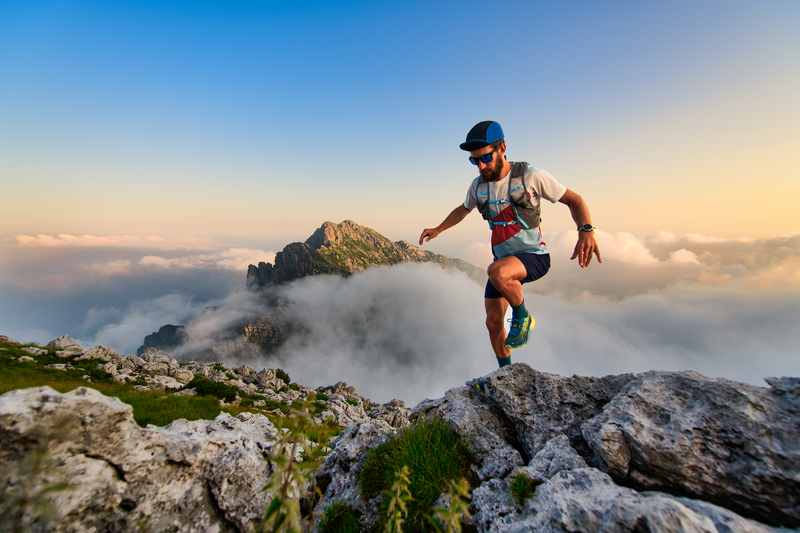
95% of researchers rate our articles as excellent or good
Learn more about the work of our research integrity team to safeguard the quality of each article we publish.
Find out more
MINI REVIEW article
Front. Chem. , 07 September 2022
Sec. Inorganic Chemistry
Volume 10 - 2022 | https://doi.org/10.3389/fchem.2022.976781
This article is part of the Research Topic Radioiodine Detection and Management View all 8 articles
Reactions between phosphoric acid [H3PO4] or ammonium hydrogen phosphates [i.e., NH4H2PO4, (NH4)2HPO4] and halide salts can be used to dehalogenate (remove halides from) salt-based waste streams, where the process of removing halides yields products that have more efficient disposal pathways for repository storage. In this context, the term efficiency is defined as higher waste loadings and simplified immobilization processes with potential for recycle of certain salt components (e.g., 37Cl as H37Cl or NH437Cl). The main streams identified for these processes are nuclear wastes generated during electrochemical reprocessing of used nuclear fuel as well as used halide salts from molten salt reactor operation. The potential byproducts of these reactions are fairly consistent across the range of halide species (i.e., F, Cl, Br, I) where the most common are hydrogen halides [e.g., HCl(g)] or ammonium halides (e.g., NH4Cl). However, trihalide compounds (e.g., NCl3), nitrogen triiodide ammine adducts [NI3·(NH3)x], and ammonium triiodide (NH4I3) are also possible. Several of these byproducts (i.e., NCl3, NBr3, NI3, and NH4I3) are shock-sensitive contact explosives so their production in these processes must be tracked and carefully controlled, which includes methods of immediate neutralization upon production such as direct transport to a caustic scrubber for dissolution. Several benefits arise from utilizing H3PO4 as the phosphate additive during dehalogenation reactions for making iron phosphate waste forms including more oxidized iron (higher Fe3+:Fe2+ ratios), higher chemical durabilities, and the avoidance of trihalides, but the byproducts are hydrogen halides, which are corrosive and require special handling.
Salt-based nuclear wastes can be generated through electrochemical reprocessing (pyroprocessing) or operation of molten salt reactors (MSRs). If the radionuclide-containing salt wastes cannot be directly disposed in a nuclear waste repository, it is possible that these wastes can be treated prior to disposal to improve the available options for waste form production (Riley, 2020). One of these treatment processes includes dehalogenation of the salt where the halides are removed and 1) recovered and recycled, 2) immobilized in a different form, or 3) potentially discarded. The primary goal of partitioning the wastes is to find more suitable and efficient waste forms for the different waste constituents since halide solubilities in traditional borosilicate glass nuclear waste forms are very low and the retentions of halides during melting to create glassy waste forms are also low (Hrma, 2010; Riley et al., 2012; Riley et al., 2014). Secondary goals include the benefit of recovering valuable isotopes like 37Cl for applications like MSRs. While both 35Cl and 37Cl are stable isotopes, the motivation for using 37Cl-enriched salts for MSRs are 1) to prevent neutron activation of natural 35Cl to the long-lived radioisotope of 36Cl (t1/2 = 3.01 × 105 years) and 2) to decrease parasitic neutron absorption (McFarlane et al., 2019). Having long-lived radioisotopes in nuclear wastes drives repository dose calculations so they should be minimized, if possible, to save on disposal costs and potential environmental impacts. Since radioiodine is also an issue from a repository dose standpoint due to long-lived 129I (t1/2 = 1.57 × 107 years), selective removal of iodine from these salts and maximizing iodine loading in a high efficiency waste form is also desired. Bromine is a fission product present in nuclear waste streams (Riley et al., 2018) and, unlike the other halogens, is a liquid at room temperature with a boiling temperature of 58.8°C (Lide 2007-2008). Following dehalogenation, immobilization of the fission product oxides could be realized in a phosphate waste form including phosphate glass (e.g., iron phosphate, iron aluminophosphate, aluminophosphate) (Day et al., 1998; Siemer, 2013a; Siemer, 2013b; Day and Ray, 2013; Bai et al., 2021) and/or phosphate-based crystalline matrices (e.g., monazite) (McCarthy et al., 1978; Boatner et al., 1980).
Demonstrated methods for dehalogenating chloride-based salt wastes include reactions between chloride-based salts with ammonium phosphates [e.g., NH4H2PO4 and (NH4)2HPO4] shown in Eqs 1, 2 (Donze et al., 2000; Donze et al., 2001; Bekaert et al., 2006; Riley et al., 2020), reactions with H3PO4 such as that shown in Eq. 3 (Lavrinovich et al., 2003; Park et al., 2007a; Park et al., 2007b; Park et al., 2008; Park et al., 2011; Siemer, 2012; Lee et al., 2019), reactions with hydrogen-based zeolites like ultrastable H-Y zeolite shown in Eq. 4 (Wasnik et al., 2019a; Wasnik et al., 2019b), or through high-temperature reactions with steam to produce metal oxides shown in Eq. 5 (Sato et al., 2002). A third ammonium phosphate material, (NH4)3PO4, will be mentioned here for completion purposes, but it is quite unstable; however, it could potentially be adapted for these types of processes.
Dehalogenation processes are useful for waste form production of chloride-based salt wastes because it allows for much higher waste loadings in the final waste form for the remaining fission products because the halides no longer limit this loading capacity as it does with other salt waste form options like crystalline matrices with physical halide limits such as chlorosodalite [e.g., Na8(AlSiO4)6Cl] (Vance et al., 2012) and chlorapatite [e.g., Ca5(PO4)3Cl] (Vance et al., 2012) due to crystal chemistry stoichiometries (Figure 1B). When the salt loading limits are exceeded for crystalline-based halide-host matrices, residual salts can be observed (Figure 1B), which are not a chemically durable or stable form for long-term disposal.
FIGURE 1. (A) Gibbs free energies of formation for various ammonium halide salts (Riley et al., 2020). (B) Residual halite (NaCl) mass, based on X-ray diffraction data, as a function of salt loading used to make glass-bonded sodalite waste forms showing that residual salts are observed when the salt fractions are too high (>8 mass%) due to crystal stoichiometry limits (Riley et al., 2017). (C) Powder X-ray diffraction data showing the majority phase of NH4Cl with a minor NH4I phase in solid condensates recovered after a reaction between NH4H2PO4 and a Cl/I-containing salt simulant at temperatures up to 600°C in an alumina crucible (Riley et al., 2020). Parts (A,C) of this figure were modified from the originals by Riley et al. (2020) and were reprinted with permission. Copyright Elsevier (2020). Part (B) was modified from the original by Riley et al. (2017) and reprinted with permission. Copyright Elsevier (2017).
Fluoride salts can be processed using phosphate precursors as well. Wang et al. (2004) demonstrated that NH4H2PO4 can be used to defluorinate LiF salt with a byproduct of NH4F through Eq. 6 or produce NH4F through the reaction between HF(g) and NH3(g) as shown in Eq. 7. It is likely that these types of reversible reactions could take place at different times in the same system depending on the experimental conditions. Regarding Eq. 7, both hydrogen halides (e.g., HCl) and NH3(g) can be found as byproducts of these reactions as well as in the decomposition reactions of the phosphate reagents. However, to the knowledge of the authors, no such studies have been performed starting from pure iodine-containing salts. In a study by Xiang et al. (2019), NH4H2PO4, Cs2CO3, SrCO3, PbBr2, and NaBr were reacted together resulting in a 45P2O5-20PbBr2-10NaBr-13Cs2O-12SrO glass containing CsPbBr3 crystals after a mechanical stress was applied. This study provides evidence of Br retention after heating NH4H2PO4 and Br-containing compounds to 680°C in air.
These types of reactions can be studied in real-time using characterization techniques like differential scanning calorimetry (DSC; i.e., phase change temperatures, heats of reaction), thermogravimetric analysis (TGA; i.e., mass loss over a range of changing temperatures and/or times), evolved gas analysis (EGA; i.e., identification of off-gas species from the reactions) with an attached gas chromatograph and mass spectrometer, or hot stage X-ray diffraction. In a recent study (Riley et al., 2021a), EGA was utilized to study NH4Cl decomposition as well as monitor the reactions between NH4H2PO4 and KCl. This study showed similar byproducts of NH3, HCl, and H2O for both of these experiments at temperatures as low as ∼200–300°C providing evidence that heat treatment temperatures, heating rates, and temperature dwell times are important parameters for preventing decomposition of the byproducts if ammonium halide salt products are desired [Eq. 8].
Thermodynamic calculations performed using HSC Chemistry show that the ammonium halides should form spontaneously based on negative Gibbs free energies of formation (ΔGf°) across the temperature range of 0 ≤ T ≤ 600°C as shown in Figure 1A (Riley et al., 2020). Also, the ΔGf° values show that the formation preference of the ammonium halides is in the order of NH4F → NH4Cl → NH4Br → NH4I with the more favorable reactions being the lighter ammonium-halide complexes (Figure 1A and Figures 2E–H). In the presence of salt simulants with both chlorine and iodine, both NH4Cl and NH4I were observed in the solid condensates after reactions with NH4H2PO4 at temperatures up to 600°C (Figure 1C) (Riley et al., 2020). However, what these calculations did not show was the formation of other, unwanted potential byproducts when nitrogen-containing reactants are used to dehalogenate some of these salts.
FIGURE 2. Potential halogen-based byproducts generated during dehalogenation processes including (A–D) hydrogen halides; (E–H) ammonium halides; (I–L) nitrogen trihalides; (M) NI3·(NH3)x ammine adduct where x = 1, 2, 3, 5, or 12; and (N) NH4I3. The yellow triangles (!) for some species indicate additional hazards that are described in the text in more detail.
In reactions involving nitrogen-containing compounds and halide vapors, complexes such as nitrogen trihalides (i.e., NF3, NCl3, NBr3, and NI3) can form as well as ammonium triiodide (e.g., NH4I3) or ammines (adducts) of ammonia nitrogen triiodide [NI3·(NH3)x] where x = 1, 2, 3, 5, or 12 according to Matyáš and Pachman (2013) (Figure 2M). The pure hydrogen halide compounds HF, HCl, HBr, and HI are all colorless gases at room temperature with different boiling temperatures (Tbs) of 19.5°C, −85°C, −66.8°C, and −35.4°C, respectively (Lide 2007-2008; Matyáš and Pachman, 2013) (see Figures 2A–D). The ammonium halide salts are all white in appearance and some can decompose when heated into NH3(g) and hydrogen halides [e.g., Eq. 8]. For instance, NH4F decomposes at 100°C, NH4Cl decomposes at 338°C, NH4Br boils at 452°C, and NH4I sublimes at 235°C (Lide 2007-2008; Matyáš and Pachman, 2013). The nitrogen trihalide compounds NF3, NCl3, and NI3 all behave differently with Tbs of −129.1°C, 71°C, and −20°C (sublimation temperature) and have different appearances of a colorless gas, a yellow oily liquid, and a dark solid, respectively (Lide 2007-2008; Matyáš and Pachman, 2013) (see Figures 2I, J, L). The compound NBr3 is a deep red solid, and the Tb could not be found reported in the literature but it is known to be explosive at temperatures as low as −100°C even under slight disturbances (Jander, 1976) (see Figure 2K), likely making the Tb determination difficult. The ammine compounds of NI3·(NH3)x are black-colored crystals and this is the expected appearance of NH4I3 as well (Fedoroff et al., 1975) (see Figure 2N).
The primary concern with NCl3, NBr3, NI3, and NH4I3 is that they are known contact explosives (Matyáš and Pachman, 2013); NF3 is not a contact explosive but it is both a toxic gas and greenhouse gas with high global warming potential (Tsai, 2008). Contact explosives are highly unstable materials that can react or explode violently when exposed even to very small amounts of external energy (e.g., gentle contact, sound, α particles, light, spark discharge, mild heating) or strong light and can do so in the absence of oxygen (e.g., in an inert glovebag, glovebox, or hot cell) (Henderson, 1922; Meerkämper, 1954; Bowden, 1958; Fedoroff et al., 1975; Matyáš and Pachman, 2013). These high-energy reactions can proceed to produce diatomic halide gases [e.g., I2(g)] along with other byproducts documented through experimentation (or proposed) in Eqs 9–11 (Holleman and Wiberg, 2001) and are often used in chemistry demonstrations for students.
Alternatively, both NH4I3 and NI3 can be neutralized chemically through reactions with high-pH solutions such as those present within a caustic scrubber whereby the complexes are dissolved and dissociate into more stable (less dangerous) species. Aqueous caustic scrubbers are often used in nuclear applications to neutralize acidic species and an alternative to this approach with similar capabilities would be a nonaqueous molten hydroxide scrubber (Haefner and Tranter, 2007; Riley et al., 2016; Riley et al., 2019; Andrews et al., 2021; Bollinger et al., 2022). These acids include the hydrogen halide acids (i.e., HF, HCl, HBr, and HI; Figures 2A–D), which are likely byproducts when H3PO4 or ammonium phosphates are present, all of which can be neutralized through the hydroxide ions present within caustic scrubbers. However, it is possible that other acids will be present in these streams as well depending on the application (e.g., HNO3), which would also be neutralized by the caustic scrubber or molten hydroxide scrubber, which is a secondary benefit.
Despite the volatile and solid-condensable byproducts [e.g., hydrogen halide gases, H2O(g), N2(g), NH3(g), ammonium halides] and the initial salt chemistry, if these reactions are performed in air, it is likely that the material remaining in the crucible after dehalogenation of halide salts with phosphate precursors [i.e., H3PO4, NH4H2PO4, (NH4)2HPO4] would be fairly consistent in composition no matter which phosphate reactant is used where the salt cations are converted from halides to oxides within a P2O5 matrix [Eqs 1–3, 5 above]. This is one of the more important benefits of dehalogenation as it greatly simplifies the next steps required to fully immobilize the remaining product, which can be reacted with glass-forming chemicals (e.g., Fe2O3) to produce a chemically durable waste form for disposal in a nuclear waste repository (Park et al., 2007a; Park et al., 2007b; Park et al., 2008; Park et al., 2011; Siemer, 2012; Ebert et al., 2018; Ebert and Fortner, 2019a; Ebert and Fortner, 2019b; Lee et al., 2019; Ebert and Fortner, 2020; Stariha and Ebert, 2020; Riley et al., 2021b; Stariha and Ebert, 2021).
The fates of the halides following dehalogenation need to be considered. If halide recycle is desired such as the recovery of valuable 37Cl from MSR-based wastes, capture as HCl or NH4Cl should provide multiple pathways for reuse. One such option for 37Cl recycle is to use NH4Cl to convert U0 to UCl3 that potentially could be returned to MSRs as a fuel source or as an oxidant for electrochemical reprocessing (Herrmann, 2017; Frank et al., 2018; Riley et al., 2020). For Cl, Br, and I, if these are captured in caustic scrubbers, the products can likely be immobilized directly in a halide-specific waste form like sodalite or apatite starting from these halide-containing solutions and reacting them with reagents (e.g., zeolites) at room temperature and atmospheric pressure or at elevated temperatures and pressures in an autoclave (Henderson and Taylor, 1978; Weller and Wong, 1989; Vance et al., 2012; Cao et al., 2017; Chong et al., 2017; Nam et al., 2018). For fluorine, it is likely that captured fluoride byproducts could be discarded or immobilized in a fluoride-based waste form like a CaF2-based glass-ceramic waste form (Gregg et al., 2020).
When formulating and synthesizing phosphate glasses containing high alkali contents, previous work can be drawn upon as well such as the work done with aluminophosphates, iron aluminophosphates, and iron phosphates where a range of phosphate precursors were used to produce glasses including P2O5, H3PO4, NaPO3, Al(PO3)3, AlPO4, and NH4H2PO4 (Brow, 1993; Brow et al., 1993; Day et al., 1998; Mesko et al., 2000; Siemer, 2012; Stefanovsky et al., 2014; Stefanovsky et al., 2017; Bai et al., 2021). In a study by Bai et al. (2021) directly comparing the same glass compositions produced with H3PO4 with those made using NH4H2PO4, glasses made with H3PO4 showed significantly higher Fe3+:Fe2+ ratios based on Mössbauer spectroscopy, which can lead to more chemically resistant (higher chemical durability) waste forms (Yu et al., 1997). This is another benefit for using H3PO4 as the phosphate additive when producing iron phosphate waste forms as opposed to ammonium hydrogen phosphates.
Based on the information provided above, several potentially problematic species could be generated from reacting phosphates with halide salt streams. The main hazards include the corrosive hydrogen halides (i.e., HF, HCl, HBr, and HI) and shock-sensitive trihalide compounds (i.e., NCl3, NBr3, NI3, and NH4I3). As previously discussed, the thermal stabilities of all possible byproducts vary extensively meaning that processes and facilities for managing these byproducts will likely differ for each halide-based salt. The phase-change (Tbs) and decomposition temperatures reported here are for pure compounds. Thus, these temperatures do not represent the actual conditions expected where water is present as a byproduct that will result in dilution (and therefore adjustments to the phase-change temperatures) of the pure compounds. Also, several of these byproducts have very low Tbs and, if produced, would likely be vented as gases. However, it seems that after careful selection of the phosphate precursor, reaction temperatures, reaction times, the molar ratios of the scavenging reactant cation to the total halide content (e.g., NH4+:Cl−, H+:Cl−), and byproduct management, several options exist for removing the halides from salt-based nuclear wastes containing fission-product (Riley et al., 2020; Riley et al., 2021a). Since the primary MSR designs currently under consideration are either chloride-based or fluoride-based, these types of processes are possible for recycling the valuable 37Cl from chloride-based MSRs or removing the fluorine from fluoride-based wastes so that more effective waste management options are available for storing the fission product cations, such as phosphate waste forms like glass, crystallized glass, or glass ceramics (Siemer, 2012; Zhang et al., 2013; Liu et al., 2019; Riley et al., 2019; Riley et al., 2020; Wang et al., 2020; Riley et al., 2021a; Riley et al., 2021b). Management of the halide-based byproducts for waste disposal depends on the specific halide distribution and isotopes present for each, which will likely include some iodine-based species. Separate potential waste form options exist for halide-based species including mineral synthesis from solutions (e.g., caustic scrubbers) (Bollinger et al., 2022) into crystalline matrices like apatite [e.g., Ca5(PO4)3F, Ca5(PO4)3Cl, Pb10(VO4)6I2] (Metcalfe and Donald, 2004; Donald et al., 2007; Metcalfe et al., 2008; Vance et al., 2012; Cao et al., 2017), spodiosite [e.g., Ca2(PO4)Cl] (Metcalfe et al., 2008; Vance et al., 2012), and sodalite [e.g., Na8(AlSiO4)6Cl, Na8(AlSiO4)6I2] (Strachan and Babad, 1979; Nakazawa et al., 1999; Vance et al., 2012; Lepry et al., 2013; Chong et al., 2020).
This paper provides a very brief overview of the types of reactions and byproducts that can be expected when reacting halide-containing nuclear salt wastes with phosphate precursors such as H3PO4, NH4H2PO4, and (NH4)2HPO4. While most of the byproducts are very manageable from these types of reactions, the primary concerns come from potential trihalides that can form under certain conditions including NCl3(s), NBr3(s), NI3(s), and NH4I3(s), which are all shock-sensitive contact explosives. While these are potential byproducts from the reactions described herein, other byproducts are also possible from such streams that do not pose these types of issues such as ammonium halides (e.g., NH4I) and dihalides [e.g., I2(g)]. Due to the inherent instabilities with most of the triiodides, is also likely that these complexes will undergo decomposition prior to condensing depending on the processing conditions. In any case, removing the condensate products of these reactions and transporting them towards a caustic scrubber or molten hydroxide scrubber should be an adequate method for neutralization and preventing downstream transport or unwanted release to the environment. Formation of the triiodides can be avoided if H3PO4 is used instead of the ammonium hydrogen phosphates. The hydrogen halide byproducts can be neutralized in a caustic scrubber.
BR and SC participated in the preparation of the original draft. Both authors listed have made a substantial, direct, and intellectual contribution to the work and approved it for publication.
This work was supported by the United States Department of Energy (DOE) Office of Nuclear Energy’s Material Recovery and Waste Form Development (MRWFD) Campaign. Pacific Northwest National Laboratory (PNNL) is operated by Battelle Memorial Institute for the DOE under contract DE-AC05-76RL01830.
The authors declare that the research was conducted in the absence of any commercial or financial relationships that could be construed as a potential conflict of interest.
The reviewer DS declared a shared affiliation with the authors to the handling editor at the time of review.
All claims expressed in this article are solely those of the authors and do not necessarily represent those of their affiliated organizations, or those of the publisher, the editors and the reviewers. Any product that may be evaluated in this article, or claim that may be made by its manufacturer, is not guaranteed or endorsed by the publisher.
The Supplementary Material for this article can be found online at: https://www.frontiersin.org/articles/10.3389/fchem.2022.976781/full#supplementary-material
Andrews, H. B., McFarlane, J., Chapel, A. S., Ezell, N. D. B., Holcomb, D. E., de Wet, D., et al. (2021). Review of molten salt reactor off-gas management considerations. Nucl. Eng. Des. 385, 111529. doi:10.1016/j.nucengdes.2021.111529
Bai, J., Brow, R. K., Kim, C.-W., Sandineni, P., and Choudhury, A. (2021). Redox effects on the structure and properties of Na-Mo-Fe-phosphate glasses. J. Non. Cryst. Solids 557, 120573. doi:10.1016/j.jnoncrysol.2020.120573
Bekaert, E., Montagne, L., Palavit, G., Delevoye, L., Kunegel, A., and Wattiaux, A. (2006). Conversion of tin and alkali chlorides to phosphates and vitrification into silicate glasses. J. Non. Cryst. Solids 352 (38), 4112–4121. doi:10.1016/j.jnoncrysol.2006.06.007
Boatner, L. A., Beall, G. W., Abraham, M. M., Finch, C. B., Huray, P. G., and Rappaz, M. (1980). “Monazite and other lanthanide orthophosphates as alternate actinide waste forms,” in Advances in Nuclear Science & Technology. Editor C. J. M. NorthrupJr. (Boston, MA: Springer), 289–296.
Bollinger, D. L., Erickson, J., Bussey, J. M., and McCloy, J. S. (2022). Process optimization of caustic scrubber and iodine-129 immobilization in sodalite-based waste forms. MRS Adv. 7 (5), 110–116. doi:10.1557/s43580-022-00229-y
Bowden, F. P. (1958). The initiation of explosion by neutrons, α-particles and fission products. Proc. Proc. R. Soc. A 246, 216–219.
Brow, R. K., Kirkpatrick, R. J., and Turner, G. L. (1993). Nature of alumina in phosphate glass: II, structure of sodium aluminophosphate glass. J. Am. Ceram. Soc. 76 (4), 919–928. doi:10.1111/j.1151-2916.1993.tb05316.x
Brow, R. K. (1993). Nature of alumina in phosphate glass: I, properties of sodium aluminophosphate glass. J. Am. Ceram. Soc. 76 (4), 913–918. doi:10.1111/j.1151-2916.1993.tb05315.x
Cao, C., Chong, S., Thirion, L., Mauro, J. C., McCloy, J. S., and Goel, A. (2017). Wet chemical synthesis of apatite-based waste forms - a novel room temperature method for the immobilization of radioactive iodine. J. Mat. Chem. A 5 (27), 14331–14342. doi:10.1039/C7TA00230K
Chong, S., Peterson, J. A., Nam, J., Riley, B. J., and McCloy, J. S. (2017). Synthesis and characterization of iodosodalite. J. Am. Ceram. Soc. 100 (5), 2273–2284. doi:10.1111/jace.14772
Chong, S., Riley, B. J., Asmussen, R. M., Lawter, A. R., Bruffey, S. H., Nam, J., et al. (2020). Iodosodalite synthesis with hot isostatic pressing of precursors produced from aqueous and hydrothermal processes. J. Nucl. Mater. 538, 152222. doi:10.1016/j.jnucmat.2020.152222
Day, D. E., and Ray, C. S. (2013). A Review of iron phosphate Glasses and Recommendations for vitrifying hanford waste. INL/EXT-13-30389. Idaho Falls, ID: Idaho National Laboratory.
Day, D. E., Wu, Z., Ray, C., and Hrma, P. (1998). Chemically durable iron phosphate glass waste forms. J. Non. Cryst. Solids 241, 1–12. doi:10.1016/s0022-3093(98)00759-5
Donald, I. W., Metcalfe, B. L., Fong, S. K., Gerrard, L. A., Strachan, D. M., and Scheele, R. D. (2007). A glass-encapsulated calcium phosphate wasteform for the immobilization of actinide-fluoride-and chloride-containing radioactive wastes from the pyrochemical reprocessing of plutonium metal. J. Nucl. Mater. 361 (1), 78–93. doi:10.1016/j.jnucmat.2006.11.011
Donze, S., Montagne, L., Palavit, G., and Antonini, G. (2001). Structure and properties of phosphate glasses prepared from lead and cadmium chlorides. Phys. Chem. Glass 42 (2), 133–138.
Donze, S., Montagne, L., and Palavit, G. (2000). Thermal conversion of heavy metal chlorides (PbCl2, CdCl2) and alkaline chlorides (NaCl, KCl) into phosphate glasses. Chem. Mat. 12 (7), 1921–1925. doi:10.1021/cm991205d
Ebert, W. L., and Fortner, J. A. (2019b). Analyses of iron phosphate glasses for dehalogenated salt waste. ANL/CFCT-19/5. Lemont, IL: Argonne National Laboratory.
Ebert, W. L., and Fortner, J. A. (2020). Corrosion behavior of developmental iron phosphate glass waste forms. ANL/CFCT-20/1. Lemont, IL: Argonne National Laboratory.
Ebert, W. L., and Fortner, J. A. (2019a). Corrosion tests with developmental iron phosphate glass waste forms. ANL/CFCT-19/7. Lemont, IL: Argonne National Laboratory.
Ebert, W. L., Riley, B. J., Peterson, J. A., and Frank, S. M. (2018). Durability Testing of developmental iron phosphate waste Forms for echem salt waste. NTRD-MRWFD-2018-000513. Lemont, IL: Argonne National Laboratory.
Fedoroff, B. T., Sheffield, O. E., and Kaye, S. M. (1975). Encyclopedia of explosives and related items. New Jersey: Picatinny Arsenal.
Frank, S., Riley, B., and Ebert, W. L.Personal communication (2018). Status report on NH4Cl capture and conversion, milestone M3NT-18IN030105024. Idaho Falls, ID: Idaho National Laboratory.
Gregg, D. J., Vance, E. R., Dayal, P., Farzana, R., Aly, Z., Holmes, R., et al. (2020). Hot isostatically pressed (HIPed) fluorite glass-ceramic wasteforms for fluoride molten salt wastes. J. Am. Ceram. Soc. 103 (10), 5454–5469. doi:10.1111/jace.17293
Haefner, D. R., and Tranter, T. J. (2007). Methods of gas phase Capture of Iodine from fuel reprocessing off-gas: A literature survey. INL/EXT-07-12299. Idaho Falls, ID: Idaho National Laboratory.
Henderson, C. M. B., and Taylor, D. (1978). The thermal expansion of synthetic aluminosilicate-sodalites, M8(Al6Si6O24)X2. Phys. Chem. Min. 2, 337–347. doi:10.1007/bf00307576
Herrmann, S. D. (2017). Plan for ternary salt synthesis with Mark IV electrorefiner uranium dendrites in FCF. Idaho Falls, ID: Idaho National Laboratory.Personal communication
Hrma, P. R. (2010). Retention of Halogens in waste glass. PNNL-19361. Richland, WA: Pacific Northwest National Laboratory.
Jander, J. (1976). Recent chemistry and structure investigation of nitrogen triiodide, tribromide, trichloride, and related compounds. Adv. Inorg. Chem. Radiochem. 19, 1–63. doi:10.1016/S0065-2792(08)60070-9
Lavrinovich, Y. G., Kormilitsyn, M. V., Konovalov, V. I., Tselishchev, I. V., Tomilin, S. V., and Chistyakov, V. M. (2003). Vitrification of chloride wastes in the pyroelectrochemical method of reprocessing irradiated nuclear fuel. At. Energy 95 (5), 781–785. doi:10.1023/B:ATEN.0000016764.68862.5f
Lee, K. R., Riley, B. J., Park, H.-S., Choi, J.-H., Han, S. Y., Hur, J.-M., et al. (2019). Investigation of physical and chemical properties for upgraded SAP (SiO2-Al2O3-P2O5) waste form to immobilize radioactive waste salt. J. Nucl. Mater. 515, 382–391. doi:10.1016/j.jnucmat.2019.01.004
Lepry, W. C., Riley, B. J., Crum, J. V., Rodriguez, C. P., and Pierce, D. A. (2013). Solution-based approaches for making high-density sodalite waste forms to immobilize spent electrochemical salts. J. Nucl. Mater. 442 (1-3), 350–359. doi:10.1016/j.jnucmat.2013.08.033
Liu, J., Wang, F., Liao, Q., Zhu, H., Liu, D., and Zhu, Y. (2019). Synthesis and characterization of phosphate-based glass-ceramics for nuclear waste immobilization: Structure, thermal behavior, and chemical stability. J. Nucl. Mater. 513, 251–259. doi:10.1016/j.jnucmat.2018.11.002
Matyáš, R., and Pachman, J. (2013). “Nitrogen Halides,” in Primary Explosives (Berlin: Springer-Verlag) Chapter 11, 289–302.
McCarthy, G. J., White, W. B., and Pfoertsch, D. E. (1978). Synthesis of nuclear waste monazites, ideal actinide hosts for geologic disposal. Mat. Res. Bull. 13 (11), 1239–1245. doi:10.1016/0025-5408(78)90215-5
McFarlane, J., Taylor, P., Holcomb, D. E., and Poore, W. P. (2019). Review of hazards associated with molten salt reactor fuel processing operations. ORNL/TM-2019/1195. Oak Ridge, TN: Oak Ridge National Laboratory.
Meerkämper, B. (1954). Zum Verhalten des Jodstickstoffs beim Belichten mit Strahlung verschiedener Intensität. Z. Elektrochem. 58 (6), 387–416. doi:10.1002/bbpc.19540580607
Mesko, M., Day, D., and Bunker, B. (2000). Immobilization of CsCI and SrF2 in iron phosphate glass. Waste Manag. 20, 271–278. doi:10.1016/s0956-053x(99)00331-1
Metcalfe, B. L., and Donald, I. W. (2004). Candidate wasteforms for the immobilization of chloride-containing radioactive waste. J. Non. Cryst. Solids 348 (0), 225–229. doi:10.1016/j.jnoncrysol.2004.08.173
Metcalfe, B. L., Donald, I. W., Fong, S. K., Gerrard, L. A., Strachan, D. M., and Scheele, R. D. (2008). Calcium phosphate: A potential host for halide contaminated plutonium wastes. MRS Proc. 1124, 1124–Q0402. doi:10.1557/PROC-1124-Q04-02
Nakazawa, T., Kato, H., Okada, K., Ueta, S., and Mihara, M. (1999). Iodine immobilization by sodalite waste form. MRS Proc. 663, 51. doi:10.1557/proc-663-51
Nam, J., Chong, S., Riley, B. J., and McCloy, J. S. (2018). Iodosodalite waste forms from low-temperature aqueous process. MRS Adv. 3 (20), 1093–1103. doi:10.1557/adv.2018.225
Park, H.-S., Cho, I.-H., Eun, H. C., Kim, I.-T., Cho, Y. Z., and Lee, H.-S. (2011). Characteristics of wasteform composing of phosphate and silicate to immobilize radioactive waste salts. Environ. Sci. Technol. 45 (5), 1932–1939. doi:10.1021/es1029975
Park, H.-S., Kim, I.-T., Cho, Y.-Z., Eun, H.-C., and Kim, J.-H. (2007a). Characteristics of solidified products containing radioactive molten salt waste. Environ. Sci. Technol. 41 (21), 7536–7542. doi:10.1021/es0712524
Park, H.-S., Kim, I.-T., Cho, Y.-Z., Eun, H.-C., and Lee, H.-S. (2008). Stabilization/solidification of radioactive salt waste by using xSiO2-yAl2O3-zP2O5 (SAP) material at molten salt state. Environ. Sci. Technol. 42 (24), 9357–9362. doi:10.1021/es802012x
Park, H.-S., Kim, I.-T., Kim, H.-Y., Ryu, S.-K., and Kim, J.-H. (2007b). Stabilization/solidification of radioactive molten salt waste via gel-route pretreatment. Environ. Sci. Technol. 41 (4), 1345–1351. doi:10.1021/es0615472
Riley, B. J., Chong, S., and Lonergan, C. E. (2021a). Dechlorination apparatus for treating chloride salt wastes: System evaluation and scale-up. ACS Omega 6, 32239–32252. doi:10.1021/acsomega.1c05065
Riley, B. J. (2020). Electrochemical salt wasteform development: A review of salt treatment and immobilization options. Ind. Eng. Chem. Res. 59 (21), 9760–9774. doi:10.1021/acs.iecr.0c01357
Riley, B. J., McFarlane, J., DelCul, G. D., Vienna, J. D., Contescu, C. I., and Forsberg, C. W. (2019). Molten salt reactor waste and effluent management strategies: A review. Nucl. Eng. Des. 345, 94–109. doi:10.1016/j.nucengdes.2019.02.002
Riley, B. J., McFarlane, J., DelCul, G. D., Vienna, J. D., Contescu, C. I., Hay, L. M., et al. (2018). Identification of potential waste processing and waste form options for molten salt reactors. NTRD-MSR-2018-000379, PNNL-27723. Richland, WA: Pacific Northwest National Laboratory.
Riley, B. J., Peterson, J. A., Vienna, J. D., Ebert, W. L., and Frank, S. M. (2020). Dehalogenation of electrochemical processing salt simulants with ammonium phosphates and immobilization of salt cations in an iron phosphate glass waste form. J. Nucl. Mater. 529, 151949. doi:10.1016/j.jnucmat.2019.151949
Riley, B. J., Rieck, B. T., McCloy, J. S., Crum, J. V., Sundaram, S. K., and Vienna, J. D. (2012). Tellurite glass as a waste form for mixed alkali-chloride waste streams: Candidate materials selection and initial testing. J. Nucl. Mater. 424 (1-3), 29–37. doi:10.1016/j.jnucmat.2012.01.024
Riley, B. J., Schweiger, M. J., Kim, D.-S., Lukens, W. W., Williams, B. D., Iovin, C., et al. (2014). Iodine solubility in a low-activity waste borosilicate glass at 1000 °C. J. Nucl. Mater. 452 (1-3), 178–188. doi:10.1016/j.jnucmat.2014.04.027
Riley, B. J., Vienna, J. D., and Ebert, W. L. (2021b). Road map for developing iron phosphate waste forms for salt wastes. PNNL-30998, ANL/CFCT-20/44. Richland, WA: Pacific Northwest National Laboratory.
Riley, B. J., Vienna, J. D., Frank, S. M., Kroll, J. O., Peterson, J. A., Canfield, N. L., et al. (2017). Glass binder development for a glass-bonded sodalite ceramic waste form. J. Nucl. Mater. 489, 42–63. doi:10.1016/j.jnucmat.2017.03.041
Riley, B. J., Vienna, J. D., Strachan, D. M., McCloy, J. S., and Jerden, J. L. (2016). Materials and processes for the effective capture and immobilization of radioiodine: A review. J. Nucl. Mater. 470, 307–326. doi:10.1016/j.jnucmat.2015.11.038
Sato, F., Myochin, M., Terunuma, H., and Arai, O. (2002). “Examination of reaction conditions for oxidation of salt waste,” in Proceedings of Japan Atomic Energy Research Inst Conf, Tokai, Ibaraki, 31 Oct - 2 Nov 2001, 527–531.
Siemer, D. D. (2013a). “A practical solution to Hanford's tank waste problem,” in Proceedings of GLOBAL - International Nuclear Fuel Cycle Conference - Nuclear Energy at a Crossroads, Salt Lake City, Utah, September 29-October 3, 2013, 319–324.
Siemer, D. D. (2012). Improving the integral fast reactor's proposed salt waste management system. Nucl. Technol. 178 (3), 341–352. doi:10.13182/nt12-a13599
Siemer, D. D. (2013b). “Vitrification of IFR and MSBR halide salt reprocessing wastes,” in Proceedings of GLOBAL - International Nuclear Fuel Cycle Conference - Nuclear Energy at a Crossroads, Salt Lake City, Utah, September 29-October 3, 2013, 475–482.
Stariha, S. A., and Ebert, W. L. (2020). Corrosion behavior of developmental iron phosphate glass waste forms: FY20 status report. ANL/CFCT-20/34. Lemont, IL: Argonne National Laboratory.
Stariha, S. A., and Ebert, W. L. (2021). Corrosion behavior of developmental iron phosphate waste forms: FY21 status report. ANL/CFCT-21/14. Lemont, IL: Argonne National Laboratory.
Stefanovsky, S. V., Myasoedov, B. F., Remizov, M. B., and Belanova, E. A. (2014). IR and Raman spectroscopy of sodium-aluminophosphate glasses for immobilizing high-level wastes from spent nuclear fuel reprocessing. J. Appl. Spectrosc. 81 (4), 618–623. doi:10.1007/s10812-014-9979-5
Stefanovsky, S. V., Stefanovsky, O. I., Remizov, M. B., Kozlov, P. V., Belanova, E. A., Makarovsky, R. A., et al. (2017). Sodium–aluminum–iron phosphate glasses as legacy high level waste forms. Prog. Nucl. Energy 94, 229–234. doi:10.1016/j.pnucene.2016.01.022
Strachan, D. M., and Babad, H. (1979). Iodide and iodate sodalites for long-term storage of I-129. Am. Ceram. Soc. Bull. 58 (3), 327.
Tsai, W.-T. (2008). Environmental and health risk analysis of nitrogen trifluoride (NF3), a toxic and potent greenhouse gas. J. Hazard. Mat. 159 (2), 257–263. doi:10.1016/j.jhazmat.2008.02.023
Vance, E. R., Davis, J., Olufson, K., Chironi, I., Karatchevtseva, I., and Farnan, I. (2012). Candidate waste forms for immobilisation of waste chloride salt from pyroprocessing of spent nuclear fuel. J. Nucl. Mater. 420 (1-3), 396–404. doi:10.1016/j.jnucmat.2011.09.020
Wang, D., Li, H., Wang, Z., Wu, X., Sun, Y., Huang, X., et al. (2004). New solid-state synthesis routine and mechanism for LiFePO4 using LiF as lithium precursor. J. Solid State Chem. 177 (12), 4582–4587. doi:10.1016/j.jssc.2004.09.013
Wang, F., Liu, J., Wang, Y., Liao, Q., Zhu, H., Li, L., et al. (2020). Synthesis and characterization of iron phosphate based glass-ceramics containing sodium zirconium phosphate phase for nuclear waste immobilization. J. Nucl. Mater. 531, 151988. doi:10.1016/j.jnucmat.2020.151988
Wasnik, M. S., Grant, A. K., Carlson, K., and Simpson, M. F. (2019a). Dechlorination of molten chloride waste salt from electrorefining via ion-exchange using pelletized ultra-stable H-Y zeolite in a fluidized particle reactor. J. Radioanal. Nucl. Chem. 320 (2), 309–322. doi:10.1007/s10967-019-06476-y
Wasnik, M. S., Livingston, T. C., Carlson, K., and Simpson, M. F. (2019b). Kinetics of dechlorination of molten chloride salt using protonated ultrastable Y zeolite. Ind. Eng. Chem. Res. 58 (33), 15142–15150. doi:10.1021/acs.iecr.9b02577
Weller, M. T., and Wong, G. (1989). Mixed halide sodalites. Eur. J. Solid State Inorg. Chem. 26 (6), 619–633.
Xiang, X., Lin, H., Li, R., Cheng, Y., Huang, Q., Xu, J., et al. (2019). Stress-induced CsPbBr3 nanocrystallization on glass surface: Unexpected mechanoluminescence and applications. Nano Res. 12 (5), 1049–1054. doi:10.1007/s12274-019-2338-3
Yu, X., Day, D. E., Long, G. J., and Brow, R. K. (1997). Properties and structure of sodium-iron phosphate glasses. J. Non. Cryst. Solids 215 (1), 21–31. doi:10.1016/S0022-3093(97)00022-7
Keywords: dehalogenation, hydrogen halides, ammonium halides, nitrogen trihalides, ammonium triiodide, molten salt reactors, electrochemical reprocessing
Citation: Riley BJ and Chong S (2022) Dehalogenation reactions between halide salts and phosphate compounds. Front. Chem. 10:976781. doi: 10.3389/fchem.2022.976781
Received: 23 June 2022; Accepted: 01 August 2022;
Published: 07 September 2022.
Edited by:
Feng Luo, East China University of Technology, ChinaReviewed by:
Anna Shelyug, Institute of Solid State Chemistry, RussiaCopyright © 2022 Riley and Chong. This is an open-access article distributed under the terms of the Creative Commons Attribution License (CC BY). The use, distribution or reproduction in other forums is permitted, provided the original author(s) and the copyright owner(s) are credited and that the original publication in this journal is cited, in accordance with accepted academic practice. No use, distribution or reproduction is permitted which does not comply with these terms.
*Correspondence: Brian J. Riley, YnJpYW4ucmlsZXlAcG5ubC5nb3Y=
Disclaimer: All claims expressed in this article are solely those of the authors and do not necessarily represent those of their affiliated organizations, or those of the publisher, the editors and the reviewers. Any product that may be evaluated in this article or claim that may be made by its manufacturer is not guaranteed or endorsed by the publisher.
Research integrity at Frontiers
Learn more about the work of our research integrity team to safeguard the quality of each article we publish.