- 1Center for Organic Photonics and Electronics Research (OPERA), Kyushu University, Fukuoka, Japan
- 2Department of Chemistry and Biochemistry, Kyushu University, Fukuoka, Japan
- 3Department of Chemistry, Graduate School of Science, Nagoya University, Nagoya, Japan
- 4Research Center for Materials Science (RCMS) and Integrated Research Consortium on Chemical Sciences (IRCCS), Nagoya University, Nagoya, Japan
- 5Institute of Transformative Bio-Molecules (WPI-ITbM), Nagoya University, Nagoya, Japan
- 6International Institute for Carbon Neutral Energy Research (WPI-I2CNER), Kyushu University, Fukuoka, Japan
We studied the photophysical and electroluminescent (EL) characteristics of a series of azaborine derivatives having a pair of boron and nitrogen aimed at the multi-resonance (MR) effect. The computational study with the STEOM-DLPNO-CCSD method clarified that the combination of a BN ring-fusion and a terminal carbazole enhanced the MR effect and spin-orbit coupling matrix element (SOCME), simultaneously. Also, we clarified that the second triplet excited state (T2) plays an important role in efficient MR-based thermally activated delayed fluorescence (TADF). Furthermore, we obtained a blue–violet OLED with an external EL quantum efficiency (EQE) of 9.1%, implying the presence of a pronounced nonradiative decay path from the lowest triplet excited state (T1).
1 Introduction
Recently, organic emitters providing narrowband emission attracted intense interest aimed at the display applications in organic light-emitting diodes (OLEDs) (Hall et al., 2020; Teng, et al., 2020; Ha et al., 2021; Monkman 2021) because such emitters can achieve high color purity, i.e., high color reproduction area ratio vs. the National Television System Committee (NTSC) color gamut. At the early dawn of OLED development, fluorescence materials had been widely used as an emitter (Tang and VanSlyke, 1987; Adachi et al., 1988; Dodabalapur, 1997; Hung et al., 1997; Shi and Tang., 1997). However, they provide only 25% electron-hole pair-to- photon conversion efficiency because of the spin-statistical theorem (Rothberg and Lovinger, 1996; Köhler et al., 2009). Instead, room temperature phosphorescence materials have resolved this critical problem, achieving 100% internal quantum efficiency (Baldo et al., 1998; Adachi et al., 2001). Nevertheless, the materials had several disadvantages, such as high cost, board emission spectra, and a rather long triplet lifetime, originating from the precious metal complexes and MLCT emission characters. In 2012, on the other hand, our group reported a state-of-the-art emitter based on thermally activated delayed fluorescence (TADF), realizing 100% upconversion from a lowest excited triplet (T1) to a lowest excited singlet (S1) using simple aromatic compounds (Uoyama et al., 2012). The TADF emitters can harvest both prompt emission by conventional fluorescence and delayed emission from S1 via reverse intersystem crossing (RISC) from T1. After this report, a wide variety of TADF materials have been developed worldwide (Nakanotani et al., 2021). However, the TADF emitters also possess the crucial problem of broad emission spectra due to the emission originating from the donor–acceptor (D-A) configuration, i.e., the formation of CT excitons to achieve a small energy splitting between S1 and T1 (ΔEST) (Endo et al., 2009; Endo et al., 2011; Uoyama et al., 2012).
In 2016, Hatakeyama et al. (2016) reported a new type of TADF emitters, achieving narrowband emission with a full width at half maximum (FWHM) of less than 30 nm. These unique emitters were designed by using the MR effects which can localize their highest occupied and lowest unoccupied molecular orbitals (HOMO and LUMO) on the adjacent atoms constituting heterocyclic aromatic cores, achieving unique HOMO and LUMO separation without using the D-A configuration. Also, due to the rigid molecular structures, the vibrational mode of the core structures was significantly suppressed, providing narrowband emission. Furthermore, very recently, a wide variety of MR-TADF emitters were reported, providing excellent device performance in OLEDs. However, most of the reported MR-TADF emitters have a relatively large ΔEST compared to the well-elaborated donor–acceptor type TADF emitters, resulting in a small RISC rate (<105 s−1) while maintaining a large radiative decay rate (>108 s−1). Therefore, MR-TADF emitters are often used as a terminal emitter in TADF-assisted fluorescence (hyperfluorescence) devices (Nakanotani et al., 2014; Chan et al., 2021). In the recent trend, continuously researchers have been focused on obtaining stable MR-TADF emitters having the compatibility of a narrower FWHM and a large RISC rate by engineering the MR effect to achieve highly stable and high color purity OLEDs (Kondo et al., 2019; Oda et al., 2019).
Previously, we reported the synthesis of MR materials showing narrowband emission with a simple heterocyclic aromatic structure composed of a dibenzo [1,4] azaborine skeleton (BN1)-based core having a single couple of boron (B) and nitrogen (N) (Figure 1A) (Ando et al., 2019). This B-N core is the minimum building block to achieve HOMO-LUMO separation on heterocycles rather than the first reported MR-TADF materials of DABNA (Hatakeyama et al., 2016). Based on the scaffold of BN1, the ring-fused planar fashion of triarylborane and/or triarylamine to expand the π-conjugation would be expected to improve their photophysical properties (BN2, BN3, and BN4). In this report, we studied the detailed photophysical and OLED characteristics of these emitters. As a result, we found the original azaborine backbone has no TADF property, and the planarization of the B- and N-phenyl groups by ring-fusion provides the TADF property. Especially, the carbazole formation enhances the SOC, and the most planar BN4 showed the best TADF characteristics. In addition, it was suggested that the major nonradiative decay of BN4 occurs from the T1 state because the theoretical EQE has a considerably large difference from the experimental EQE depending on the ratio of two nonradiative decays from S1 and T1.
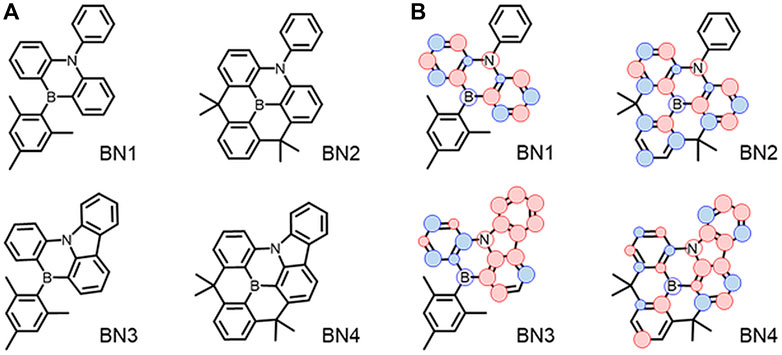
FIGURE 1. (A) Chemical structures of dibenzo [1,4] azaborine derivatives. (B) Schematic illustration of the spin density of hole (red) and electron (blue) on main backbone at S1 state.
2 Results and discussion
2.1 Computational analysis with wave function-based methodology
First, we performed a theoretical study based on a quantum chemical calculation for BN1-4. Recently, Pershin et al. (2019) studied some MR materials, demonstrating the good agreement of ΔEST between theoretical calculation and experimental values by not using the density functional theory (DFT) method but the wave function-based method with the spin-component scaled (SCS) coupled-cluster model (CC2). While we applied these methods to BN1-4 at first, the calculation results did not provide a good agreement with the experimental results as shown in the supplemental information (Supplementary Figure S1, Supplementary Table S1). Also, similar to the several reports of MR-TADF emitters (Hatakeyama et al., 2016; Oda et al., 2019; Lee et al., 2021), the DFT calculation with the B3LYP/6-31G(d) level (Stephen et al., 1994) provided a large difference with the experimental results for S1, T1 levels, and ΔEST. In addition, the values of the oscillator strength (f) were inconsistent with the experimentally estimated ones. Next, we also used the SCS-CC2 level. While it showed better agreement with the experimental ΔEST, it resulted in lower values when N-phenyl units were incorporated into the fused rings. Moreover, a considerable difference was observed in the energy levels of both singlet and triplet excited states.
With the comprehensive evaluation of the calculation methods, we found the Similarity Transformed Equation-Of-Motion Domain-based Local Pair Natural Orbital Coupled-Cluster Singles and Doubles (STEOM-DLPNO-CCSD) method, which is a wave function-based quantum chemistry approach based on EOM-CCSD (Stephen et al., 1994), provides the best results with the experimental values for S1, T1 levels, and ΔEST. The calculated values of singlet, triplet levels, ΔEST, spin-orbit coupling matrix element (SOCME), and f are summarized in Figure 2. The hole and electron distributions at the S1 state for each material are illustrated in Figure 1B. Also, the spin density difference plots for S1, T1, and T2 are shown in Supplementary Figure S2. Because of the higher energy of the S2 level than that of the S1, T1, and T2 levels, it is reasonable to consider only the lower three levels for the emission process in all BNs. Although the absolute f values for the BNs’ S1 levels are overestimated, the order is consistent with the π-extension trend of the ring-fused structures. The ΔEST values, which are also reduced by the π-extension trend, are estimated to be 0.30–0.40 eV, while these values are slightly overestimated, i.e., <0.1 eV, compared to the experimental values. Interestingly, the SOCME showed a large difference for BNs, which should be related to the hole and electron distributions at each state of S1, T1, and T2. In the case of BN1 and BN2, the SOCMEs between S1 and T1 were estimated to be 0.00 cm−1 because of the similar hole and electron distributions, which is the forbidden transition explained by El-Sayed’s rule. Furthermore, since the SOCMEs between S1 and T2 in BN1 and BN2 are rather small values of 0.01 and 0.04 cm−1, respectively, they would show virtually no TADF activity. Instead, BN3 and BN4 are TADF-active. When an N-phenyl group is fused with the BN ring (BN3), the hole distribution of the S1 state locates mainly on the carbazole moiety, and the electron is distributed on the azaborine moiety, inducing the charge transfer (CT) nature. In the T1 state, both hole and electrons are distributed on the carbazole moiety, while they distribute on the azaborine moiety in the T2 state. These spin distribution differences among S1, T1, and T2 enhance the SOCME of BN3 (0.19 and 0.10 cm−1 for S1-T1 and S1-T2, respectively). In the case of BN4, the spin distribution of T1 is almost as same as BN3, while that of the T2 level spreads to the whole molecule. Interestingly, the SOCME of BN4 is as same as that with BN3 (0.12 and 0.08 cm−1 for S1-T1 and S1-T2, respectively).
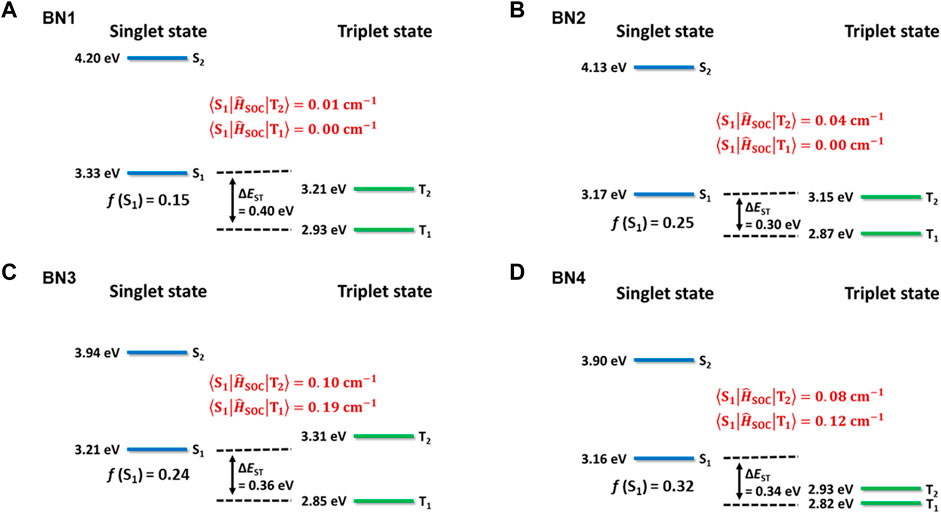
FIGURE 2. Calculated excited state energies for (A) BN1, (B) BN2, (C) BN3, and (D) BN4 at the STEOM-DLPNO-CCSD level of theory. Singlet (S1, S2) and triplet (T1, T2) levels were based on the geometry optimized for each level. Oscillator strength (f) values were indicated for the S1–S0 transition at the S1 state geometry. Spin-orbit coupling (SOC) strengths were estimated by using initial and final state geometries.
2.2 Photophysical properties of BN molecule series in solution
Figure 3 shows the ultraviolet–visible (UV–Vis) absorption and emission spectra of BN1-4 in toluene (1.0 × 10–5 mol L−1). Previously, we demonstrated the estimation method of experimental f values for fluorescence materials using the integral of the molar absorption coefficient (ε) which originates from the absorption component located at the lowest energy, and we applied this method to TADF materials (Hirata et al., 2015; Tsuchiya et al., 2020; Tsuchiya et al., 2021a). Also, the transition dipole moment (Q) and radiative decay rate from an S1 state
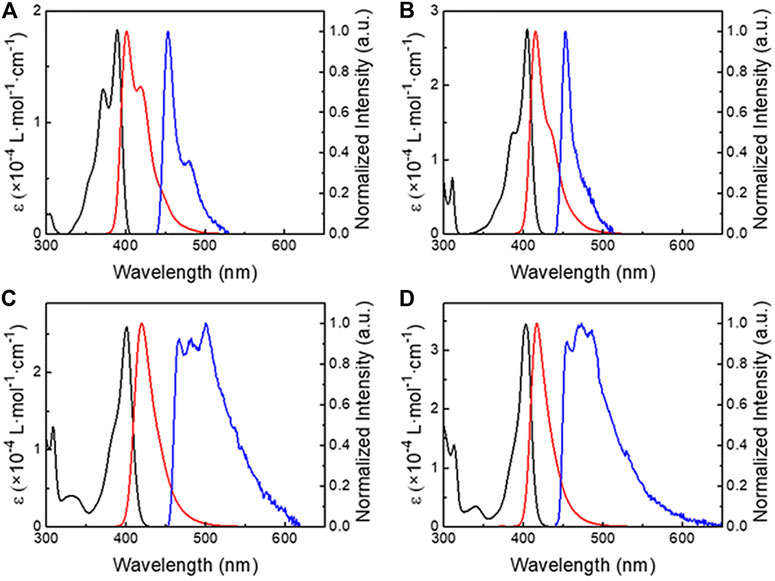
FIGURE 3. Absorption (black line), fluorescence (red line), and phosphorescence (blue line, at 77 K) spectra of (A) BN1, (B) BN2, (C) BN3, (D) BN4 in toluene (1.0 × 10–5 mol L−1).
As expected in the spin distribution on the computational analysis, the N-phenyl planarized ones (BN3 and 4) showed similar broad phosphorescence spectra having vibronic structures. On the other hand, BN1 and 2 showed narrowband phosphorescence. The fluorescence and phosphorescence in all BNs provided similar spectra in various solvents (Supplementary Figure S4). In other words, BNs have less polarity sensitivity for both S1 and T1 energies. The estimated S1, T1 energy levels, and ΔEST values in toluene are summarized in Table 1. In addition, the experimentally obtained
2.3 Thermally activated delayed fluorescence properties from the transient decay curve in a DPEPO-doped film
Because all BNs would show very weak TADF property in solution, we investigated the photophysical properties of BNs in their solid-state films to suppress the nonradiative decays. We used bis [2-(diphenylphosphino) phenyl] ether oxide (DPEPO) as a high T1 host matrix to confine the high T1 energy of BNs (>2.7 eV). In addition, we fabricated BN-doped DPEPO films with a doping ratio of 1 wt% to inhibit the aggregation. The absorption and emission spectra of each BN in DPEPO showed almost the same emission spectra as those in toluene (Table 2 and Supplementary Figure S6). Thus, it was confirmed that BNs do not aggregate significantly in the doped films. The transient PL properties were investigated by a steak camera system with a fourth harmonic Nd:YAG laser (266 nm) under vacuum (Figure 4 and Supplementary Figure S7).
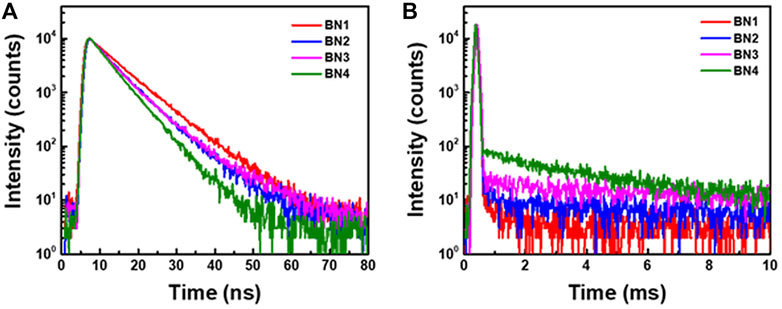
FIGURE 4. Transient PL decay curves of (A) prompt and (B) delayed emissions for BN1, 2, 3, and 4 in DPEPO films (1 wt% doped) were measured with a streak camera under the vacuum at 300 K.
BNs showed a comparable radiative decay rate
2.4 Organic light-emitting diode device employing BN4 as an emitter
Finally, we fabricated an OLED with BN4 as an emitter (Figure 5) with the device structure of indium tin oxide (ITO)/1,1-bis [(di-4-tolylamino) phenyl]cyclohexane (TAPC, 35 nm)/4,4′,4″-tris (carbazol-9-yl)-triphenylamine (TCTA, 10 nm)/1,3-bis (9-carbazol-9-yl) benzene (mCP, 10 nm)/DPEPO doped with 1 wt% of BN4 (30 nm)/DPEPO (10 nm)/2,2′,2"-(1,3,5-benzinetriyl) -tris (1-phenyl-1-H-benzimidazole) (TPBi, 45 nm)/LiF (0.8 nm)/Al (100 nm). The electroluminescence spectrum showed good agreement with the PL spectrum of BN4 in a DPEPO host (λEL,max = 423 nm, FWHM = 31 nm). The CIE coordinates were (0.17, 0.04). The maximum EQE (
where
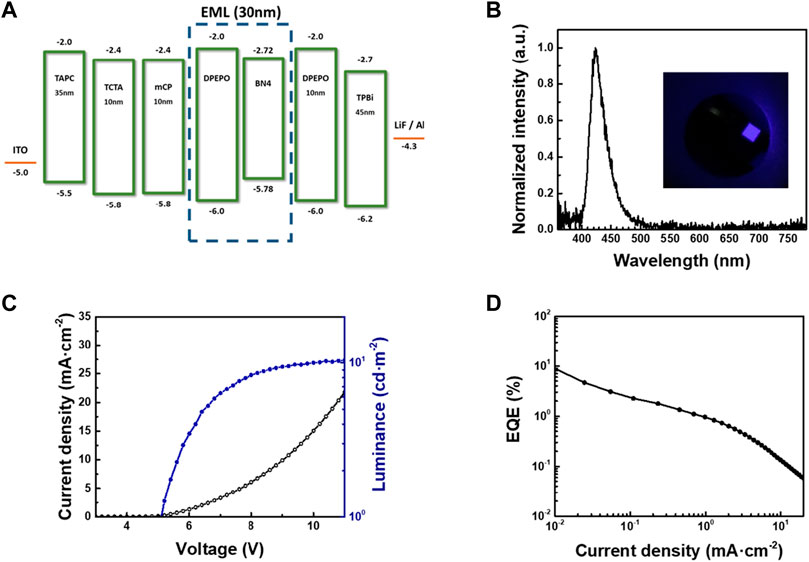
FIGURE 5. OLED device characteristics of BN4; (A) schematic device structure and energy level alignment, (B) normalized EL spectra, (C) J-V-L profile, and (D) EQE-J curves.
Recently, this equation has been widely applied to TADF OLEDs since it is reasonable to apply it for highly efficient emitters, i.e.,
where
3 Conclusion
In this study, we focused on the minimum structural component of an azaborine backbone structure, which is necessary to provide MR-TADF characteristics. By planarization with the ring fusion of B- or N-phenyls, the molecular structure became more rigid, demonstrating clear MR characteristics with suppressing vibronic structures in their spectra. In particular, unlike B-phenyl planarization, which reduces FWHM and ΔEST by simply extending π-conjugation, it was confirmed that the vibronic structures of emission were suppressed and molecular SOCME was improved through planarization of the N-phenyl moiety. Furthermore, we confirmed that the ring fusion of B- or N-phenyls showed a synergistic effect of them. The TADF nature was mainly dependent on the SOCME because BNs showed an appreciably large ΔEST (0.24–0.36 eV). Carbazole formation enhanced the SOC effectively, and further planarization reduced the T2 state energy and promoted the RISC process. Finally, we fabricated the OLED having blue–violet emission (λmax, 423 nm) with EQE = 9.1%, demonstrating good agreement with the maximum theoretical value of EQE = 9.2% when the nonradiative decay from the T1 state is considered. Considering the high PLQY of over 80% in the BN4 film, it clearly indicates that suppressing the nonradiative process from T1 is mandatory for efficient MR-TADF OLED in the blue–violet region.
4 Materials and methods
4.1 Chemicals and instruments
All solvents were used as purchased from the Tokyo Chemical Industry (Tokyo, Japan) or Fuji Film-Wako Chemicals (Tokyo, Japan). All synthesis procedures for BN1-4 were provided in the previous report (Ando et al., 2019). Absorption spectra of the samples were measured using an ultraviolet–visible,-near-infrared spectrometer (Lambda 950-PKA, Perkin-Elmer, MA, United States). Fluorescence and phosphorescence spectra were measured using a spectrofluorometer (FP-8600, JASCO International, Japan). The photoluminescence quantum yield (PLQY) was measured using a PLQY measurement system (Quantaurus-QY, Hamamatsu Photonics, Hamamatsu, Japan). The transient PL decay characteristics of BN4 in toluene were recorded by a dynamic range streak camera system (C10910-01, Hamamatsu Photonics, Hamamatsu, Japan) with a third harmonic YAG laser (355 nm, 10 Hz, PL-2250, EKSPLA, Lithuania) as an excitation source. The transient PL emission and PL decay of BN1, 2, 3, and 4 doped in a DPEPO host film were recorded under vacuum with a streak camera (C4334, Hamamatsu Photonics, Hamamatsu, Japan) with a third harmonic YAG laser (266 nm, 10 Hz, LS-2132UTF, LOTIS TII, Belarus) as an excitation source.
4.2 Theoretical calculations
The BNs were analyzed by DFT calculation with the B3LYP/6-31 + g* (Lee et al., 1988; Beck, 1993) level of theory on Gaussian 16 (Frisch et al., 2016), SCS-CC2 level of theory on TURBOMOLE 7.1.1 (2016), and STEOM-DLPNO-CCSD level of theory on ORCA 4.2.1 software (Neese et al., 2020).
4.3 Photophysical measurement
The photophysical properties of BNs were measured in toluene solution (1.0 × 10–5 mol L−1), which was saturated with inert gas. Also, thermally evaporated films composed of 1 wt% emitter in a DPEPO host were measured under the vacuum condition. The transient PL decay profiles of streak camera measurement were analyzed with the ex-Gauss fitting using Eqs 4, 5; the detailed explanation is written in Supplementary Material S1.
In this time, n = 3 and m = 2 were employed to explain three G curves, i.e., probability density functions, as an instrument-related function (IRF) and bi-exponential curve as an emission decay.
Data availability statement
The original contributions presented in the study are included in the article/Supplementary Material; further inquiries can be directed to the corresponding authors.
Author contributions
JB performed the experimental work for photophysics and device fabrication. MK, NA, and SY designed and synthesized the molecules. JB, X-KC, and Y-TL contributed to theoretical calculation. JB, YT, TN, C-YC, MA, and HN contributed to analysis of the data. JB, YT, and CA wrote the manuscript. All authors reviewed the manuscript.
Funding
This work is supported by Kyulux Inc. JSPS Core-to-core Program (Grant Number: JPJSCCA20180005), and WPI-I2CNER MEXT Japan, KAKENHI Grant 18H05261 from the Japan Society for the Promotion of Science (JSPS) and CREST (JPMJCR21O5), Japan Science and Technology Agency (JST).
Conflict of interest
The authors declare that the research was conducted in the absence of any commercial or financial relationships that could be construed as a potential conflict of interest.
The reviewer GX declared a past co-authorship with the author CA to the handling editor.
Publisher’s note
All claims expressed in this article are solely those of the authors and do not necessarily represent those of their affiliated organizations, or those of the publisher, the editors, and the reviewers. Any product that may be evaluated in this article, or claim that may be made by its manufacturer, is not guaranteed or endorsed by the publisher.
Supplementary material
The Supplementary Material for this article can be found online at: https://www.frontiersin.org/articles/10.3389/fchem.2022.990918/full#supplementary-material
References
Adachi, C., Baldo, M. A., Thompson, M. E., and Forrest, S. R. (2001). Nearly 100% internal phosphorescence efficiency in an organic light emitting device. J. Appl. Phys. 90, 5048–5051. doi:10.1063/1.1409582
Adachi, C., Tokito, S., Tsutsui, T., and Saito, S. (1988). Electroluminescence in organic films with three-layer structure. Jpn. J. Appl. Phys. 27, L269–L271. doi:10.1143/JJAP.27.L269
Ando, M., Sakai, M., Ando, N., Hirai, M., and Yamaguchi, S. (2019). Planarized: B, N -phenylated dibenzoazaborine with a carbazole substructure: Electronic impact of the structural constraint. Org. Biomol. Chem. 17, 5500–5504. doi:10.1039/c9ob00934e
Baldo, M. A., O’Brien, D. F., You, Y., Shoustikov, A., Sibley, S., Thompson, M. E., et al. (1998). Highly efficient phosphorescent emission from organic electroluminescent devices. Nature 395, 151–154. doi:10.1038/25954
Beck, A. D. (1993). Density-functional thermochemistry. III. The role of exact exchange. J. Chem. Phys. 98, 5648–5652. doi:10.1063/1.464913
Chan, C. Y., Tanaka, M., Lee, Y. T., Wong, Y. W., Nakanotani, H., Hatakeyama, T., et al. (2021). Stable pure-blue hyperfluorescence organic light-emitting diodes with high-efficiency and narrow emission. Nat. Photonics 15, 203–207. doi:10.1038/s41566-020-00745-z
Dias, F. B., Penfold, T. J., and Monkman, A. P. (2017). Photophysics of thermally activated delayed fluorescence molecules. Methods Appl. Fluoresc. 5, 012001. doi:10.1088/2050-6120/aa537e
Dodabalapur, A. (1997). Organic light emitting diodes. Solid State Commun. 102, 259–267. doi:10.1016/S0038-1098(96)00714-4
Endo, A., Ogasawara, M., Takahashi, A., Yokoyama, D., Kato, Y., and Adachi, C. (2009). Thermally activated delayed fluorescence from Sn4+-porphyrin complexes and their application to organic light-emitting diodes -A novel mechanism for electroluminescence. Adv. Mat. 21, 4802–4806. doi:10.1002/adma.200900983
Endo, A., Sato, K., Yoshimura, K., Kai, T., Kawada, A., Miyazaki, H., et al. (2011). Efficient up-conversion of triplet excitons into a singlet state and its application for organic light emitting diodes. Appl. Phys. Lett. 98, 083302. doi:10.1063/1.3558906
Frisch, M. J., Trucks, G. W., Schlegel, H. B., Scuseria, G. E., Robb, M. A., Cheeseman, J. R., et al. (2016). Gaussian 16, revision A.03. Wallingford CT: Gaussian Inc.
Fukuzumi, S., Itoh, A., Ohkubo, K., and Suenobu, T. (2015). Size-selective incorporation of donor–acceptor linked dyad cations into zeolite Y and long-lived charge separation. RSC Adv. 5, 45582–45585. doi:10.1039/c5ra06165b
Ha, J. M., Hur, S. H., Pathak, A., Jeong, J. E., and Woo, H. Y. (2021). Recent advances in organic luminescent materials with narrowband emission. NPG Asia Mat. 13, 53. doi:10.1038/s41427-021-00318-8
Hall, D., Suresh, S. M., dos Santos, P. L., Duda, E., Bagnich, S., Pershin, A., et al. (2020). Improving processability and efficiency of resonant TADF emitters: A design strategy. Adv. Opt. Mat. 8, 1901627. doi:10.1002/adom.201901627
Hatakeyama, T., Shiren, K., Nakajima, K., Nomura, S., Nakatsuka, S., Kinoshita, K., et al. (2016). Ultrapure blue thermally activated delayed fluorescence molecules: Efficient HOMO-LUMO separation by the multiple resonance effect. Adv. Mat. 28, 2777–2781. doi:10.1002/adma.201505491
Hirata, S., Sakai, Y., Masui, K., Tanaka, H., Lee, S. Y., Nomura, H., et al. (2015). Highly efficient blue electroluminescence based on thermally activated delayed fluorescence. Nat. Mat. 14, 330–336. doi:10.1038/nmat4154
Hung, L. S., Tang, C. W., and Mason, M. G. (1997). Enhanced electron injection in organic electroluminescence devices using an Al/LiF electrode. Appl. Phys. Lett. 70, 152–154. doi:10.1063/1.118344
Kondo, Y., Yoshiura, K., Kitera, S., Nishi, H., Oda, S., Gotoh, H., et al. (2019). Narrowband deep-blue organic light-emitting diode featuring an organoboron-based emitter. Nat. Photonics 13, 678–682. doi:10.1038/s41566-019-0476-5
Lee, C., Yang, W., and Parr, R. G. (1988). Development of the Colle-Salvetti correlation-energy formula into a functional of the electron density. Phys. Rev. B 37, 785–789. doi:10.1103/PhysRevB.37.785
Lee, Y. T., Chan, C. Y., Tanaka, M., Mamada, M., Balijapalli, U., Tsuchiya, Y., et al. (2021). Investigating HOMO energy levels of terminal emitters for realizing high-brightness and stable TADF-assisted fluorescence organic light-emitting diodes. Adv. Electron. Mat. 7, 2001090–2001099. doi:10.1002/aelm.202001090
Masui, K., Nakanotani, H., and Adachi, C. (2013). Analysis of exciton annihilation in high-efficiency sky-blue organic light-emitting diodes with thermally activated delayed fluorescence. Org. Electron. 14, 2721–2726. doi:10.1016/j.orgel.2013.07.010
Monkman, A. (2021). Why do we still need a stable long lifetime deep blue OLED emitter? ACS Appl. Mat. Interfaces 14, 20463–20467. doi:10.1021/acsami.1c09189
Nakanotani, H., Higuchi, T., Furukawa, T., Masui, K., Morimoto, K., Numata, M., et al. (2014). High-efficiency organic light-emitting diodes with fluorescent emitters. Nat. Commun. 5, 4016–4017. doi:10.1038/ncomms5016
Nakanotani, H., Tsuchiya, Y., and Adachi, C. (2021). Thermally-activated delayed fluorescence for light-emitting devices. Chem. Lett. 50, 938–948. doi:10.1246/cl.200915
Neese, F., Wennmohs, F., Becker, U., and Riplinger, C. (2020). The ORCA quantum chemistry Program package. J. Chem. Phys. 152, 224108. doi:10.1063/5.0004608
Oda, S., Kawakami, B., Kawasumi, R., Okita, R., and Hatakeyama, T. (2019). Multiple resonance effect-induced sky-blue thermally activated delayed fluorescence with a narrow emission band. Org. Lett. 21, 9311–9314. doi:10.1021/acs.orglett.9b03342
Pershin, A., Hall, D., Lemaur, V., Sancho-Garcia, J. C., Muccioli, L., Zysman-Colman, E., et al. (2019). Highly emissive excitons with reduced exchange energy in thermally activated delayed fluorescent molecules. Nat. Commun. 10, 597–7. doi:10.1038/s41467-019-08495-5
Rothberg, L. J., and Lovinger, A. J. (1996). Status of and prospects for organic electroluminescence. J. Mat. Res. 11, 3174–3187. doi:10.1557/JMR.1996.0403
Samanta, P. K., Kim, D., Coropceanu, V., and Brédas, J.-L. (2017). Up-conversion intersystem crossing rates in organic emitters for thermally activated delayed fluorescence: Impact of the nature of singlet vs triplet excited states. J. Am. Chem. Soc. 139, 4042–4051. doi:10.1021/jacs.6b12124
Shi, J., and Tang, C. W. (1997). Doped organic electroluminescent devices with improved stability. Appl. Phys. Lett. 70, 1665–1667. doi:10.1063/1.118664
Stephen, P. J., Devlin, F. J., Chabalowski, C. F., and Frisch, M. J. (1994). Ab initio calculation of vibrational absorption and circular dichroism spectra using density functional force fields. J. Phys. Chem. 98, 11623–11627. doi:10.1021/j100096a001
Tang, C. W., and VanSlyke, S. A. (1987). Organic electroluminescent diodes. Appl. Phys. Lett. 51, 913–915. doi:10.1063/1.98799
Teng, J. M., Wang, Y. F., and Chen, C. F. (2020). Recent progress of narrowband TADF emitters and their applications in OLEDs. J. Mat. Chem. C 8, 11340–11353. doi:10.1039/d0tc02682d
Tsuchiya, Y., Diesing, S., Bencheikh, F., Wada, Y., dos Santos, P. L., Kaji, H., et al. (2021a). Exact solution of kinetic analysis for thermally activated delayed fluorescence materials. J. Phys. Chem. A 125, 8074–8089. doi:10.1021/acs.jpca.1c04056
Tsuchiya, Y., Ishikawa, Y., Lee, S. H., Chen, X. K., Brédas, J. L., Nakanotani, H., et al. (2021b). Thermally activated delayed fluorescence properties of trioxoazatriangulene derivatives modified with electron donating groups. Adv. Opt. Mat. 9, 2002174–2002178. doi:10.1002/adom.202002174
Tsuchiya, Y., Tsuji, K., Inada, K., Bencheikh, F., Geng, Y., Kwak, H. S., et al. (2020). Molecular design based on donor-weak donor scaffold for blue thermally-activated delayed fluorescence designed by combinatorial DFT calculations. Front. Chem. 8, 403–411. doi:10.3389/fchem.2020.00403
Keywords: TADF, thermally activated delayed fluorescence, multi-resonance, azaborine, blue–violet OLED
Citation: Bae J, Sakai M, Tsuchiya Y, Ando N, Chen X-K, Nguyen TB, Chan C-Y, Lee Y-T, Auffray M, Nakanotani H, Yamaguchi S and Adachi C (2022) Multiple resonance type thermally activated delayed fluorescence by dibenzo [1,4] azaborine derivatives. Front. Chem. 10:990918. doi: 10.3389/fchem.2022.990918
Received: 11 July 2022; Accepted: 17 August 2022;
Published: 19 September 2022.
Edited by:
Youhei Takeda, Osaka University, JapanReviewed by:
Guohua Xie, Wuhan University, ChinaNadzeya Kukhta, University of Washington, United States
Copyright © 2022 Bae, Sakai, Tsuchiya, Ando, Chen, Nguyen, Chan, Lee, Auffray, Nakanotani, Yamaguchi and Adachi. This is an open-access article distributed under the terms of the Creative Commons Attribution License (CC BY). The use, distribution or reproduction in other forums is permitted, provided the original author(s) and the copyright owner(s) are credited and that the original publication in this journal is cited, in accordance with accepted academic practice. No use, distribution or reproduction is permitted which does not comply with these terms.
*Correspondence: Youichi Tsuchiya, dHN1Y2hpeWFAb3BlcmEua3l1c2h1LXUuYWMuanA=; Shigehiro Yamaguchi, eWFtYWd1Y2hpQGNoZW0ubmFnb3lhLXUuYWMuanA=; Chihaya Adachi, YWRhY2hpQGNzdGYua3l1c2h1LXUuYWMuanA=