- 1Chemical Science Division, Lawrence Berkeley National Laboratory, Berkeley, CA, United States
- 2Advanced Light Source, Lawrence Berkeley National Laboratory, Berkeley, CA, United States
- 3Energy & Environment S&T, Idaho National Laboratory, Idaho Falls, ID, United States
The need to reduce atmospheric CO2 concentrations necessitates CO2 capture technologies for conversion into stable products or long-term storage. A single pot solution that simultaneously captures and converts CO2 could minimize additional costs and energy demands associated with CO2 transport, compression, and transient storage. While a variety of reduction products exist, currently, only conversion to C2+ products including ethanol and ethylene are economically advantageous. Cu-based catalysts have the best-known performance for CO2 electroreduction to C2+ products. Metal Organic Frameworks (MOFs) are touted for their carbon capture capacity. Thus, integrated Cu-based MOFs could be an ideal candidate for the one-pot capture and conversion. In this paper, we review Cu-based MOFs and MOF derivatives that have been used to synthesize C2+ products with the objective of understanding the mechanisms that enable synergistic capture and conversion. Furthermore, we discuss strategies based on the mechanistic insights that can be used to further enhance production. Finally, we discuss some of the challenges hindering widespread use of Cu-based MOFs and MOF derivatives along with possible solutions to overcome the challenges.
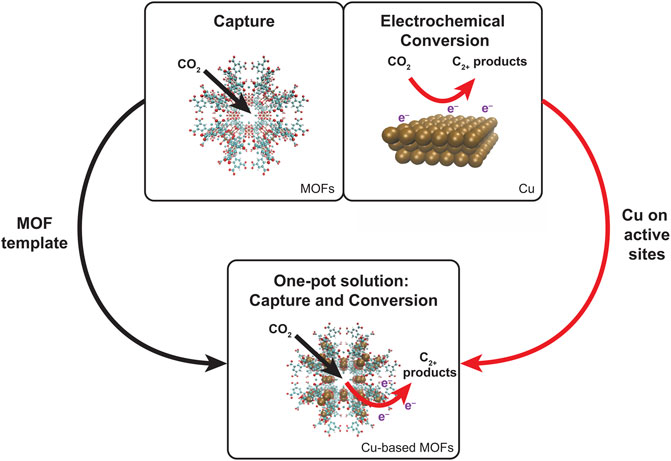
GRAPHICAL ABSTRACT | A one-pot solution of combined capture and conversion represents the ideal solution with the MOFs component aiding capture and the Cu constituents supporting conversion to C2+ products via CO2ER.
1 Introduction
The exponential increase in greenhouse gases (GHGs) including CO2 since the industrial revolution is the key driver of climate change (Field and Barros, 2014; Sun et al., 2017). Currently, the concentration of CO2 in the atmosphere is greater than 400 ppm (Jiao et al., 2019) with fossil fuels accounting for 75% of the increase in anthropogenic CO2 emissions (Field and Barros, 2014; Rafiee et al., 2018). CO2 levels are projected to increase to 685 ppm by 2050 (OECD, 2012) but CO2 levels need to be maintained at ≤ 450 ppm to avoid seriously jeopardizing the environment (Metz, 2007). While replacing fossil fuels with renewable sources of energy is the long-term solution, capturing CO2 from concentrated sources and from the atmosphere is urgently needed (Irena, 2018; Birol, 2019; Gielen et al., 2019). Three main separation technologies to capture CO2 are liquid absorbents, solid adsorbents, and membranes (‘Paul and Michelle, 2020: Report of the Basic Energy Sciences Workshop for Carbon Capture: Beyond 2020’, 2020) but the only method currently being used in an industrial scale is absorption using aqueous amine solutions (Iyer et al., 2017). However, it is fraught with issues like solvent losses and comes with a high sorbent regeneration cost. Thus, there is an ongoing search for better materials (and processes) that can achieve efficient and cost-effective carbon capture (‘Paul and Michelle, 2020: Report of the Basic Energy Sciences Workshop for Carbon Capture: Beyond 2020’, 2020). In this review, we evaluate a class of solid adsorbents in more detail- Metal Organic Frameworks (MOFs). These crystalline porous materials are composed of multi-metallic units surrounded by organic linkers (Eddaoudi et al., 2001; Long and Yaghi, 2009; Zhou et al., 2012; “Joe” Zhou and Kitagawa, 2014; Lu et al., 2014) and satisfy some of the prerequisites of an ideal CO2 capture material: a. high selectivity towards binding CO2, b. high capacity, c. low energy requirement for releasing CO2, d. thermal and chemical stability along with thermal capacity, and e. good synthesizability.
The step following carbon capture is either storage or conversion. Limited known geological storage options along with associated costs of transportation and suffocation risks from potential leaks suggest that conversion and utilization should be strongly committed (Sabri et al., 2021). Conversion achieves the dual objective of converting CO2 to value-added products including urea, methane, methanol, etc., while simultaneously avoiding the risks associated with storage. Conversion of CO2 invariably translates to CO2 reduction. Most thermal CO2 reduction requires harsh conditions involving high temperature and pressures, requires additional energy input and can hinder broad deployment. Depending on the energy source, it could require additional carbon-emitting fossil combustion. This can be circumvented by using CO2 electrochemical reduction (CO2ER) instead of thermal and pressure-driven methods (Kondratenko et al., 2013; Lu et al., 2014; Zhao et al., 2017; Jiao et al., 2019; Wang et al., 2022). Electrochemistry enables production of chemicals that are often more difficult to produce from thermal methods (Gattrell et al., 2006; Kondratenko et al., 2013). While a wide variety of value-added products can be obtained, not all of them are economically feasible. Nitopi et al. weighed the market price of an array of value-added products against the energy required to produce them and concluded that C2+ products such as ethanol, ethylene, and propanol are the most economically feasible (Nitopi et al., 2019). Hence, in this review, we shall focus on processes that exclusively produce C2+ products. Among the catalysts used for CO2ER, Cu-based ones are unparalleled for synthesis of C2+ products (Nitopi et al., 2019).
In this review, we focus on the conversion part of the capture and conversion process because the former is a process driven by thermodynamics and is a very well-studied topic on its own (Eddaoudi et al., 2001; Long and Yaghi, 2009; Zhou et al., 2012; Joe Zhou and Kitagawa, 2014; Lu et al., 2014). Here, we wanted to showcase the impact of electrocatalysis on CO2 reduction within the framework of a conversion material. We explore mechanisms for CO2ER to C2+ products in Cu-based electrodes to develop insights into the process. Such mechanistic knowledge shall guide different strategies that enhance C2+ product formation by optimizing the catalyst and reaction conditions. We then highlight the emerging potential of having a one-pot solution that combines capture and conversion. This has been demonstrated with Cu-based MOF derivatives where the constituent MOFs adsorb CO2 and provide a nice framework for constituent Cu sites to act as catalytic sites for CO2ER. Furthermore, the structure of MOF enhances catalytic activity via synergistic effects in addition to CO2 capture. While some of the mechanistic insights and improvement strategies in Cu electrocatalysis are transferable, we also discuss the additional requirements that are mandated by the one-pot Cu-based MOF solution. Finally, we discuss challenges hindering commercial use of these materials and how they can be overcome, paving the way for using these materials as suitable candidates for broad deployment of CO2 reduction systems.
2 CO2 conversion
Catalysts used to fabricate electrodes are broadly classified into four groups based on the selectivity of their CO2 reduction products: a. formate; b. CO; c. H2; and d. hydrocarbon, aldehydes, and alcohols. The formate producers are metals such as Pb, Hg, Tl, and In, while some metals such as Au, Ag, and Zn primarily produce CO. A few metals including Ni, Fe, Pt, and Ti reduce water to H2 and are termed H2 producing. Cu is the only metal belonging to the fourth category making it ideal for producing C2+ products. This is heavily attributed to the unique characteristic of Cu of having a negative adsorption energy for CO while maintaining a positive one for H species. This translates to the ability to retain and eventually reduce CO while preventing the hydrogen evolution reaction (HER). The adsorption characteristics of CO play an instrumental role because reduction to CO is the first reaction step in CO2ER to C2+ products (Nitopi et al., 2019). CO production is enhanced in the presence of catalysts such as Ag and they have been increasingly used to design bi-metallic catalysts with increased reduction to C2+ products (Nitopi et al., 2019).
While electrodes fabricated from Cu can synthesize a variety of products such as CO, formate, C2H4, and CH4, we are primarily interested in producing C2+ products given their high benefit-to-cost ratio. In specific, formation of the C-C bond in C2+ is the critical first reaction for oligomerization into a range of materials, chemicals, and fuels, enabling CO2 to serve as a primary industrial building block. Many processes exist to maximize the production of C2+ products but from a reduction pathway perspective, they broadly result in these effects: a. increasing the availability of CO and b. enhancing CO dimerization reactions. The first tier of products synthesized after two electron transfer from CO2 in the CO2ER reduction pathway consists of formate and CO (Nitopi et al., 2019). The former is a terminal product, implying that it does not undergo further reduction. Thus, for C2+ production, we need to drive the reduction process to CO, not formate. Increasing the adsorption, transport, and overall availability of CO can enhance formation of C2+ products. This is the principle behind employing metals that selectively produce CO such as Ag, Au, and Zn as a co-catalyst (Nitopi et al., 2019). For example, a study found Cu-Ag bimetallic electrodes resulted in higher production of CH3CHO and C2H4 compared to pure Cu electrode (Ishimaru et al., 2000). The second process is based on a reaction pathway that involves enhancing CO dimerization reactions (Nitopi et al., 2019). Cu (100) is observed to amplify those reactions and is reported to be one of the surfaces with a high selectivity for C2H4 over CH4 (Hori et al., 1995). There are other strategies to boost C2+ products, the most important and widespread one is increasing the electrochemically-active surface area (ECSA). Some methods for increasing ECSA include using Cu nanostructures and stepped Cu surfaces. For example, Manthiram et al. observed fourfold higher current density along with a faradaic efficiency of 80% for CH4 production on Cu nanoparticles with an initial size of 7 nm as compared to polycrystalline Cu foil (Manthiram et al., 2014). These processes are depicted in Figure 1.
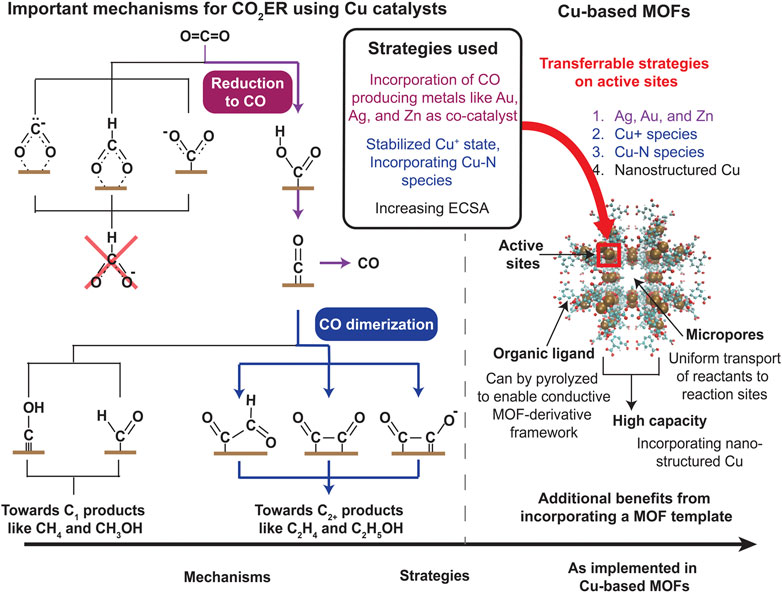
FIGURE 1. A partial CO2ER pathway indicating major reactions process (Hori et al., 1994; 1997; Peterson et al., 2010; Kuhl et al., 2012; Cheng et al., 2015; Kortlever et al., 2015; Montoya et al., 2015; Feaster et al., 2017; Chernyshova et al., 2018; Garza et al., 2018; Lum et al., 2018; Liu et al., 2019; Nitopi et al., 2019). Mechanisms that play a critical role in the production on C2+ products via CO2ER on Cu-based catalysts along with strategies used to enhance them are specifically highlighted. These strategies can be implemented in a Cu-based MOF system; the chemically tunable active sites along with additional advantages of a MOF template for catalysis highlights the ability of Cu-based MOFs to produce C2+ products via CO2ER.
3 Integrated CO2 capture and conversion
Carbon conversion is preceded by carbon capture, but such sequential treatment suffers from the additional cost required for compression and transportation. For transportation, CO2 needs to be compressed up to 150 bar which translates to at least around 360 kJ/kg CO2 of work (Huck et al., 2014). Other challenges with compression include the removal of residual moisture to avoid creating corrosive conditions in the tank and dissipation of heat during the compression process. These costs can be avoided if both processes occur in the same plant location, termed an integrated cascade system (Sabri et al., 2021). The extremely optimistic end of this spectrum is simultaneous capture and conversion. Often, this is achieved by materials that function as both CO2 adsorbers and CO2 reduction catalysts.
Interestingly, metal organic frameworks (MOFs) which belong to the category of solid adsorbers can serve as both an adsorbent for CO2 capture as well as a catalyst for CO2 conversion. Some of the attractive features of MOFs that make it ideal for CO2 capture include a. porous structure, b. chemically tunability, c. compatibility with other materials, d. structural flexibility, and e. hydrophobicity (Ding et al., 2019). The open metal sites and organic linkers serve as Lewis acid and basic sites, respectively, both of which can coordinate and bind CO2 (Ding et al., 2019). For example, M-MOF-74 (M: Mg, Ni, Co., Zn) coordinates CO2 via the metal sites (Kong et al., 2012; Queen et al., 2014) while IRMOF uses its N-containing Lewis base (amines) as the site for binding CO2 (Millward and Yaghi, 2005). Moreover, CO2 capture is enhanced with linkers functionalized with polar functional groups like -F, -Br, -NO2, and -SO3 as the additional dipole interacts with the quadrupole of CO2 (Debatin et al., 2010; Gassensmith et al., 2011; Zhang et al., 2016; Mosca et al., 2018; Ding et al., 2019). Additionally, the chemical features can be altered by easily functionalizing the linkers and modifying metal centers. These sites and modifications enhance CO2 capture via synergistic effects. Lastly, the pore size and flexibility of these hydrophobic structures are tunable (Ding et al., 2019).
Synthesizing C2+ products via electroreduction of CO2 calls for Cu-based electrodes to serve as catalysts. The easily tunable characteristic of MOFs translates to the ability to incorporate Cu within the framework (as shown in Figure 1). Cu-based MOFs such as HKUST-1 exist that exhibit selectivity for C2+ products when used as an electrochemical catalyst (Han et al., 2021a). Table 1 highlights the catalysts, products, and synthesis efficiency. More importantly, we believe that it is important to highlight that not only do Cu-based MOFs combine both capture and conversion capabilities, but the combination increases the performance of both processes.
First, the availability of uniformly dispersed active sites and high surface area already makes MOFs an excellent catalyst template in general while the constituent micropores ensure uniform transport of reactants to the catalytic sites (Ding et al., 2019). MOFs have been used for thermal reduction of CO2 to synthesize organic products via cycloaddition, carboxylation, and cyclization reactions (Zalomaeva et al., 2013; Liu et al., 2015; Zhang et al., 2016; Liang et al., 2017; Xiong et al., 2017; Nguyen et al., 2018; Wang et al., 2018). For example, MMCF-2 has been shown to provide a 95% yield for cycloaddition of CO2 with propylene oxide in the presence of a co-catalyst (Gao et al., 2014).
Second, nanostructured Cu has been shown to display a higher catalytic activity compared to its bulk counterpart owing to a higher ECSA (Nitopi et al., 2019) arising from the structural increase in steps, facets, and edge atoms. Moreover, grain boundaries are found to promote C-C coupling reactions while suppressing HER (Sun et al., 2021). This effect can be readily exploited in MOFs and they provide an opportunity of significantly enhancing ECSA by incorporating Cu nanostructures and single atoms. For example, Cu-based MOFs such as HKUST-1 have been shown to produce C2H4 and C2H5OH at 59% efficiency (Han et al., 2021a). Moreover, a Cu-based HKUST-1 embedded on carbon catalysts via an electrochemical reaction resulted in ∼10-fold increase in faradaic efficiency for synthesizing C2+ products compared to a pristine Cu foil (Wang et al., 2021). This enhancement was attributed to the stepped surfaces, facets, and edges that contributed to the 1.24-fold higher ECSA observed compared to the Cu foil. Cu nanocrystallites were generated in MOF-Cu3(HITP)2 and the rich grain boundaries aided in C-C coupling reactions resulting in producing C2H4 with 60%–70% efficiency (Sun et al., 2021).
Third and most importantly, MOFs provide a structure where additional chemical species can be easily incorporated among the plethora of active sites available. From studies done on Cu electrodes, a variety of additions were found to enhance CO2 conversion that holds true for Cu-based MOFs as well, and are well-suited to be adapted within the MOF structure (Figure 1). One of the primary chemical modifications that enhances C2+ product formation is the addition of metals that produce CO such as Au, Ag, and Zn as co-catalysts. As mentioned in section 1, Au nanoparticles dispersed on a polycrystalline Cu foil results in a 100 x higher reduction rate to C2+ products compared to Cu alone (Morales-Guio et al., 2018). This effect is also observed in CuZn bimetallic material embedded in a carbonized MOF, where the influence of Zn led to a 5-fold increase in faradaic efficiency in producing C2H4 and C2H5OH (Juntrapirom et al., 2021). A similar effect was also seen in Ag/Cu bimetallic catalysts based on a Cu-based MOF derivative where many C2+ products were produced with an efficiency of 21% (Sikdar et al., 2022).
MOFs can also incorporate non-metals within its framework. Appending N-containing Cu-N species is credited to amplify formation of C2+ products and lend higher stability. The former is attributed to the more stable adsorption of the CH2 intermediate species and inhibition of HER (Jin et al., 2021). This is cited as the reason for the Cu2O/Cu@NC catalyst made from Cu-NBDC MOF showing 24% efficiency in forming C2H4 in direct contrast to the Cu2O/Cu@C catalyst (no N content) which did not produce any C2H4 (Jin et al., 2021). N, N dimethylformamide (DMF) was used in Cu-BTC and it was found to increase dimerization reactions producing oxalic acid with an efficiency of 51% (Senthil Kumar, Senthil Kumar and Anbu Kulandainathan, 2012). Similarly, N-containing ligands in Cu (II)/ade-MOFs augmented C2H4 generation with a 45% efficiency (Yang et al., 2019). An N-containing BEN-Cu-BTC derivative displayed efficiencies of 18% and 11% for C2H5OH and C2H4, respectively (Cheng et al., 2019). As seen from section 2, adding N-containing species is also a strategy used to increase CO2 capture as these groups interact with CO2 to enhance adsorption making this a dual-purpose addition for both capture and conversion.
Furthermore, stabilizing the Cu+ state was shown to boost synthesis of C2+ products via enhancing CO dimerization. This effect was observed in HKUST-1 where the high Cu+/Cu0 ratio resulted in producing C2H4 and C2H5OH with efficiency of 59% (Han et al., 2021a). For similar reasons, the Cu@CuxO structure in a Cu-based MOF derivative created C2H4 with 51% efficiency (Yao et al., 2020).
While MOFs contain a plethora of advantages for CO2ER, they are also plagued by some disadvantages. Some of them are the lack of conductivity and stability. This necessitates the addition of conductive supports that can in turn, complicate manufacturability. To truly enhance the catalytic activity to the maximum limit, we need to explore methods of making the MOF conductive on its own. Fortuitously, this can be achieved by pyrolyzing the MOFs to create MOF derivatives that not only are stable and conducting because of the carbonization of the organic linkers at high temperatures, but the process also creates additional active sites (Chen et al., 2018). These reasons led to oxide-derived Cu/carbon catalyst generated from HKUST-1 via pyrolysis displaying a very low overpotential (−0.1 V vs. RHE) for production of C2H5OH (Zhao et al., 2017). Other such Cu-based MOF derivatives are listed in Table 1 with the products and their efficiencies. These examples demonstrate that Cu-based MOF derivatives are well suited for CO2ER to C2+ products.
4 Conclusion
An integrated carbon capture and conversion process is urgently needed to address atmospheric GHG concentrations. A one-pot solution avoids the additional cost, energy burden, and risks of compression and transportation that is associated with the CO2 storage (and succeeding conversion). Only conversion to C2+ products is reported to be economically feasible. Since electrochemical reduction can be achieved under milder temperature and pressure conditions, they are preferred over thermal reduction. Cu-based catalysts are the gold standard for CO2ER to synthesize C2+ products while MOFs are renowned for their CO2 capture abilities. The chemical tunability of MOFs makes it possible to make Cu-based MOFs which fit perfectly with the requirement of the one-pot solution to enable CO2 capture and CO2ER synthesis of C2+ products. A series of Cu-based MOFs were explored and their performance in converting CO2 to C2+ products were summarized (Table 1). We also delved deeper into the mechanisms that are involved in C2+ product formation and various strategies that can be used to enhance it. Notable among these processes are incorporating Ag/Au/Zn as co-catalysts to increase CO yield, as converting CO2 to CO is the first step in the CO2ER pathway. Another strategy is increasing the ECSA of the catalyst, which is the principle behind using nanostructured Cu instead of polycrystalline Cu foil. Moreover, these Cu nanoclusters can be embedded into MOFs as well. We also explored one critical disadvantage of MOFs for electrocatalysis: their low electrical conductivity, which necessitates using conductive supports. However, using pyrolyzed MOFs is an easy way to achieve the requisite electrical conductivity while also boosting stability. These materials classes are highlighted in Figure 2. We believe that this one-pot solution can be used for cleaned and reacted flue gas with the added advantage of avoiding energies involved in pressurization and collection. This has been reported in a few studies (D’Alessandro et al., 2010; Kong et al., 2012; McDonald et al., 2012). Moreover, we want to highlight that this technology is in its conceptual stage and is not a working technology yet. Transformation to a full-fledged technology would require detailed evaluation of the scientific and engineering challenges such as separation of the reduction product from electrolyte and optimizing extended operation. A technoeconomic analysis would be useful to determine the scalability and to direct the implementation towards viable pathways.
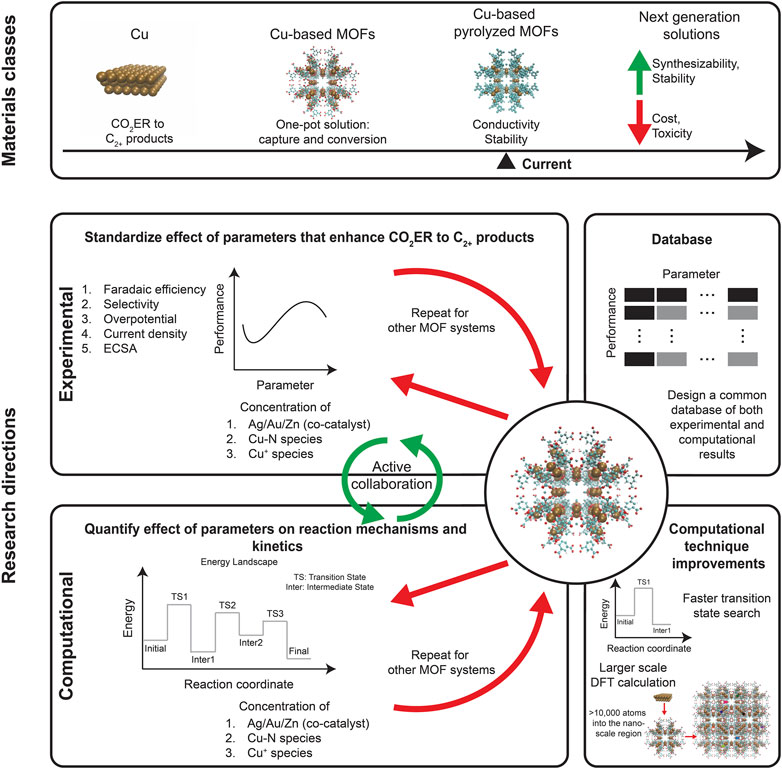
FIGURE 2The materials classes involved in the CO2ER to C2+ products: each successive progression highlights an improvement over the prior group. Future research directions are depicted.
While Cu-based MOFs display a high potential for CO2ER, translating it to commercial use requires us to overcome challenges associated with the scaling-up of fabrication and deployment of MOFs. Firstly, MOFs are expensive and often, the primary contributing factor is the solvent cost (DeSantis et al., 2017). For example, it accounts for 40% of the cost in HKUST-1 made via solvothermal process (DeSantis et al., 2017). Opting for alternative processes such as liquid assisted grinding (LAG) and aqueous synthesis has the potential to reduce the cost by 34%–83% as these processes use less solvent in comparison (DeSantis et al., 2017). Moreover, the presence of inorganic nano-sized metal ions that are non-biodegradable along with trapped non-aqueous solvents such as dimethylformamide (DMF) make MOFs toxic (Kumar et al., 2019). More detailed studies on the toxic effects of MOFs are required for design of less toxic MOFs. Additionally, operation of MOFs for electrocatalytic applications often subject them to exposure to harsh chemical environments making chemical stability essential for efficient operation. Stability, in turn, is a direct result of the bonding environment including interactions such as hydrogen bonding and pi stacking (Coe, 2002; Nouar et al., 2008; Howarth et al., 2016). Incorporating a more inert central metal ion in the MOF structure can increase chemical stability (Kang et al., 2011; Leus et al., 2016). MOF derivatives synthesized via pyrolysis also slow down metal nanoparticle corrosion in the electrolyte, which in turn improves stability (Xiao et al., 2022). Another strategy to consider would be wrapping a protecting film on the surface of MOFs and MOF derivatives (Xiao et al., 2022). Mechanical stability is another factor affecting operation and while strategies like interweaving MOF networks (Tan and Cheetham, 2011; Burtch et al., 2014; Katz et al., 2015) are often used, this can be redundant in MOFs for CO2ER as liquid electrolyte filling MOFs enhances its stability already (Bennett et al., 2015; van de Voorde et al., 2015). Thermal stability is not as important in MOFs for CO2ER as electroreduction does not require harsh thermal conditions for CO2 capture or conversion. Finally, the poor electrical conductivity of the organic linkers necessitates presence of conductive substrates such as carbon cloth (Xiao et al., 2022). As previously highlighted, MOF derivatives created via pyrolysis can provide the required conductivity with the formed carbon layers. By altering synthesis parameters, the degree of graphitization can be enhanced. By adjusting the chemistry of the MOF precursors, the degree of defect carbon structure can be tuned. Both strategies can be used to optimize the resulting MOF derivative for enhanced conductivity (Xiao et al., 2022).
Lastly, we summarize our review with Figure 2 and we provide conjectures regarding research needs for the next-generation one-pot carbon-neutral solutions. Future research should standardize experimental results to facilitate comparison across multiple studies, enabling the community to accelerate the discovery process (Ward et al., 2022). Most of the electrochemical reductions occur at the interface of the electrode and the electrolyte. Using experimental characterization techniques can help us understand bonding and identify species participating in the reaction. Overall, investigating the solid/liquid interface using characterization techniques requires overcoming two major challenges. First, characterization techniques need to be adapted for high pressure environments, as opposed to high vacuum, to replicate the more realistic experimental conditions. Second, the region corresponding to the electrical double layer is small and sandwiched between the bulk electrode and the bulk electrolyte, requiring for Å to nm resolution. Thus, the characterization techniques need to be specifically modified to enable a focus on the solid/liquid interface. Recent developments in characterization techniques like Fourier Transform Infrared spectroscopy (FTIR), Raman spectroscopy, and Ambient-Pressure X-Ray Spectroscopy (APXPS) have been able to overcome these challenges (Tian & Ren, 2004; Zaera, 2014; Favaro et al., 2017b; Salmeron, 2018; Lu et al., 2019; Han et al., 2021b; Radjenovic et al., 2021; Ye & Liu, 2021; Hao et al., 2022). Using these characterization tools could enable a deeper understanding of the mechanisms involved in the activation process (Favaro et al., 2017a; Qian et al., 2019; Qian et al., 2020). The field will benefit from active collaboration across experimental and computational investigations with the former focused on evaluating the performance of Cu-based MOFs as a function of parameters involved in the mechanism enhancement. On the other hand, computational studies can play a key role in assessing the energy landscape involved in the conversion of CO2 to specific products. These assessments can be accelerated by implementing larger scale density functional theory (DFT) calculations (Kronik et al., 2006; Zhou et al., 2006; Xu et al., 2019; 2021; Motamarri et al., 2020; Dogan et al., 2022; Hao et al., 2022; Xu et al., 2022) along with a faster transition state search procedure (Koistinen et al., 2017; Garrido Torres et al., 2019; Meyer et al., 2019). Eventually, a database common to both experimental and computational work, as outlined by the Materials Project (Jain et al., 2013) can be constructed which can go a long way towards helping design high performance solutions.
Author contributions
AJ and JQ contributed to the conception of the review. AJ wrote the first draft of the manuscript. All authors contributed to manuscript revision.
Funding
JQ was supported by the Laboratory Directed Research and Development Program of Lawrence Berkeley National Laboratory through Contract No. DE-AC02-05CH11231. AJ and EC. were supported by an Early Career Award in the Condensed Phase and Interfacial Molecular Science Program, in the Chemical Sciences Geosciences and Biosciences Division of the Office of Basic Energy Sciences of the United States. Department of Energy under Contract No. DE-AC02-05CH11231. SWS was supported by Idaho National Laboratory under the United States Department of Energy through contract DE-AC07-05ID14517.
Acknowledgments
The authors are grateful to Yingchao Yang for his discussions and feedback.
Conflict of interest
The authors declare that the research was conducted in the absence of any commercial or financial relationships that could be construed as a potential conflict of interest.
Publisher’s note
All claims expressed in this article are solely those of the authors and do not necessarily represent those of their affiliated organizations, or those of the publisher, the editors and the reviewers. Any product that may be evaluated in this article, or claim that may be made by its manufacturer, is not guaranteed or endorsed by the publisher.
Abbreviations
DOBDC; 2,5-Dihydroxyterephthalate, BTC; Benzene-1,3,5-tricarboxylate, BDC; 1,4-Benzenedicarboxylate, BPDC; Biphenyl-1,4-dicarboxylate, M-MOF-74; [M2(DOBDC) (H2O)2] (M = Mg2+, Ni2+, Co2+, Zn2+), IRMOF-3; [Zn4O(NH2BDC)3], HKUST-1; [Cu3(BTC)2(H2O)3], HITP; 2,3,6,7,10,11-hexaiminotriphenylene, H-CuTCPP; Helical Cu porphyrinic MOF meso-tetra (4- carboxyphenyl) porphyrin, CuAdeAce; Copper(II)–adeninate–acetate, CuDTA MOA; Copper bis-bidentate dithiooxamidate metal-organic aerogel, Cu(II)/ade-MOF; CuII/adeninato/carboxylato metal-biomolecule frameworks, Cu MOF-NC; BEN-Cu BTC; Calcination of N-containing benzimidazole-modified Cu-BTC MOFs (BEN-Cu-BTC), Cu-NBDC; Cu 2-aminoterephthalic acid, Cu-GNC-VL; Cu/Cu2O nanocomposite loaded on the surface of carbon derived from direct carbonization of two-dimensional cross-like zeolitic imidazolate framework-L coated vertically on graphene oxide, H4TACTMB; 1,4,7,10-tetrazazcyclododecane-N,N′,N″,N‴-tetra-p-methylbenzoic acid, MMCF-2; [Cu2(Cu-TACTMB) (H2O)3(NO3)2], F-IRMOF-3; Functionalized IRMOF; NH2 groups converted to quaternary ammonium salt though introduction of CH3I.
References
Albo, J., Vallejo, D., Beobide, G., Castillo, O., Castaño, P., Irabien, A., et al. (2017). Copper-based metal–organic porous materials for CO2 electrocatalytic reduction to alcohols. ChemSusChem 10 (6), 1100–1109. doi:10.1002/cssc.201600693
An, B., Li, Z., Song, Y., Zhang, J., Zeng, L., Wang, C., et al. (2019). Cooperative copper centres in a metal–organic framework for selective conversion of CO2 to ethanol. Nat. Catal. 2 (8), 709–717. doi:10.1038/s41929-019-0308-5
Bennett, T. D., Sotelo, J., Tan, J. C., and Moggach, S. A. (2015). Mechanical properties of zeolitic metal–organic frameworks: Mechanically flexible topologies and stabilization against structural collapse. CrystEngComm 17 (2), 286–289. doi:10.1039/C4CE02145B
Burtch, N. C., Jasuja, H., and Walton, K. S. (2014). Water stability and adsorption in metal-organic frameworks. Chem. Rev. 114 (20), 10575–10612. doi:10.1021/cr5002589
Chen, Y.-Z., Zhang, R., Jiao, L., and Jiang, H. L. (2018). Metal–organic framework-derived porous materials for catalysis. Coord. Chem. Rev. 362, 1–23. doi:10.1016/j.ccr.2018.02.008
Cheng, T., Xiao, H., and Goddard, W. A. I. I. I. (2015). Free-energy barriers and reaction mechanisms for the electrochemical reduction of CO on the Cu(100) surface, including multiple layers of explicit solvent at pH 0. J. Phys. Chem. Lett. 6 (23), 4767–4773. doi:10.1021/acs.jpclett.5b02247
Cheng, Y.-S., Chu, X. P., Ling, M., Li, N., Wu, K. L., Wu, F. H., et al. (2019). An MOF-derived copper@nitrogen-doped carbon composite: The synergistic effects of N-types and copper on selective CO 2 electroreduction. Catal. Sci. Technol. 9 (20), 5668–5675. doi:10.1039/C9CY01131E
Chernyshova, I., Somasundaran, P., and Ponnurangam, S. (2018). On the origin of the elusive first intermediate of CO2 electroreduction. Proc. Natl. Acad. Sci. 115 (40), E9261–E9270. doi:10.1073/pnas.1802256115
Coe, C. G. (2002). “Structural effects on the adsorptive properties of molecular sieves for air separation,” in Access in nanoporous materials. Editors T. J. Pinnavaia, and M. F. Thorpe (Boston, MA: Springer US (Fundamental Materials Research), 213–229. doi:10.1007/0-306-47066-7_14
D'Alessandro, D. M., Smit, B., and Long, J. R. (2010). Carbon dioxide capture: Prospects for new materials. Angew. Chem. Int. Ed. 49 (35), 6058–6082. doi:10.1002/anie.201000431
Debatin, F., Thomas, A., Kelling, A., Hedin, N., Bacsik, Z., Senkovska, I., et al. (2010). In situ synthesis of an imidazolate-4-amide-5-imidate ligand and formation of a microporous zinc–organic framework with H2-and CO2-storage ability. Angew. Chem. 122 (7), 1280–1284. doi:10.1002/ange.200906188
DeSantis, D., Mason, J. A., James, B. D., Houchins, C., Long, J. R., and Veenstra, M. (2017). Techno-economic analysis of metal–organic frameworks for hydrogen and natural gas storage. Energy and Fuels 31 (2), 2024–2032. doi:10.1021/acs.energyfuels.6b02510
Ding, M., Flaig, R. W., Jiang, H. L., and Yaghi, O. M. (2019). Carbon capture and conversion using metal–organic frameworks and MOF-based materials. Chem. Soc. Rev. 48 (10), 2783–2828. doi:10.1039/C8CS00829A
Dogan, M., Liou, K.-H., and Chelikowsky, J. R. (2022). Real-space methods for electronic structure calculations of over 100,000 atoms. United States: TACC. doi:10.26153/TSW/43732
Eddaoudi, M., Moler, D. B., Li, H., Chen, B., Reineke, T. M., O'Keeffe, M., et al. (2001). Modular chemistry: Secondary building units as a basis for the design of highly porous and robust Metal−Organic carboxylate frameworks. Accounts Chem. Res. 34 (4), 319–330. doi:10.1021/ar000034b
Favaro, M., Liu, Z., and Crumlin, E. J. (2017b). Ambient-pressure X-ray photoelectron spectroscopy to characterize the solid/liquid interface: Probing the electrochemical double layer. Synchrotron Radiat. News 30 (2), 38–40. doi:10.1080/08940886.2017.1289806
Favaro, M., Xiao, H., Cheng, T., Goddard, W. A., Yano, J., and Crumlin, E. J. (2017a). Subsurface oxide plays a critical role in CO2 activation by Cu (111) surfaces to form chemisorbed CO2, the first step in reduction of CO2. Proc. Natl. Acad. Sci. 114 (26), 6706–6711. doi:10.1073/pnas.1701405114
Feaster, J. T., Shi, C., Cave, E. R., Hatsukade, T., Abram, D. N., Kuhl, K. P., et al. (2017). Understanding selectivity for the electrochemical reduction of carbon dioxide to formic acid and carbon monoxide on metal electrodes. ACS Catal. 7 (7), 4822–4827. doi:10.1021/acscatal.7b00687
Field, C. B., and Barros, V. R. (2014). Climate change 2014: Impacts, adaptation, and vulnerability: Working group II contribution to the fifth assessment report of the intergovernmental panel on climate change. New York, NY: Cambridge University Press.
Gao, W.-Y., Chen, Y., Niu, Y., Williams, K., Cash, L., Perez, P. J., et al. (2014). Crystal engineering of an nbo topology metal–organic framework for chemical fixation of CO2 under ambient conditions. Angew. Chem. Int. Ed. 53 (10), 2615–2619. doi:10.1002/anie.201309778
Garrido Torres, J. A., Jennings, P. C., Hansen, M. H., Boes, J. R., and Bligaard, T. (2019). Low-scaling algorithm for nudged elastic band calculations using a surrogate machine learning model. Phys. Rev. Lett. 122 (15), 156001. doi:10.1103/physrevlett.122.156001
Garza, A. J., Bell, A. T., and Head-Gordon, M. (2018). Mechanism of CO2 reduction at copper surfaces: Pathways to C2 products. ACS Catal. 8 (2), 1490–1499. doi:10.1021/acscatal.7b03477
Gassensmith, J. J., Furukawa, H., Smaldone, R. A., Forgan, R. S., Botros, Y. Y., Yaghi, O. M., et al. (2011). Strong and reversible binding of carbon dioxide in a green metal–organic framework. J. Am. Chem. Soc. 133 (39), 15312–15315. doi:10.1021/ja206525x
Gattrell, M., Gupta, N., and Co, A. (2006). A review of the aqueous electrochemical reduction of CO2 to hydrocarbons at copper. J. Electroanal. Chem. 594 (1), 1–19. doi:10.1016/j.jelechem.2006.05.013
Gielen, D., Boshell, F., Saygin, D., Bazilian, M. D., Wagner, N., and Gorini, R. (2019). The role of renewable energy in the global energy transformation. Energy Strategy Rev. 24, 38–50. doi:10.1016/j.esr.2019.01.006
Han, Y., Zhang, H., Yu, Y., and Liu, Z. (2021b). In situ characterization of catalysis and electrocatalysis using APXPS. ACS Catal. 11 (3), 1464–1484. doi:10.1021/acscatal.0c04251
Han, Y., Zhu, S., Xu, S., Niu, X., Xu, Z., Zhao, R., et al. (2021a). Understanding structure-activity relationship on metal-organic-framework-derived catalyst for CO2 electroreduction to C2 products. ChemElectroChem 8 (16), 3174–3180. doi:10.1002/celc.202100942
Hao, H., Pestana, L. R., Qian, J., Liu, M., Xu, Q., Head-Gordon, T., et al. (2022). Chemical transformations and transport phenomena at interfaces. New Jersey, United States: Wiley Interdisciplinary Reviews: Computational Molecular Science, e1639. doi:10.1002/WCMS.1639
Hori, Y. (2008). “Electrochemical CO2 reduction on metal electrodes,” in Modern aspects of Electrochemistry. Editors C. G. Vayenas, R. E. White, and M. E. Gamboa-Aldeco (New York, NY: Springer), 89–189. (Modern Aspects of Electrochemistry). doi:10.1007/978-0-387-49489-0_3
Hori, Y., Takahashi, R., Yoshinami, Y., and Murata, A. (1997). Electrochemical reduction of CO at a copper electrode. J. Phys. Chem. B 101 (36), 7075–7081. doi:10.1021/jp970284i
Hori, Y., Wakebe, H., Tsukamoto, T., and Koga, O. (1995). Adsorption of CO accompanied with simultaneous charge transfer on copper single crystal electrodes related with electrochemical reduction of CO2 to hydrocarbons. Surf. Sci. 335, 258–263. doi:10.1016/0039-6028(95)00441-6
Hori, Y., Wakebe, H., Tsukamoto, T., and Koga, O. (1994). Electrocatalytic process of CO selectivity in electrochemical reduction of CO2 at metal electrodes in aqueous media. Electrochimica Acta 39 (11), 1833–1839. doi:10.1016/0013-4686(94)85172-7
Howarth, A. J., Liu, Y., Li, P., Li, Z., Wang, T. C., Hupp, J. T., et al. (2016). Chemical, thermal and mechanical stabilities of metal-organic frameworks. Nat. Rev. Mater. 1, 15018. doi:10.1038/natrevmats.2015.18
Huck, J. M., Lin, L. C., Berger, A. H., Shahrak, M. N., Martin, R. L., Bhown, A. S., et al. (2014). Evaluating different classes of porous materials for carbon capture. Energy and Environ. Sci. 7 (12), 4132–4146. doi:10.1039/C4EE02636E
Ishimaru, S., Shiratsuchi, R., and Nogami, G. (2000). Pulsed electroreduction of CO[sub 2] on Cu-Ag alloy electrodes. J. Electrochem. Soc. 147 (5), 1864. doi:10.1149/1.1393448
Iyer, S. S., Bajaj, I., Balasubramanian, P., and Hasan, M. M. F. (2017). Integrated carbon capture and conversion to produce syngas: Novel process design, intensification, and optimization. Industrial Eng. Chem. Res. 56 (30), 8622–8648. doi:10.1021/acs.iecr.7b01688
Jain, A., Ong, S. P., Hautier, G., Chen, W., Richards, W. D., Dacek, S., et al. (2013). Commentary: The materials Project: A materials genome approach to accelerating materials innovation. Apl. Mater. 1 (1), 011002. doi:10.1063/1.4812323
Jiao, L., Xie, F., Chen, R., Ye, D., Zhang, B., An, L., et al. (2019). Toward CO2 utilization for direct power generation using an integrated system consisting of CO2 photoreduction with 3D TiO2/Ni-foam and a photocatalytic fuel cell. J. Mater. Chem. A 7 (11), 6275–6284. doi:10.1039/C8TA12255E
Jin, H., Xiong, L., Zhang, X., Lian, Y., Chen, S., Lu, Y., et al. (2021). Cu-Based catalyst derived from nitrogen-containing metal organic frameworks for electroreduction of CO<sub>2</sub>. Wuli Huaxue Xuebao/Acta Phys. - Chim. Sin. 37 (11), 2006017. doi:10.3866/PKU.WHXB202006017
Joe Zhou, H.-C., and Kitagawa, S. (2014). Metal–organic frameworks (MOFs). Chem. Soc. Rev. 43 (16), 5415–5418. doi:10.1039/C4CS90059F
Juntrapirom, S., Santatiwongchai, J., Watwiangkham, A., Suthirakun, S., Butburee, T., Faungnawakij, K., et al. (2021). Tuning CuZn interfaces in metal–organic framework-derived electrocatalysts for enhancement of CO2 conversion to C2 products. Catal. Sci. Technol. 11 (24), 8065–8078. doi:10.1039/D1CY01839F
Kang, I. J., Khan, N. A., Haque, E., and Jhung, S. H. (2011). Chemical and thermal stability of isotypic metal–organic frameworks: Effect of metal ions. Chem. – A Eur. J. 17 (23), 6437–6442. doi:10.1002/chem.201100316
Katz, M. J., Moon, S. Y., Mondloch, J. E., Beyzavi, M. H., Stephenson, C. J., Hupp, J. T., et al. (2015). Exploiting parameter space in MOFs: A 20-fold enhancement of phosphate-ester hydrolysis with UiO-66-NH2. Chem. Sci. 6 (4), 2286–2291. doi:10.1039/c4sc03613a
Koistinen, O. P., Dagbjartsdottir, F. B., Asgeirsson, V., Vehtari, A., and Jonsson, H. (2017). Nudged elastic band calculations accelerated with Gaussian process regression. J. Chem. Phys. 147 (15), 152720. doi:10.1063/1.4986787
Kondratenko, E., Mul, G., Baltrusaitis, J., Larrazabal, G. O., and Perez-Ramirez, J. (2013). Status and perspectives of CO2 conversion into fuels and chemicals by catalytic, photocatalytic and electrocatalytic processes. Energy and Environ. Sci. 6 (11), 3112–3135. doi:10.1039/C3EE41272E
Kong, X., Scott, E., Ding, W., Mason, J. A., Long, J. R., and Reimer, J. A. (2012). CO2 dynamics in a metal–organic framework with open metal sites. J. Am. Chem. Soc. 134 (35), 14341–14344. doi:10.1021/ja306822p
Kortlever, R., Shen, J., Schouten, K. J. P., Calle-Vallejo, F., and Koper, M. T. M. (2015). Catalysts and reaction pathways for the electrochemical reduction of carbon dioxide. J. Phys. Chem. Lett. 6 (20), 4073–4082. doi:10.1021/acs.jpclett.5b01559
Kronik, L., Makmal, A., Tiago, M. L., Alemany, M. M. G., Jain, M., Huang, X., et al. (2006). Parsec – the pseudopotential algorithm for real-space electronic structure calculations: Recent advances and novel applications to nano-structures. Phys. status solidi (b) 243 (5), 1063–1079. doi:10.1002/PSSB.200541463
Kuhl, K. P., Cave, E. R., Abram, D. N., and Jaramillo, T. F. (2012). New insights into the electrochemical reduction of carbon dioxide on metallic copper surfaces. Energy and Environ. Sci. 5 (5), 7050–7059. doi:10.1039/C2EE21234J
Kumar, P., Anand, B., Tsang, Y. F., Kim, K. H., Khullar, S., and Wang, B. (2019). Regeneration, degradation, and toxicity effect of MOFs: Opportunities and challenges. Environ. Res. 176, 108488. doi:10.1016/j.envres.2019.05.019
Leus, K., Bogaerts, T., De Decker, J., Depauw, H., Hendrickx, K., Vrielinck, H., et al. (2016). Systematic study of the chemical and hydrothermal stability of selected “stable” Metal Organic Frameworks. Microporous Mesoporous Mater. 226, 110–116. doi:10.1016/j.micromeso.2015.11.055
Liang, L., Liu, C., Jiang, F., Chen, Q., Zhang, L., Xue, H., et al. (2017). Carbon dioxide capture and conversion by an acid-base resistant metal-organic framework. Nat. Commun. 8 (1), 1233. doi:10.1038/s41467-017-01166-3
Liu, X.-H., Ma, J. G., Niu, Z., Yang, G. M., and Cheng, P. (2015). An efficient nanoscale heterogeneous catalyst for the capture and conversion of carbon dioxide at ambient pressure. Angew. Chem. Int. Ed. 54 (3), 988–991. doi:10.1002/anie.201409103
Liu, X., Schlexer, P., Xiao, J., Ji, Y., Wang, L., Sandberg, R. B., et al. (2019). pH effects on the electrochemical reduction of CO(2) towards C2 products on stepped copper. Nat. Commun. 10 (1), 32. doi:10.1038/s41467-018-07970-9
Long, J. R., and Yaghi, O. M. (2009). The pervasive chemistry of metal–organic frameworks. Chem. Soc. Rev. 38 (5), 1213–1214. doi:10.1039/B903811F
Lu, W., Wei, Z., Gu, Z. Y., Liu, T. F., Park, J., Park, J., et al. (2014). Tuning the structure and function of metal–organic frameworks via linker design. Chem. Soc. Rev. 43 (16), 5561–5593. doi:10.1039/C4CS00003J
Lu, Y. H., Larson, J. M., Baskin, A., Zhao, X., Ashby, P. D., Prendergast, D., et al. (2019). Infrared nanospectroscopy at the graphene–electrolyte interface. Nano Lett. 19 (8), 5388–5393. doi:10.1021/acs.nanolett.9b01897
Lum, Y., Cheng, T., Goddard, W. A., and Ager, J. W. (2018). Electrochemical CO reduction builds solvent water into oxygenate products. J. Am. Chem. Soc. 140 (30), 9337–9340. doi:10.1021/jacs.8b03986
Manthiram, K., Beberwyck, B. J., and Alivisatos, A. P. (2014). Enhanced electrochemical methanation of carbon dioxide with a dispersible nanoscale copper catalyst. J. Am. Chem. Soc. 136 (38), 13319–13325. doi:10.1021/ja5065284
McDonald, T. M., Lee, W. R., Mason, J. A., Wiers, B. M., Hong, C. S., and Long, J. R. (2012). Capture of carbon dioxide from air and flue gas in the alkylamine-appended metal–organic framework mmen-Mg2 (dobpdc). J. Am. Chem. Soc. 134 (16), 7056–7065. doi:10.1021/ja300034j
Metz, B. (2007). Climate change 2007: Mitigation of climate change: Contribution of working group III to the fourth assessment report of the intergovernmental panel on climate change. Cambridge ; New York: Cambridge University Press.
Meyer, R., Schmuck, K. S., and Hauser, A. W. (2019). Machine learning in computational chemistry: An evaluation of method performance for nudged elastic band calculations. J. Chem. Theory Comput. 15, 6513–6523. doi:10.1021/acs.jctc.9b00708
Millward, A. R., and Yaghi, O. M. (2005). Metal−Organic frameworks with exceptionally high capacity for storage of carbon dioxide at room temperature. J. Am. Chem. Soc. 127 (51), 17998–17999. doi:10.1021/ja0570032
Montoya, J. H., Shi, C., Chan, K., and Norskov, J. K. (2015). Theoretical insights into a CO dimerization mechanism in CO2 electroreduction. J. Phys. Chem. Lett. 6 (11), 2032–2037. doi:10.1021/acs.jpclett.5b00722
Morales-Guio, C. G., Cave, E. R., Nitopi, S. A., Feaster, J. T., Wang, L., Kuhl, K. P., et al. (2018). Improved CO2 reduction activity towards C2+ alcohols on a tandem gold on copper electrocatalyst. Nat. Catal. 1 (10), 764–771. doi:10.1038/s41929-018-0139-9
Mosca, N., Vismara, R., Fernandes, J. A., Tuci, G., Di Nicola, C., Domasevitch, K. V., et al. (2018). Nitro-functionalized bis(pyrazolate) metal–organic frameworks as carbon dioxide capture materials under ambient conditions. Chem. – A Eur. J. 24 (50), 13170–13180. doi:10.1002/chem.201802240
Motamarri, P., Das, S., Rudraraju, S., Ghosh, K., Davydov, D., and Gavini, V. (2020). DFT-FE – a massively parallel adaptive finite-element code for large-scale density functional theory calculations. Comput. Phys. Commun. 246, 106853. doi:10.1016/J.CPC.2019.07.016
Nam, D.-H., Bushuyev, O. S., Li, J., De Luna, P., Seifitokaldani, A., Dinh, C. T., et al. (2018). Metal–organic frameworks mediate Cu coordination for selective CO2 electroreduction. J. Am. Chem. Soc. 140 (36), 11378–11386. doi:10.1021/jacs.8b06407
Nguyen, P. T. K., Nguyen, H. T. D., Nguyen, H. N., Trickett, C. A., Ton, Q. T., Gutierrez-Puebla, E., et al. (2018). New metal–organic frameworks for chemical fixation of CO2. ACS Appl. Mater. Interfaces 10 (1), 733–744. doi:10.1021/acsami.7b16163
Nitopi, S., Bertheussen, E., Scott, S. B., Liu, X., Engstfeld, A. K., Horch, S., et al. (2019). Progress and perspectives of electrochemical CO2 reduction on copper in aqueous electrolyte. Chem. Rev. 119 (12), 7610–7672. doi:10.1021/acs.chemrev.8b00705
Nouar, F., Eubank, J. F., Bousquet, T., Wojtas, L., Zaworotko, M. J., and Eddaoudi, M. (2008). Supermolecular building blocks (SBBs) for the design and synthesis of highly porous metal-organic frameworks. J. Am. Chem. Soc. 130 (6), 1833–1835. doi:10.1021/ja710123s
OECD (2012). OECD environmental outlook to 2050: The consequences of inaction. Paris: Organisation for Economic Co-operation and Development.
Paul, A., and Michelle, B. (2020). Basic research needs for carbon capture: Beyond 2020: Report of the basic energy Sciences Workshop for carbon capture: Beyond 2020. United States: OSTI.GOV, 196.
Peterson, A. A., Abild-Pedersen, F., Studt, F., Rossmeisl, J., and Norskov, J. K. (2010). How copper catalyzes the electroreduction of carbon dioxide into hydrocarbon fuels. Energy and Environ. Sci. 3 (9), 1311. doi:10.1039/c0ee00071j
Qian, J., Baskin, A., Liu, Z., Prendergast, D., and Crumlin, E. J. (2020). Addressing the sensitivity of signals from solid/liquid ambient pressure XPS (APXPS) measurement. J. Chem. Phys. 153 (4), 044709. doi:10.1063/5.0006242
Qian, J., Ye, Y., Yang, H., Yano, J., Crumlin, E. J., and Goddard, W. A. (2019). Initial steps in forming the electrode–electrolyte interface: H2O adsorption and complex formation on the Ag (111) surface from combining quantum mechanics calculations and ambient pressure X-ray photoelectron spectroscopy. J. Am. Chem. Soc. 141 (17), 6946–6954. doi:10.1021/jacs.8b13672
Queen, W., Hudson, M. R., Bloch, E. D., Mason, J. A., Gonzalez, M. I., Lee, J. S., et al. (2014). Comprehensive study of carbon dioxide adsorption in the metal–organic frameworks M 2 (dobdc) (M = Mg, Mn, Fe, Co, Ni, Cu, Zn). Chem. Sci. 5 (12), 4569–4581. doi:10.1039/C4SC02064B
Radjenovic, P. M., Zhou, R. Y., Dong, J. C., and Li, J. F. (2021). Watching reactions at solid–liquid interfaces with in situ Raman spectroscopy. J. Phys. Chem. C 125 (48), 26285–26295. doi:10.1021/acsjpcc.1c08640
Rafiee, A., Rajab Khalilpour, K., Milani, D., and Panahi, M. (2018). Trends in CO2 conversion and utilization: A review from process systems perspective. J. Environ. Chem. Eng. 6 (5), 5771–5794. doi:10.1016/j.jece.2018.08.065
Sabri, M. A., Al Jitan, S., Bahamon, D., Vega, L. F., and Palmisano, G. (2021). Current and future perspectives on catalytic-based integrated carbon capture and utilization. Sci. Total Environ. 790, 148081. doi:10.1016/j.scitotenv.2021.148081
Salmeron, M. (2018). From surfaces to interfaces: Ambient pressure XPS and beyond. Top. Catal. 61 (20), 2044–2051. doi:10.1007/s11244-018-1069-0
Senthil Kumar, R., Senthil Kumar, S., and Anbu Kulandainathan, M. (2012). Highly selective electrochemical reduction of carbon dioxide using Cu based metal organic framework as an electrocatalyst. Electrochem. Commun. 25, 70–73. doi:10.1016/j.elecom.2012.09.018
Sikdar, N., Junqueira, J. R. C., Dieckhofer, S., Quast, T., Braun, M., Song, Y., et al. (2021). A metal–organic framework derived cux O y C z catalyst for electrochemical CO 2 reduction and impact of local pH change. Angew. Chem. Int. Ed. 60 (43), 23427–23434. doi:10.1002/anie.202108313
Sikdar, N., Junqueira, J. R. C., Ohl, D., Dieckhofer, S., Quast, T., Braun, M., et al. (2022). Redox replacement of silver on MOF-derived Cu/C nanoparticles on gas diffusion electrodes for electrocatalytic CO2 reduction. Chem. - A Eur. J. 28 (12), e202104249. doi:10.1002/chem.202104249
Sun, H., Chen, L., Xiong, L., Feng, K., Chen, Y., Zhang, X., et al. (2021). Promoting ethylene production over a wide potential window on Cu crystallites induced and stabilized via current shock and charge delocalization. Nat. Commun. 12 (1), 6823. doi:10.1038/s41467-021-27169-9
Sun, K., Cheng, T., Wu, L., Hu, Y., Zhou, J., Maclennan, A., et al. (2017). Ultrahigh mass activity for carbon dioxide reduction enabled by gold–iron core–shell nanoparticles. J. Am. Chem. Soc. 139 (44), 15608–15611. doi:10.1021/jacs.7b09251
Tan, J. C., and Cheetham, A. K. (2011). Mechanical properties of hybrid inorganic–organic framework materials: Establishing fundamental structure–property relationships. Chem. Soc. Rev. 40 (2), 1059. doi:10.1039/c0cs00163e
Tian, Z. Q., and Ren, B. (2004). Adsorption and reaction at electrochemical interfaces as probed by surface-enhanced Raman spectroscopy. Annu. Rev. Phys. Chem. 55, 197–229. doi:10.1146/annurev.physchem.54.011002.103833
van de Voorde, B., Stassen, I., Bueken, B., Vermoortele, F., De Vos, D., Ameloot, R., et al. (2015). Improving the mechanical stability of zirconium-based metal-organic frameworks by incorporation of acidic modulators. J. Mater. Chem. A 3 (4), 1737–1742. doi:10.1039/c4ta06396a
Wang, D., Xu, J., Zhu, Y., Wen, L., Ye, J., Shen, Y., et al. (2021). HKUST-1-derived highly ordered Cu nanosheets with enriched edge sites, stepped (211) surfaces and (200) facets for effective electrochemical CO2 reductionfacets for effective electrochemical CO2 reduction. Chemosphere 278, 130408. doi:10.1016/j.chemosphere.2021.130408
Wang, X., Gao, W. Y., Niu, Z., Wojtas, L., Perman, J. A., Chen, Y. S., et al. (2018). A metal–metalloporphyrin framework based on an octatopic porphyrin ligand for chemical fixation of CO2 with aziridines. Chem. Commun. 54 (10), 1170–1173. doi:10.1039/C7CC08844B
Wang, Y., Tian, Y., Pan, S., and Snyder, S. W. (2022). Catalytic processes to accelerate decarbonization in a net-zero carbon world. ChemSusChem 15 (24), e202201290. doi:10.1002/cssc.202201290
Ward, L., Babinec, S., Dufek, E. J., Howey, D. A., Viswanathan, V., Aykol, M., et al. (2022). Principles of the battery data genome. Joule 6 (10), 2253–2271. doi:10.1016/j.joule.2022.08.008
Xiao, L., Wang, Z., and Guan, J. (2022). 2D MOFs and their derivatives for electrocatalytic applications: Recent advances and new challenges. Coord. Chem. Rev. 472, 214777. doi:10.1016/j.ccr.2022.214777
Xiong, G., Yu, B., Dong, J., Shi, Y., Zhao, B., and He, L. N. (2017). Cluster-based MOFs with accelerated chemical conversion of CO2 through C–C bond formation. Chem. Commun. 53 (44), 6013–6016. doi:10.1039/C7CC01136A
Xu, Q., Prendergast, D., and Qian, J. (2022). Real-Space pseudopotential method for the calculation of 1 s core-level binding energies. J. Chem. Theory Comput. 18 (9), 5471–5478. doi:10.1021/acs.jctc.2c00474
Xu, Q., Sharma, A., Comer, B., Huang, H., Chow, E., Medford, A. J., et al. (2021). Sparc: Simulation package for ab-initio real-space calculations. SoftwareX 15, 100709. doi:10.1016/J.SOFTX.2021.100709
Xu, Q., Wang, S., Xue, L., Shao, X., Gao, P., Lv, J., et al. (2019). Ab initio electronic structure calculations using a real-space Chebyshev-filtered subspace iteration method. J. Phys. Condens. Matter 31 (45), 455901. doi:10.1088/1361-648X/AB2A63
Yang, F., Chen, A., Deng, P. L., Zhou, Y., Shahid, Z., Liu, H., et al. (2019). Highly efficient electroconversion of carbon dioxide into hydrocarbons by cathodized copper–organic frameworks. Chem. Sci. 10 (34), 7975–7981. doi:10.1039/C9SC02605C
Yao, K., Xia, Y., Li, J., Wang, N., Han, J., Gao, C., et al. (2020). Metal–organic framework derived copper catalysts for CO2 to ethylene conversion. J. Mater. Chem. A 8 (22), 11117–11123. doi:10.1039/D0TA02395G
Ye, Y., and Liu, Z. (2021). “APXPS of solid/liquid interfaces,” in Ambient pressure spectroscopy in complex chemical environments’ (Washington, United States: American Chemical Society), 67–92. doi:10.1021/bk-2021-1396.ch004
Zaera, F. (2014). New advances in the use of infrared absorption spectroscopy for the characterization of heterogeneous catalytic reactions. Chem. Soc. Rev. 43 (22), 7624–7663. doi:10.1039/C3CS60374A
Zalomaeva, O. v., Maksimchuk, N. V., Chibiryaev, A. M., Kovalenko, K. A., Fedin, V. P., and Balzhinimaev, B. S. (2013). Synthesis of cyclic carbonates from epoxides or olefins and CO2 catalyzed by metal-organic frameworks and quaternary ammonium salts. J. Energy Chem. 22 (1), 130–135. doi:10.1016/S2095-4956(13)60017-0
Zhang, G., Wei, G., Liu, Z., Oliver, S. R. J., and Fei, H. (2016). A robust sulfonate-based metal–organic framework with permanent porosity for efficient CO2 capture and conversion. Chem. Mater. 28 (17), 6276–6281. doi:10.1021/acs.chemmater.6b02511
Zhang, Y., Li, K., Chen, M., Wang, J., and Liu, J. (2020). Cu/Cu2O nanoparticles supported on vertically ZIF-L-coated nitrogen-doped graphene nanosheets for electroreduction of CO2 to ethanol. ACS Appl. Nano Mater. 3 (1), 257–263. doi:10.1021/acsanm.9b01935
Zhao, K., Liu, Y., Quan, X., Chen, S., and Yu, H. (2017). CO2 electroreduction at low overpotential on oxide-derived Cu/carbons fabricated from metal organic framework. ACS Appl. Mater. Interfaces 9 (6), 5302–5311. doi:10.1021/acsami.6b15402
Zhi, W.-Y., Liu, Y. T., Shan, S. L., Jiang, C. J., Wang, H., and Lu, J. X. (2021). Efficient electroreduction of CO2 to C2-C3 products on Cu/Cu2O@N-doped graphene. J. CO2 Util. 50, 101594. doi:10.1016/j.jcou.2021.101594
Zhou, X., Zhang, Y., Yang, X., Zhao, L., and Wang, G. (2012). Functionalized IRMOF-3 as efficient heterogeneous catalyst for the synthesis of cyclic carbonates. J. Mol. Catal. A Chem. 361–362, 12–16. doi:10.1016/j.molcata.2012.04.008
Keywords: one-pot solution, carbon capture, carbon conversion, C2+ products, electrochemical reduction, metal organic framework, copper catalysts
Citation: Jana A, Snyder SW, Crumlin EJ and Qian J (2023) Integrated carbon capture and conversion: A review on C2+ product mechanisms and mechanism-guided strategies. Front. Chem. 11:1135829. doi: 10.3389/fchem.2023.1135829
Received: 01 January 2023; Accepted: 31 January 2023;
Published: 16 February 2023.
Edited by:
Kun Jiang, Shanghai Jiao Tong University, ChinaReviewed by:
Yanwei Lum, National University of Singapore, SingaporeYifan Ye, University of Science and Technology of China, China
Copyright © 2023 Jana, Snyder, Crumlin and Qian. This is an open-access article distributed under the terms of the Creative Commons Attribution License (CC BY). The use, distribution or reproduction in other forums is permitted, provided the original author(s) and the copyright owner(s) are credited and that the original publication in this journal is cited, in accordance with accepted academic practice. No use, distribution or reproduction is permitted which does not comply with these terms.
*Correspondence: Ethan J. Crumlin, ZWpjcnVtbGluQGxibC5nb3Y=; Jin Qian, anFpYW4yQGxibC5nb3Y=