatment.
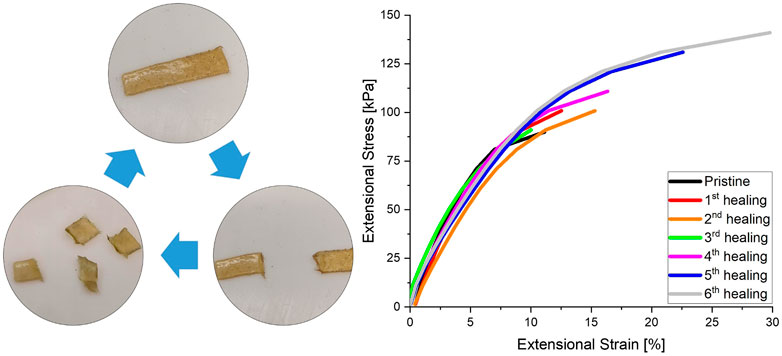
FIGURE 5. Healing cycles performed by extensional DMA testing. Reprocessing was done by hot-pressing for 1 h at 80°C in a Teflon mould (10 × 5 × 1 mm). DMA was performed by linearly increasing the extensional stress till material breakage. After breakage the sample was cut into small pieces before reprocessing.
Conclusion
In this study we synthesised a novel covalent adaptable network incorporating dynamic boronate-TAAD linkages using a small molecule network approach, resulting in a soft, rubber-like material. The material was thermally stable up to 150°C as determined by TGA, thus allowing for hot-press moulding. In contrast to the behaviour of previously reported small-molecule boronate-TAAD complexes that could only undergo (fast) exchange under acidic conditions (pH 3.8), the corresponding CANs could already undergo (stress) relaxation under neutral conditions. Surprisingly, the addition of acid did not have a further accelerating effect on the exchange. The boronate-TAAD material showed complete self-healing after multiple thermal reprocessing cycles and Arrhenius analysis gave a relatively high activation energy of 142 ± 10 kJ/mol for the crosslink relaxation process compared with regular boronic esters.
This first investigation into boronate-TAAD networks showed that the novel boronate-TAAD linkages can indeed be used to develop CANs. Coupled to the relatively simple synthesis of the TAAD moiety, this unique boronate-TAAD linkage offers an interesting alternative to the more commonly used boronic esters.
Data availability statement
The raw data supporting the conclusions of this article will be made available by the authors, without undue reservation.
Author contributions
SH and MK synthesised the building blocks. SH prepared and tested the materials. SH wrote the first draft of the manuscript. SH and MS contributed to manuscript revisions. MS secured funding for the work.
Funding
This research was funded by the Netherlands Organisation for Scientific Research (NWO) (NWO Vidi grant 016.Vidi.189.031 to MS).
Acknowledgments
We would like to thank Dr. Alexey Sukhorukov at the Zelinksy Institute of Organic Chemistry for fruitful discussions regarding the TAAD moiety. Prof. Dr. Han Zuilhof, Dr. Fedor Miloserdov, Dr. Sagar Kumar Raut, and Sybren Schoustra are thanked for their help and involvement in this project. The Netherlands Organisation for Scientific Research (NWO) is acknowledged for funding (NWO Vidi grant 016.Vidi.189.031 to MS).
Conflict of interest
The authors declare that the research was conducted in the absence of any commercial or financial relationships that could be construed as a potential conflict of interest.
Publisher’s note
All claims expressed in this article are solely those of the authors and do not necessarily represent those of their affiliated organizations, or those of the publisher, the editors and the reviewers. Any product that may be evaluated in this article, or claim that may be made by its manufacturer, is not guaranteed or endorsed by the publisher.
Supplementary material
The Supplementary Material for this article can be found online at: https://www.frontiersin.org/articles/10.3389/fchem.2023.1148629/full#supplementary-material
References
Amaral, A. J. R., Gaspar, V. M., and Mano, J. F. (2020). Responsive laminarin-boronic acid self-healing hydrogels for biomedical applications. Polym. J. 52 (8), 997–1006. doi:10.1038/s41428-020-0348-3
Banach, L., Williams, G. T., and Fossey, J. S. (2021). Insulin delivery using dynamic covalent boronic acid/ester-controlled release. Adv. Ther. 4 (11), 2100118. doi:10.1002/adtp.202100118
Bao, C. Y., Jiang, Y. J., Zhang, H. Y., Lu, X. Y., and Sun, J. Q. (2018). Room-temperature self-healing and recyclable tough polymer composites using nitrogen-coordinated boroxines. Adv. Funct. Mat. 28 (23), 1800560. doi:10.1002/adfm.201800560
Bapat, A. P., Sumerlin, B. S., and Sutti, A. (2020). Bulk network polymers with dynamic B–O bonds: Healable and reprocessable materials. Mat. Horiz. 7 (3), 694–714. doi:10.1039/c9mh01223k
Bowman, C., Du Prez, F., and Kalow, J. (2020). Introduction to chemistry for covalent adaptable networks. Polym. Chem. 11 (33), 5295–5296. doi:10.1039/d0py90102d
Briou, B., Ameduri, B., and Boutevin, B. (2021). Trends in the Diels-Alder reaction in polymer chemistry. Chem. Soc. Rev. 50 (19), 11055–11097. doi:10.1039/d0cs01382j
Cai, B., Luo, Y., Guo, Q., Zhang, X., and Wu, Z. (2017). A glucose-sensitive block glycopolymer hydrogel based on dynamic boronic ester bonds for insulin delivery. Carbohydr. Res. 445, 32–39. doi:10.1016/j.carres.2017.04.006
Cambre, J. N., and Sumerlin, B. S. (2011). Biomedical applications of boronic acid polymers. Polymer 52 (21), 4631–4643. doi:10.1016/j.polymer.2011.07.057
Cromwell, O. R., Chung, J., and Guan, Z. (2015). Malleable and self-healing covalent polymer networks through tunable dynamic boronic ester bonds. J. Am. Chem. Soc. 137 (20), 6492–6495. doi:10.1021/jacs.5b03551
Denissen, W., Droesbeke, M., Nicolay, R., Leibler, L., Winne, J. M., and Du Prez, F. E. (2017). Chemical control of the viscoelastic properties of vinylogous urethane vitrimers. Nat. Commun. 8 (1), 14857. doi:10.1038/ncomms14857
Denissen, W., Rivero, G., Nicolay, R., Leibler, L., Winne, J. M., and Du Prez, F. E. (2015). Vinylogous urethane vitrimers. Adv. Funct. Mat. 25 (16), 2451–2457. doi:10.1002/adfm.201404553
Drogkaris, V., and Northrop, B. H. (2020). Discrete boronate ester ladders from the dynamic covalent self-assembly of oligo(phenylene ethynylene) derivatives and phenylenebis(boronic acid). Org. Chem. Front. 7 (9), 1082–1094. doi:10.1039/d0qo00083c
Fares, H. M., Wang, Q. F., Yang, M., and Schlenoff, J. B. (2019). Swelling and inflation in polyelectrolyte complexes. Macromolecules 52 (2), 610–619. doi:10.1021/acs.macromol.8b01838
Gaina, V., Nechifor, M., Gaina, C., and Ursache, O. (2021). Maleimides - a versatile platform for polymeric materials designed/tailored for high performance applications. Polym.-Plast. Tech. Mat. 60 (3), 253–270. doi:10.1080/25740881.2020.1811315
Geethanjali, H. S., Melavanki, R. M., and Kusanur, R. A. (2017). Binding of boronic acids with sugars in aqueous solution at physiological pH - estimation of association and dissociation constants using spectroscopic method. J. Mol. Liq. 227, 37–43. doi:10.1016/j.molliq.2016.11.097
Giraldi, E., Scopelliti, R., Fadaei-Tirani, F., and Severin, K. (2021). Metal-stabilized boronate ester cages. Inorg. Chem. 60 (15), 10873–10879. doi:10.1021/acs.inorgchem.1c01719
Golovanov, I. S., Mazeina, G. S., Nelyubina, Y. V., Novikov, R. A., Mazur, A. S., Britvin, S. N., et al. (2018). Exploiting coupling of boronic acids with triols for a pH-dependent "Click-Declick" chemistry. J. Org. Chem. 83 (17), 9756–9773. doi:10.1021/acs.joc.8b01296
Golovanov, I. S., Sukhorukov, A. Y., Nelyubina, Y. V., Khomutova, Y. A., Ioffe, S. L., and Tartakovsky, V. A. (2015). Synthesis of B,O,N-doped adamantanes and diamantanes by condensation of oximes with boronic acids. J. Org. Chem. 80 (13), 6728–6736. doi:10.1021/acs.joc.5b00892
Gosecki, M., and Gosecka, M. (2022). Boronic acid esters and anhydrates as dynamic cross-links in vitrimers. Polymers 14 (4), 842. doi:10.3390/polym14040842
Hajj, R., Duval, A., Dhers, S., and Averous, L. (2020). Network design to control polyimine vitrimer properties: Physical versus chemical approach. Macromolecules 53 (10), 3796–3805. doi:10.1021/acs.macromol.0c00453
Hamieh, S., Saggiomo, V., Nowak, P., Mattia, E., Ludlow, R. F., and Otto, S. (2013). A "dial-a-receptor" dynamic combinatorial library. Angew. Chem. Int. Ed. Engl. 52 (47), 12368–12372. doi:10.1002/anie.201305744
Hayashi, M. (2020). Implantation of recyclability and healability into cross-linked commercial polymers by applying the vitrimer concept. Polymers 12 (6), 1322. doi:10.3390/polym12061322
He, L., Fullenkamp, D. E., Rivera, J. G., and Messersmith, P. B. (2011). pH responsive self-healing hydrogels formed by boronate-catechol complexation. Chem. Commun. 47 (26), 7497–7499. doi:10.1039/c1cc11928a
Hu, J., Gupta, S. K., Ozdemir, J., and Beyzavi, M. H. (2020). Applications of dynamic covalent chemistry concept toward tailored covalent organic framework nanomaterials: A review. ACS Appl. Nano Mat. 3 (7), 6239–6269. doi:10.1021/acsanm.0c01327
Kang, B., and Kalow, J. (2022). Internal and external catalysis in boronic ester networks. ACS macro Lett. 11 (3), 394–401. doi:10.1021/acsmacrolett.2c00056
Kissounko, D. A., Taynton, P., and Kaffer, C. (2018). New material: Vitrimers promise to impact composites. Reinf. Plast. 62 (3), 162–166. doi:10.1016/j.repl.2017.06.084
Kloxin, C. J., and Bowman, C. N. (2013). Covalent adaptable networks: Smart, reconfigurable and responsive network systems. Chem. Soc. Rev. 42 (17), 7161–7173. doi:10.1039/c3cs60046g
Kloxin, C. J., Scott, T. F., Adzima, B. J., and Bowman, C. N. (2010). Covalent adaptable networks (CANs): A unique paradigm in crosslinked polymers. Macromolecules 43 (6), 2643–2653. doi:10.1021/ma902596s
Krishna, A., Willott, J. D., Lindhoud, S., and de Vos, W. M. (2022). Hot-pressing polyelectrolyte complexes into tunable dense saloplastics. Polymer 242, 124583. doi:10.1016/j.polymer.2022.124583
Li, L., Bai, Z., and Levkin, P. A. (2013). Boronate-dextran: An acid-responsive biodegradable polymer for drug delivery. Biomaterials 34 (33), 8504–8510. doi:10.1016/j.biomaterials.2013.07.053
Liu, T., Zhao, B. M., and Zhang, J. W. (2020). Recent development of repairable, malleable and recyclable thermosetting polymers through dynamic transesterification. Polymer 194, 122392. doi:10.1016/j.polymer.2020.122392
Marco-Dufort, B., and Tibbitt, M. W. (2019). Design of moldable hydrogels for biomedical applications using dynamic covalent boronic esters. Mat. Today Chem. 12, 16–33. doi:10.1016/j.mtchem.2018.12.001
Mastalerz, M. (2010). Shape-persistent organic cage compounds by dynamic covalent bond formation. Angew. Chem. Int. Ed. Engl. 49 (30), 5042–5053. doi:10.1002/anie.201000443
Mondal, M., and Hirsch, A. K. (2015). Dynamic combinatorial chemistry: A tool to facilitate the identification of inhibitors for protein targets. Chem. Soc. Rev. 44 (8), 2455–2488. doi:10.1039/c4cs00493k
Montarnal, D., Capelot, M., Tournilhac, F., and Leibler, L. (2011). Silica-like malleable materials from permanent organic networks. Science 334 (6058), 965–968. doi:10.1126/science.1212648
Perera, M. M., and Ayres, N. (2020). Dynamic covalent bonds in self-healing, shape memory, and controllable stiffness hydrogels. Polym. Chem. 11 (8), 1410–1423. doi:10.1039/c9py01694e
Plescia, J., and Moitessier, N. (2020). Design and discovery of boronic acid drugs. Eur. J. Med. Chem. 195, 112270. doi:10.1016/j.ejmech.2020.112270
Roberts, M. C., Hanson, M. C., Massey, A. P., Karren, E. A., and Kiser, P. F. (2007). Dynamically restructuring hydrogel networks formed with reversible covalent crosslinks. Adv. Mat. 19 (18), 2503–2507. doi:10.1002/adma.200602649
Röttger, M., Domenech, T., van der Weegen, R., Breuillac, A., Nicolaÿ, R., and Leibler, L. (2017). High-performance vitrimers from commodity thermoplastics through dioxaborolane metathesis. Science 356 (6333), 62–65. doi:10.1126/science.aah5281
Rowan, S. J., Cantrill, S. J., Cousins, G. R., Sanders, J. K., and Stoddart, J. F. (2002). Dynamic covalent chemistry. Angew. Chem. Int. Ed. Engl. 41 (6), 898–952. doi:10.1002/1521-3773(20020315)41:6<898::aid-anie898>3.0.co;2-e
Schoustra, S. K., Dijksman, J. A., Zuilhof, H., and Smulders, M. M. J. (2020). Molecular control over vitrimer-like mechanics - tuneable dynamic motifs based on the Hammett equation in polyimine materials. Chem. Sci. 12 (1), 293–302. doi:10.1039/d0sc05458e
Self, J. L., Dolinski, N. D., Zayas, M. S., Read de Alaniz, J., and Bates, C. M. (2018). Bronsted-acid-catalyzed exchange in polyester dynamic covalent networks. ACS Macro Lett. 7 (7), 817–821. doi:10.1021/acsmacrolett.8b00370
Semakin, A. N., Sukhorukov, A. Y., Lesiv, A. V., Ioffe, S. L., Lyssenko, K. A., Nelyubina, Y. V., et al. (2009). Unusual intramolecular cyclization of tris(β-oximinoalkyl)amines. The first synthesis of 1,4,6,10-tetraazaadamantanes. Org. Lett. 11 (18), 4072–4075. doi:10.1021/ol9015157
Spiesschaert, Y., Taplan, C., Stricker, L., Guerre, M., Winne, J. M., and Du Prez, F. E. (2020). Influence of the polymer matrix on the viscoelastic behaviour of vitrimers. Polym. Chem. 11 (33), 5377–5385. doi:10.1039/d0py00114g
Takata, H., Ono, K., and Iwasawa, N. (2020). Controlled release of the guest molecule via borate formation of a fluorinated boronic ester cage. Chem. Commun. 56 (42), 5613–5616. doi:10.1039/d0cc01441a
Taynton, P., Yu, K., Shoemaker, R. K., Jin, Y., Qi, H. J., and Zhang, W. (2014). Heat- or water-driven malleability in a highly recyclable covalent network polymer. Adv. Mat. 26 (23), 3938–3942. doi:10.1002/adma.201400317
Wang, W., Narain, R., and Zeng, H. (2018). Rational design of self-healing tough hydrogels: A mini review. Front. Chem. 6, 497. doi:10.3389/fchem.2018.00497
Winne, J. M., Leibler, L., and Du Prez, F. E. (2019). Dynamic covalent chemistry in polymer networks: A mechanistic perspective. Polym. Chem. 10 (45), 6091–6108. doi:10.1039/c9py01260e
Yang, H. S., Cho, S., Eom, Y., Park, S. A., Hwang, S. Y., Jeon, H., et al. (2021). Preparation of self-healable and spinnable hydrogel by dynamic boronate ester bond from hyperbranched polyglycerol and boronic acid-containing polymer. Macromol. Res. 29 (2), 140–148. doi:10.1007/s13233-021-9016-5
Yang, L., Tan, X., Wang, Z., and Zhang, X. (2015). Supramolecular polymers: Historical development, preparation, characterization, and functions. Chem. Rev. 115 (15), 7196–7239. doi:10.1021/cr500633b
Yang, W., Gao, X., and Wang, B. (2005). Biological and medicinal applications of boronic acids. Netherlands: John Wiley and Sons.
Yesilyurt, V., Webber, M. J., Appel, E. A., Godwin, C., Langer, R., and Anderson, D. G. (2016). Injectable self-healing glucose-responsive hydrogels with pH-regulated mechanical properties. Adv. Mater 28 (1), 86–91. doi:10.1002/adma.201502902
Zhang, X., Gao, J. S., Zhao, X. Y., Liu, Z. T., Liu, Z. W., Wang, K., et al. (2020a). Hyperbranched polymer micelles with triple-stimuli backbone-breakable iminoboronate ester linkages. Chin. Chem. Lett. 31 (7), 1822–1826. doi:10.1016/j.cclet.2020.03.018
Zhang, X., Wang, S., Jiang, Z., Li, Y., and Jing, X. (2020b). Boronic ester based vitrimers with enhanced stability via internal boron-nitrogen coordination. J. Am. Chem. Soc. 142 (52), 21852–21860. doi:10.1021/jacs.0c10244
Zhang, Z. P., Rong, M. Z., and Zhang, M. Q. (2018). Polymer engineering based on reversible covalent chemistry: A promising innovative pathway towards new materials and new functionalities. Prog. Polym. Sci. 80, 39–93. doi:10.1016/j.progpolymsci.2018.03.002
Zhao, Z., Yao, X., Zhang, Z., Chen, L., He, C., and Chen, X. (2014). Boronic acid shell-crosslinked dextran-b-PLA micelles for acid-responsive drug delivery. Macromol. Biosci. 14 (11), 1609–1618. doi:10.1002/mabi.201400251
Zheng, N., Xu, Y., Zhao, Q., and Xie, T. (2021). Dynamic covalent polymer networks: A molecular platform for designing functions beyond chemical recycling and self-healing. Chem. Rev. 121 (3), 1716–1745. doi:10.1021/acs.chemrev.0c00938
Keywords: dynamic covalent chemistry, covalent adaptable network (CAN), boronic acid, TetraAzaADamantane, polymer (re)processing
Citation: van Hurne S, Kisters M and Smulders MMJ (2023) Covalent adaptable networks using boronate linkages by incorporating TetraAzaADamantanes. Front. Chem. 11:1148629. doi: 10.3389/fchem.2023.1148629
Received: 20 January 2023; Accepted: 13 February 2023;
Published: 23 February 2023.
Edited by:
Fabien B. L. Cougnon, University of Jyväskylä, FinlandReviewed by:
Ghislaine Vantomme, Eindhoven University of Technology, NetherlandsClare Mahon, Durham University, United Kingdom
Copyright © 2023 van Hurne, Kisters and Smulders. This is an open-access article distributed under the terms of the Creative Commons Attribution License (CC BY). The use, distribution or reproduction in other forums is permitted, provided the original author(s) and the copyright owner(s) are credited and that the original publication in this journal is cited, in accordance with accepted academic practice. No use, distribution or reproduction is permitted which does not comply with these terms.
*Correspondence: Maarten M. J. Smulders, bWFhcnRlbi5zbXVsZGVyc0B3dXIubmw=