- College of Chemistry and Materials Engineering, Wenzhou University, Wenzhou, China
A new dysprosium (III) coordination polymer [Dy(Hm-dobdc) (H2O)2]·H2O (Dy-CP), was hydrothermal synthesized based on 4,6-dioxido-1,3-benzenedicarboxylate (H4m-dobdc) ligand containing carboxyl and phenolic hydroxyl groups. The Dy(III) center adopts an octa-coordinated [DyO8] geometry, which can be described as a twisted square antiprism (D4d symmetry). Neighboring Dy(III) ions are interconnected by deprotonated Hm-dobdc3− ligand to form the two-dimensional infinite layers, which are further linked to generate three-dimensional structure through abundant hydrogen bonds mediated primarily by coordinated and lattice H2O molecules. Magnetic studies demonstrates that Dy-CP shows the field-induced slow relaxation of magnetization and the energy barrier Ueff/kB and relaxation time τ0 are 35.3 K and 1.31 × 10–6 s, respectively. Following the vehicular mechanism, Dy-CP displays proton conductivity with σ equal to 7.77 × 10–8 S cm−1 at 353 K and 30%RH. Moreover, luminescence spectra reveal that H4m-dobdc can sensitize characteristic luminescence of Dy(III) ion. Herein, good magnetism, proton conduction, and luminescence are simultaneously achieved, and thus, Dy-CP is a potential multifunctional coordination polymer material.
Introduction
Coordination polymers (CPs) have potential applications in gas storage/separation, catalysis, magnetism, and proton conduction due to their customizable compositions and variable structures (Yaghi et al., 2003; Kitagawa et al., 2004; Ferey, 2008; Zhu et al., 2018; Yuan et al., 2020; Cai et al., 2021; Chakraborty et al., 2021). In particular, CPs can integrate these multiple properties into the same molecular composite, which is an excellent platform for designing advanced multifunctional materials (Wang et al., 2017; Ge et al., 2019b; Ye et al., 2020; Zhou et al., 2020; Fan et al., 2021). In the field of molecular magnetism, Ln-CPs are of particular interest, enabling the production of magnetic materials with diverse properties, such as single-molecule magnets (SMMs) (Baldovi et al., 2014; Liu et al., 2016; Zhong et al., 2022). As we know, the magnetic anisotropy of metal ions plays a very important role in the construction of SMMs (Woodruff et al., 2013; Zhu et al., 2021). In this regard, lanthanide Dy(III) ion, may carry significant anisotropy because of its intrinsically large and unquenched orbital contribution to the magnetic moment (Ding et al., 2018; Parmar et al., 2021; Li et al., 2022). Goodwin and co-workers made a breakthrough in Dy(III)-based SMMs, reporting compound that exhibits a high effective energy barrier of 1,760 K (Goodwin et al., 2017). Therefore, we consider Dy(III) coordination compounds to be promising candidates for designing high-performance SMMs. Moreover, the high coordination number and flexible coordination geometry of Dy(III) ions can produce various interesting frameworks. Up to now, multifarious Dy-CPs with slow magnetic relaxation behaviors have been developed (Wu et al., 2020; Song et al., 2021; Su et al., 2021). Nevertheless, the inherent magnetisms of Dy(III) ions are very sensitive to various factors such as coordination geometry, magnetic interactions, etc., making the performance of Dy(III)-based SMMs difficult to predict (Pinkowicz et al., 2015; Zhang et al., 2015; Ge et al., 2020). More new topologies need to be established to study the magneto-structural correlations in depth.
Developing multifunctional magnetic CPs is currently a very attractive research topic, where magnetism can be integrated with other properties (such as proton conduction, sensing, or luminescence) to achieve multi-task expression and expand the application range of materials (Chen et al., 2017; Bera et al., 2018; Minguez Espallargas and Coronado, 2018). Among them, proton-conducting materials are potential replacements for Nafion ionomers in the catalyst layer of fuel cells, which can produce environmentally friendly energy (Yamada et al., 2013; Ramaswamy et al., 2014; Meng et al., 2017; Li et al., 2020). The easily tunable crystal structures and modifiable pore environment of CPs are ideal crystal models for designing proton conductors and gaining insight into proton transfer mechanisms (Su et al., 2020). Studies have shown that designing and developing complex hydrogen bond networks is one of the efficient strategies to improve proton conductivity in CPs, such as introducing functional Brønsted acid groups (-COOH and -OH) (Biswas et al., 2017; Bera et al., 2018).
Based on the above considerations, we envisioned that combining Dy(III) ion with carboxyl- and hydroxyl-rich organic ligand would be a sensible strategy to engineer SMM behavior with proton conduction into functional CPs. We chose the aromatic ligand 4,6-dioxido-1,3-benzenedicarboxylate (H4m-dobdc), and to our knowledge, Dy(III) complexes based on this ligand have been not been reported (Kapelewski et al., 2018; Barnett et al., 2019). Carboxyl and phenolic hydroxyl groups have high affinity with Dy(III) ion and diverse coordination modes, and more importantly, they can also act as efficient hydrogen bond acceptors/donors, forming infinite hydrogen bond networks to facilitate proton transport (Li et al., 2017; Wang et al., 2021; Bhadra et al., 2022). Herein, a two-dimensional (2D) CP [Dy(Hm-dobdc) (H2O)2]·H2O (Dy-CP), was hydrothermal synthesized through the interaction of Dy(III) ion and judiciously selected organic ligands, and its field-induced slow relaxation behavior and proton conduction properties were demonstrated.
Experimental sections
Synthesis of [Dy(Hm-dobdc) (H2O)2]·H2O (Dy-CP)
The reactants H4m-dobdc (0.0297 g, 0.15 mmol), Dy(NO3)3·6H2O (0.0918 g, 0.2 mmol), and 10 ml H2O were placed in a 15 ml Teflon cup. The mixture was heated to 140°C for 3 days. After cooling, the light brown block crystals of Dy-CP were obtained with a yield of 24% (based on H4m-dobdc). Anal. Calcd (%): C, 23.34; H, 2.20%. Found: C, 23.18; H, 2.14%. IR (cm−1, KBr): 3,859.56(s), 3,468.01(w), 3,217.27(w), 2,657.91(w), 1,853.59(w), 1,811.16(w), 1,780.3(w), 1,720.5(s), 1,705.07(s), 1,643.35(s), 1,566.2(m), 1,519.91(w), 1,465.9(m), 1,400.32(s), 1,346.31(m), 1,301.95(m), 1,226.73(w), 1,195.87(s), 1,083.99(w), 956.69(w), 893.04(m), 844.82(m), 819.75(w), 783.1(w), 754.17(w), 723.31(m), 700.16(m), 677.01(w), 653.87(m), 619.15(m), 578.64(w), 526.57(w), 472.56(m), 430.13(w).
Result and disscussion
Description of crystal structure
The brown-orange block crystals of [Dy(Hm-dobdc) (H2O)2]·H2O (Dy-CP) were obtained by the reaction of H4m-dobdc and Dy(NO3)3·6H2O at 140°C. Single-crystal analysis shows that Dy-CP crystallizes in the monoclinic space group P21/n, and the crystallographic data are summarized in Supplementary Table S1. Its asymmetric unit involves one Dy(III) ion, one Hm-dobdc3− ligand, two coordinated H2O molecules, and one uncoordinated H2O molecule (Figure 1A). The Dy1 center adopts an octa-coordinated [DyO8] geometry with four Ocarboxylate atoms (O1, O2, O5, and O6) from three Hm-dobdc3− ligands, the another Ocarboxylate atom (O1) and one Ophenoxide atom (O3) from one Hm-dobdc3− ligand, and two Owater atom (O7 and O8) from two coordinated H2O (Figure 1B and Supplementary Figure S1). Dy−O bond lengths are in the range of 2.287 (3) Å to 2.495 (3) Å, similar to those reported for Dy(III) compounds with oxygen donors (Supplementary Table S2) (Song et al., 2021). Shape analysis revealed that the exact geometry of Dy1 ion can be assigned to a twisted square antiprism (D4d symmetry) with a SAPR-8 factor of 1.244 (Supplementary Table S3) (Ge et al., 2017). As shown in Figure 1B, the Dy1 ion is unevenly distributed between two square planes. The distance of Dy1 ion from the center of the top plane (O1, O3, O6 and O8) is 1.225 Å, which is closer than the distance (1.415 Å) to the center of the bottom plane (O1, O2, O5 and O7). The dihedral angle between these two planes is 5.157°, and the bending angle α defined as center-Dy1-center is 171.45°.
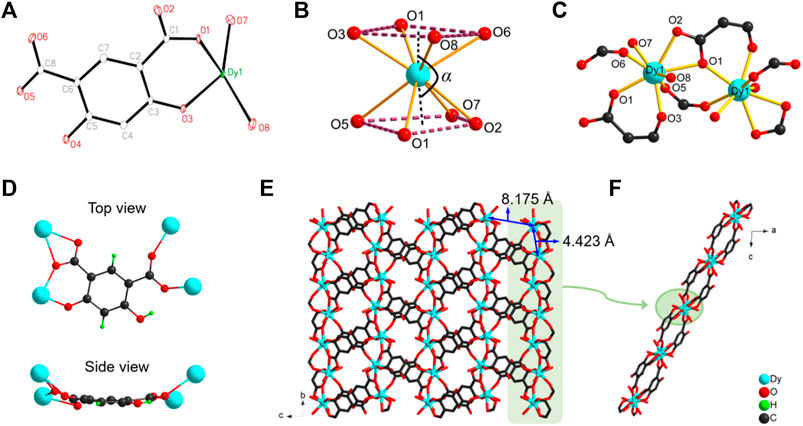
FIGURE 1. (A) The labeled asymmetric unit of Dy-CP (B) coordination sphere of Dy1 center (C) connection between adjacent metal centers (D) top view and side view of coordination mode of Hm-dobdc3− linker (E) 2D structure of Dy-CP in the bc plane (F) side view of the 2D layer. Uncoordinated H2O molecules are removed for clarity.
One Hm-dobdc3− ligand is coordinated to four Dy1 ions via one deprotonated phenolic hydroxyl group and two deprotonated carboxyl groups. Of the two carboxyl groups, one is ligated in a μ2-η2:η1 chelating mode and the other in a μ2-η1:η1 mode (Figure 1D). After coordination, the Hm-dobdc3− is not planar viewed from the side. The multiple coordination sites and variable coordination configuration of Hm-dobdc3− play a key role in constructing Dy-CP. The adjacent Dy1 ions are linked together by one μ2-η1:η1 carboxylate group and one μ2-O1 atom from Hm-dobdc3− to generate the one-dimensional metal chain along the crystallographic b-axis (Figures 1C–E). The nearest Dy···Dy separation is 4.423 Å and Dy1-O1-Dy1 angle is 132.85°. Each chain is linked by the polytopic Hm-dobdc3− ligand (Dy···Dy = 8.175 Å) generating the 2D infinite layer (Figures 1E,F).
In the stacking motif, these 2D layers are stacked along the crystallographic a-axis in an–AAA–fashion, generating small-sized pores (Figure 2A). Furthermore, uncoordinated phenolic hydroxyl group is oriented towards the interior of the pore to create a targeted hydrophilic environment in which the uncoordinated H2O molecules reside. Abundant O−H···O hydrogen bonds are formed between the lattice H2O molecule, coordinated H2O molecule and the Hm-dobdc3− ligand (Supplementary Table S4) (Wang et al., 2009). One coordinated H2O molecule (O8) and one lattice H2O molecule (O9) and their symmetry-related counterparts yield a centrosymmetric cyclic H2O tetramer (Supplementary Figure S2). In the tetramer, the O8 water monomer is the hydrogen bond donor and the O9 atom acts as the acceptor. The average distance of O···O is only 2.727 Å. This hydrogen-bonding network is beneficial for stabilizing H2O molecules (Sasaki et al., 2018). The tetramers link the adjacent layers to generate a 3D framework (Figures 2B,C). The hydrophilicity and multiple hydrogen bonds facilitate the exploration of proton conduction (Meng et al., 2017).
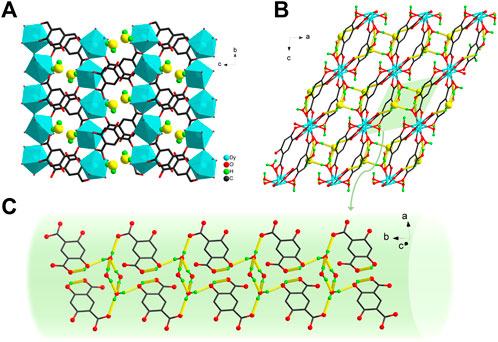
FIGURE 2. (A) The stacking motif of Dy-CP along the crystallographic a-axis. Yellow balls represent the O atoms of lattice H2O molecules (B) 3D framework driven by the O−H···O hydrogen bonds (yellow dashed lines) (C) an enlarged view of the hydrogen bonds formed between lattice H2O molecule, coordinated H2O molecule and Hm-dobdc3− ligands. Some unrelated atoms are omitted for clarity.
FT-IR spectra, purity and structural stability
The FTIR spectra of H4m-dobdc and Dy-CP are shown in Supplementary Figure S3. Both samples contain a broad -OH stretching vibration absorption band around 3,200 cm−1. Compared with the free ligand, the shift of the characteristic peaks for the symmetric and asymmetric stretching of the carboxyl groups in Dy-CP suggests that H4m-dobdc reacts with Dy(III) site. The enhanced absorption band in the 3,300–3,700 cm−1 region in Dy-CP indicates the presence of H2O molecules directly coordinating to the Dy(III) sites and/or generating hydrogen bonds (Vitillo and Ricchiardi, 2017). Thermogravimetric analysis (TGA) curve reveals that the lattice H2O molecule can be stored in the pore of Dy-CP at room temperature and higher, with release occurring around 90–192°C (weight loss of 4.40%, calculated 4.37%, Supplementary Figure S4). Moreover, maintaining better stability in aqueous solution is a prerequisite for CPs to be used as proton-conducting materials (Yuan et al., 2018; Su et al., 2020; Yang et al., 2021). Powder X-ray diffraction (PXRD) measurement confirms the absence of any other phases in Dy-CP, with the experimental diffraction peak positions consistent with that simulated using crystal data (Figure 3). The synthesized samples were immersed in water and boiling water for several days. PXRD profiles of all water-soaked samples are in good agreement with the pristine one, indicating the retained crystallinity of Dy-CP in water (Figure 3). The good stability in water will provide new opportunity for proton conduction.
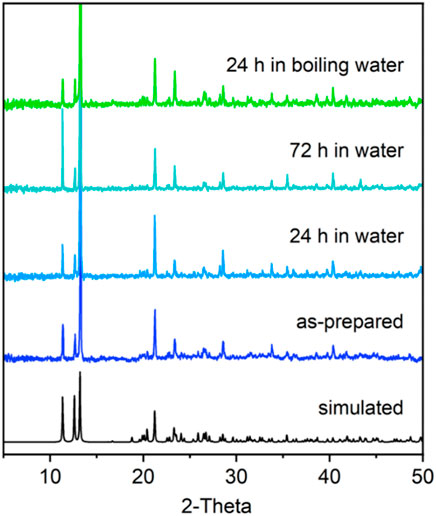
FIGURE 3. PXRD patterns of synthesized Dy-CP (5–50°) sample and those were treated with water for several days.
Magnetic properties
The direct-current (DC) magnetic susceptibilities experiments were carried out on polycrystalline samples of Dy-CP in an applied field of 1 kOe between 2 and 300 K (Figure 4). The χMT value at 300 K is 14.56 cm3 K mol−1, which is a little higher than the theoretical value for a free Dy(III) ion (14.17 cm3 K mol−1; g = 4/3, J = 15/2) (Cui et al., 2021). With a lowering of the temperature from 300 to 10 K, the χMT value decreases gradually, and then drops rapidly to the minima of 7.84 cm3 K mol−1 at 2 K, which may be caused by the antiferromagnetic interactions between adjacent Dy(III) ions and/or the progressive depopulation of the excited Stark sublevels of Dy(III) ions (Wu et al., 2020). Considering the slightly longer Dy⋅⋅⋅Dy distance compared to the literature reports, antiferromagnetic interaction maybe not dominate in Dy-CP.
The field-dependent magnetization (M) of Dy-CP was also collected in the field (H) range of 0–70 kOe at 2.0, 3.0 and 5.0 K, respectively (Figure 4 inset). The M value of Dy-CP increases slowly as H increases, and a maximum of 5.84 Nβ is reached at 70 kOe and 2.0 K. The nonsaturation of M and the non-superimposed isothermal magnetization curves (M vs. H T−1) suggest the presence of low-lying excited states and/or significant magnetic anisotropy in Dy-CP (Ge et al., 2019a; Cui et al., 2021).
Considering the magnetic anisotropy of Dy(III) ion, the alternating-current (ac) magnetic susceptibilities of Dy-CP were measured to explore the dynamic magnetic behavior. Under zero dc field, the out-of-phase (χ″) signals keep silent at high frequency of 707 Hz (Supplementary Figure S5). When an additional 1.5 kOe dc field is applied, the good-shaped peaks can be easily observed in the χ″ vs. T graph (Figure 5). The peak position of the χ"(T) signal shifts gradually to the high temperature component as the frequency increases, showing the obvious slow magnetic relaxation expected for SMMs (Chen et al., 2016). At 999 Hz, the maximum value of χ"(T) appears around 5.5 K. The relaxation time τ was extracted from the peaks of χ″ signals in Figure 5A. At the high temperature, τ is linearly dependent on T−1, which can be fitted using Arrhenius law to afford the thermal energy barrier (Ueff/kB) and the pre-exponential factor (τ0) are 30.3 K and 6.82 × 10–7 s, respectively (Figure 6), confirming a field-induced SMM performance (10–6–10–11 s) (Bera et al., 2018; Bera et al., 2019). At the lower temperature, the relationship between ln τ and T−1 deviates from the linearity of Arrhenius law, suggesting the intervention of other possible relaxation processes.
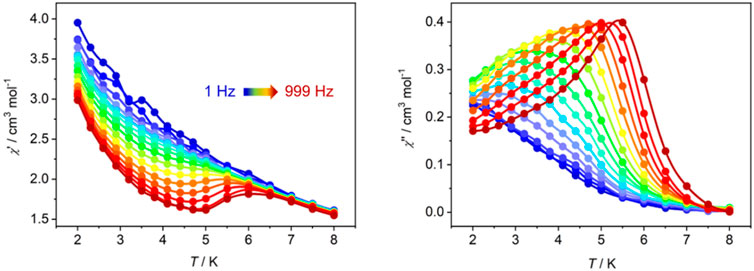
FIGURE 5. Temperature-dependent χ′ (left) and χ″ (right) ac susceptibilities for Dy-CP measured in 1.5 kOe dc field.
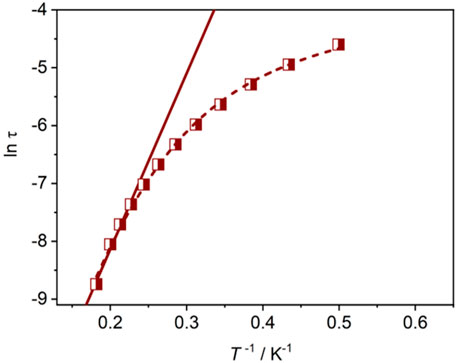
FIGURE 6. ln τ vs. T−1 plot for Dy-CP and the Arrhenius law τ−1 = τ0−1 exp (-Ueff/kBT) (solid) and Equation τ−1 = AH2T + CTn + τ0−1exp (-Ueff/kBT) (dashed) fitting lines.
For further investigation of the magnetic dynamics, the frequency-dependent ac susceptibilities were also collected. χ"(F) peaks can be observed clearly in the high frequency region, as shown in Supplementary Figure S6. Slightly higher χ" values in the low frequency region, especially at lower temperatures, suggest that other relaxation processes may exist (Gonzalez et al., 2021). Its Cole−Cole diagrams exhibit semicircular shape in the high frequency and irregular shape in the low frequency regions. Fitting the data between 2.3 and 5.5 K by the extended Debye functions (Gao et al., 2018) gives α value ranging from 0.05 to 0.16 for the relaxation in high frequency region (Supplementary Figure S7). Unfortunately, the fit for the low frequency region is unsuccessful due to limited frequency and/or temperature. The fit of τ takes into account the multiple relaxation processes reveals that the relaxation occurs via the temperature-dependent Orbach (τ0−1exp (-Ueff/kBT)), Raman (CTn), and direct (AH2T) mechanisms (Figure 6 short dashed line). Parameters A = 1.93 × 10–5 s−2 Oe−2, n = 5.27, C = 0.50 s−1 K−5.27, Ueff/kB = 35.3 K, and τ0 = 1.31 × 10–6 s were obtained, which are consistent with the expectations of Kramer ion Dy(III)-based SMMs (Ge et al., 2017).
Proton conduction
The presence of an intricate network of hydrogen bonds in Dy-CP suggests the efficient proton transfer pathways. Subsequent ac impedance of the compact pellet was measured under controlled experimental condition, and the proton conductivities (σ) were calculated by fitting the Nyquist plots. At 303 K and 30% relative humidity (RH), the Nyquist plot displays a partial semicircle in the high frequency component and a small oblique tail in the low frequency component, which is the fingerprint of proton transport behavior (Elahi et al., 2019). The related σ is 4.37 × 10–10 S cm−1 (Figure 7). Further studies found that the proton conductivity of Dy-CP is temperature dependent. As temperature increases, the size of the semicircle appears to decrease significantly, corresponding to the enhanced conductivity. At 353 K, σ reaches 7.77 × 10–8 S cm−1. This trend in conductivity can be explained by several plausible reasons, (i) the pKw values of the coordinated and lattice H2O molecules decrease, favoring the release of proton; (ii) the stable existence of lattice H2O molecules at elevated temperature, facilitating the preservation of strong hydrogen bonds and (iii) thermally assisted proton hopping on hydrogen-bonding array containing H2O molecules (Tang et al., 2014; Bera et al., 2018). According to the linear fit of Arrhenius law σT = σ0 exp (Ea/kBT), the activation energy Ea = 0.93 eV is estimated (Supplementary Figure S8). Value more than 0.4 eV indicates that a vehicular mechanism operates for proton conduction in Dy-CP (Su et al., 2020). Additionally, the structural of Dy-CP was integrated after the impedance measurement, as PXRD pattern demonstrated (Supplementary Figure S9).
Luminescence property
The solid-state luminescence property of Dy-CP was measured at room temperature. When excited at 329 nm, Dy-CP exhibits two emission peaks at 481 and 475 nm, corresponding to hypersensitive 4F9/2–6H15/2 and 4F9/2–6H13/2 transitions of Dy(III) ion (Supplementary Figure S10). Notably, the disappearance of the broadband emission of the ligand implies an effective energy transfer from the ligand to the metal, and H4m-dobdc ligand brings an efficient antenna effect (Zhong et al., 2020).
Conclusion
In summary, a two-dimensional coordination polymer [Dy(Hm-dobdc) (H2O)2]·H2O (Dy-CP) containing abundant hydrogen bonds has been successfully prepared and structurally characterized. Magnetic investigation demonstrates that Dy-CP exhibits the field-induced SMM property with the energy barrier equal to 35.3 K. The impedance analysis of Dy-CP displays proton conductivity (7.77 × 10–8 S cm−1 at 353 K) at 30% RH. Furthermore, luminescence spectra reveal that H4m-dobdc can sensitize characteristic luminescence of Dy(III) ion at 481 and 475 nm. This phenomenon suggests that introducing Dy(III) ion and functional carboxyl and phenolic hydroxyl groups is beneficial for the development of multifunctional coordination polymers possessing luminescence, proton conduction, and magnetism.
Data availability statement
The datasets presented in this study can be found in online repositories. The names of the repository/repositories and accession number(s) can be found in the article/Supplementary Material.
Author contributions
Original idea was conceived by J-FC, H-PX, XL, and J-YG; experiments and data analysis were performed by J-FC, Y-LG, D-HW, H-TC, and Z-LM.; structure characterization was performed by J-FC, H-TC, H-PX, and J-YG; manuscript was drafted J-FC, H-PX, and J-YG. All authors have given approval to the manuscript.
Funding
This work was supported by the Natural Science Foundation of Zhejiang Province (No. LY20B010003), and the National Natural Science Foundation of China (No. 21801054).
Conflict of interest
The authors declare that the research was conducted in the absence of any commercial or financial relationships that could be construed as a potential conflict of interest.
Publisher’s note
All claims expressed in this article are solely those of the authors and do not necessarily represent those of their affiliated organizations, or those of the publisher, the editors and the reviewers. Any product that may be evaluated in this article, or claim that may be made by its manufacturer, is not guaranteed or endorsed by the publisher.
Supplementary material
The Supplementary Material for this article can be found online at: https://www.frontiersin.org/articles/10.3389/fchem.2022.974914/full#supplementary-material
References
Baldovi, J. J., Coronado, E., Gaita-Arino, A., Gamer, C., Gimenez-Marques, M., and Minguez Espallargas, G. (2014). A SIM-MOF: Three-dimensional organisation of single-ion magnets with anion-exchange capabilities. Chem. Eur. J. 20 (34), 10695–10702. doi:10.1002/chem.201402255
Barnett, B. R., Parker, S. T., Paley, M. V., Gonzalez, M. I., Biggins, N., Oktawiec, J., et al. (2019). Thermodynamic separation of 1-butene from 2-butene in metal-organic frameworks with open metal sites. J. Am. Chem. Soc. 141 (45), 18325–18333. doi:10.1021/jacs.9b09942
Bera, S. P., Mondal, A., and Konar, S. (2019). Lanthanide-based layer-type two-dimensional coordination polymers featuring slow magnetic relaxation, magnetocaloric effect and proton conductivity. Chem. Asian J. 14 (20), 3702–3711. doi:10.1002/asia.201900842
Bera, S. P., Mondal, A., Roy, S., Dey, B., Santra, A., and Konar, S. (2018). 3D isomorphous lanthanide coordination polymers displaying magnetic refrigeration, slow magnetic relaxation and tunable proton conduction. Dalton Trans. 47 (43), 15405–15415. doi:10.1039/c8dt03498b
Bhadra, B. N., Ahmed, I., Lee, H. J., and Jhung, S. H. (2022). Metal-organic frameworks bearing free carboxylic acids: Preparation, modification, and applications. Coord. Chem. Rev. 450, 214237. doi:10.1016/j.ccr.2021.214237
Biswas, S., Chakraborty, J., Singh Parmar, V., Bera, S. P., Ganguli, N., and Konar, S. (2017). Channel-assisted proton conduction behavior in hydroxyl-rich lanthanide-based magnetic metal-organic frameworks. Inorg. Chem. 56 (9), 4956–4965. doi:10.1021/acs.inorgchem.6b03147
Cai, G., Yan, P., Zhang, L., Zhou, H. C., and Jiang, H. L. (2021). Metal-organic framework-based hierarchically porous materials: Synthesis and applications. Chem. Rev. 121 (20), 12278–12326. doi:10.1021/acs.chemrev.1c00243
Chakraborty, G., Park, I. H., Medishetty, R., and Vittal, J. J. (2021). Two-dimensional metal-organic framework materials: Synthesis, structures, properties and applications. Chem. Rev. 121 (7), 3751–3891. doi:10.1021/acs.chemrev.0c01049
Chen, Q., Li, J., Meng, Y. S., Sun, H. L., Zhang, Y. Q., Sun, J. L., et al. (2016). Tuning slow magnetic relaxation in a two-dimensional dysprosium layer compound through guest molecules. Inorg. Chem. 55 (16), 7980–7987. doi:10.1021/acs.inorgchem.6b01014
Chen, Y. C., Liu, J. L., Lan, Y., Zhong, Z. Q., Mansikkamaki, A., Ungur, L., et al. (2017). Dynamic magnetic and optical insight into a high performance pentagonal bipyramidal Dy(III) single-ion magnet. Chem. Eur. J. 23 (24), 5708–5715. doi:10.1002/chem.201606029
Cui, M., Yang, L., Li, F., Zhou, L., Song, Y., Fang, S. M., et al. (2021). Multifunctional Dy(III) enantiomeric pairs showing enhanced photoluminescences and third-harmonic generation responses through the coordination role of homochiral tridentate N, N, N-pincer ligands. Inorg. Chem. 60 (17), 13366–13375. doi:10.1021/acs.inorgchem.1c01682
Ding, Y. S., Yu, K. X., Reta, D., Ortu, F., Winpenny, R. E. P., Zheng, Y. Z., et al. (2018). Field- and temperature-dependent quantum tunnelling of the magnetisation in a large barrier single-molecule magnet. Nat. Commun. 9 (1), 3134. doi:10.1038/s41467-018-05587-6
Elahi, S. M., Lai, Q. H., Ren, M., Bao, S. S., Kurmoo, M., and Zheng, L. M. (2019). Two- and three-dimensional heterometallic ln[Ru2-α-Ammonium diphosphonate] nets: Structures, porosity, magnetism, and proton conductivity. Inorg. Chem. 58 (20), 14034–14045. doi:10.1021/acs.inorgchem.9b02026
Fan, L., Zhao, D., Zhang, H., Wang, F., Li, B., Yang, L., et al. (2021). A hydrolytically stable amino-functionalized Zinc(II) metal-organic framework containing nanocages for selective gas adsorption and luminescent sensing. Microporous Mesoporous Mat. 326, 111396. doi:10.1016/j.micromeso.2021.111396
Ferey, G. (2008). Hybrid porous solids: Past, present, future. Chem. Soc. Rev. 37 (1), 191–214. doi:10.1039/b618320b
Gao, F., Zhang, Y. Q., Sun, W., Liu, H., and Chen, X. (2018). Syntheses, structures and magnetic properties of macrocyclic Schiff base-supported homodinuclear lanthanide complexes. Dalton Trans. 47 (33), 11696–11704. doi:10.1039/c8dt02243g
Ge, J. Y., Chen, Z., Qiu, Y. R., Huo, D., Zhang, Y. Q., Wang, P., et al. (2019a). Modulating magnetic property of phthalocyanine supported M(II)-Dy(III) (M = Ni, Zn) heterodinuclear complexes. Inorg. Chem. 58 (14), 9387–9396. doi:10.1021/acs.inorgchem.9b01179
Ge, J. Y., Chen, Z., Zhang, L., Liang, X., Su, J., Kurmoo, M., et al. (2019b). A two-dimensional iron(II) coordination polymer with synergetic spin-crossover and luminescent properties. Angew. Chem. Int. Ed. 58 (26), 8789–8793. doi:10.1002/anie.201903281
Ge, J. Y., Cui, L., Li, J., Yu, F., Song, Y., Zhang, Y. Q., et al. (2017). Modulating single-molecule magnetic behavior of a dinuclear erbium(III) complex by solvent exchange. Inorg. Chem. 56 (1), 336–343. doi:10.1021/acs.inorgchem.6b02243
Ge, J. Y., Qiu, Y. R., Wang, H. Y., Su, J., Wang, P., and Chen, Z. (2020). Magnetic relaxation dynamics of a binuclear diluted Er(III)/Y(III) compound influenced by lattice solvent. Chem. Asian J. 15 (19), 3013–3019. doi:10.1002/asia.202000655
Gonzalez, J., Sevilla, P., Gabarro-Riera, G., Jover, J., Echeverria, J., Fuertes, S., et al. (2021). A multifunctional dysprosium-carboxylato 2D metall-organic framework. Angew. Chem. Int. Ed. 60 (21), 12001–12006. doi:10.1002/anie.202100507
Goodwin, C. A. P., Ortu, F., Reta, D., Chilton, N. F., and Mills, D. P. (2017). Molecular magnetic hysteresis at 60 kelvin in dysprosocenium. Nature 548 (7668), 439–442. doi:10.1038/nature23447
Kapelewski, M. T., Runcevski, T., Tarver, J. D., Jiang, H. Z. H., Hurst, K. E., Parilla, P. A., et al. (2018). Record high hydrogen storage capacity in the metal-organic framework Ni2(m-dobdc) at near-ambient temperatures. Chem. Mat. 30 (22), 8179–8189. doi:10.1021/acs.chemmater.8b03276
Kitagawa, S., Kitaura, R., and Noro, S. (2004). Functional porous coordination polymers. Angew. Chem. Int. Ed. 43 (18), 2334–2375. doi:10.1002/anie.200300610
Li, A.-L., Gao, Q., Xu, J., and Bu, X.-H. (2017). Proton-conductive metal-organic frameworks: Recent advances and perspectives. Coord. Chem. Rev. 344, 54–82. doi:10.1016/j.ccr.2017.03.027
Li, W.-H., Deng, W.-H., Wang, G.-E., and Xu, G. (2020). Conductive MOFs. EnergyChem 2 (2), 100029. doi:10.1016/j.enchem.2020.100029
Li, X. L., Wang, A., Cui, M., Gao, C., Yu, X., Su, B., et al. (2022). Modulating two pairs of chiral Dy(III) enantiomers by distinct beta-diketone ligands to show giant differences in single-ion magnet performance and nonlinear optical response. Inorg. Chem. 61 (24), 9283–9294. doi:10.1021/acs.inorgchem.2c01031
Liu, C. M., Zhang, D. Q., and Zhu, D. B. (2016). A 3D MOF constructed from dysprosium(III) oxalate and capping ligands: Ferromagnetic coupling and field-induced two-step magnetic relaxation. Chem. Commun. 52 (26), 4804–4807. doi:10.1039/c6cc00498a
Meng, X., Wang, H. N., Song, S. Y., and Zhang, H. J. (2017). Proton-conducting crystalline porous materials. Chem. Soc. Rev. 46 (2), 464–480. doi:10.1039/c6cs00528d
Minguez Espallargas, G., and Coronado, E. (2018). Magnetic functionalities in MOFs: From the framework to the pore. Chem. Soc. Rev. 47 (2), 533–557. doi:10.1039/c7cs00653e
Parmar, V. S., Mills, D. P., and Winpenny, R. E. P. (2021). Mononuclear dysprosium alkoxide and aryloxide single-molecule magnets. Chem. Eur. J. 27 (28), 7625–7645. doi:10.1002/chem.202100085
Pinkowicz, D., Southerland, H. I., Avendano, C., Prosvirin, A., Sanders, C., Wernsdorfer, W., et al. (2015). Cyanide single-molecule magnets exhibiting solvent dependent reversible "on" and "off" exchange bias behavior. J. Am. Chem. Soc. 137 (45), 14406–14422. doi:10.1021/jacs.5b09378
Ramaswamy, P., Wong, N. E., and Shimizu, G. K. (2014). MOFs as proton conductors--challenges and opportunities. Chem. Soc. Rev. 43 (16), 5913–5932. doi:10.1039/c4cs00093e
Sasaki, T., Miyata, M., and Sato, H. (2018). Helicity and topological chirality in hydrogen-bonded supermolecules characterized by advanced graph set analysis and solid-state vibrational circular dichroism spectroscopy. Cryst. Growth Des. 18 (8), 4621–4627. doi:10.1021/acs.cgd.8b00599
Song, X. J., Hu, Z. B., Li, M. M., Feng, X., Kong, M., Xue, X. M., et al. (2021). Reversible switching of single-molecule magnetic behaviour by desorption/adsorption of solvent ligand in a new Dy(III)-Based metal organic framework. Front. Chem. 9, 714851. doi:10.3389/fchem.2021.714851
Su, J., He, W., Li, X.-M., Sun, L., Wang, H.-Y., Lan, Y.-Q., et al. (2020). High electrical conductivity in a 2D MOF with intrinsic superprotonic conduction and interfacial pseudo-capacitance. Matter 2 (3), 711–722. doi:10.1016/j.matt.2019.12.018
Su, J., Yuan, S., Li, J., Wang, H. Y., Ge, J. Y., Drake, H. F., et al. (2021). Rare-earth metal tetrathiafulvalene carboxylate frameworks as redox-switchable single-molecule magnets. Chem. Eur. J. 27 (2), 622–627. doi:10.1002/chem.202004883
Tang, Q., Liu, Y., Liu, S., He, D., Miao, J., Wang, X., et al. (2014). High proton conduction at above 100 °C mediated by hydrogen bonding in a lanthanide metal-organic framework. J. Am. Chem. Soc. 136 (35), 12444–12449. doi:10.1021/ja5069855
Vitillo, J. G., and Ricchiardi, G. (2017). Effect of pore size, solvation, and defectivity on the perturbation of adsorbates in MOFs: The paradigmatic Mg2(dobpdc) case study. J. Phys. Chem. C 121 (41), 22762–22772. doi:10.1021/acs.jpcc.7b06252
Wang, F. M., Hu, B. X., Lustig, W. P., Zhou, L., Xiang, J., Chen, L. Z., et al. (2021). Three robust blue-emitting anionic metal-organic frameworks with high stability and good proton conductivities. Inorg. Chem. 60 (23), 17926–17932. doi:10.1021/acs.inorgchem.1c02499
Wang, H.-Y., Cui, L., Xie, J.-Z., Leong, C. F., D’Alessandro, D. M., and Zuo, J.-L. (2017). Functional coordination polymers based on redox-active tetrathiafulvalene and its derivatives. Coord. Chem. Rev. 345, 342–361. doi:10.1016/j.ccr.2016.10.011
Wang, L., Zhu, L., Yin, P., Fu, W., Chen, J., Hao, J., et al. (2009). From 0D dimer to 2D network-supramolecular assembly of organic derivatized polyoxometalates with remote hydroxyl via hydrogen bonding. Inorg. Chem. 48 (19), 9222–9235. doi:10.1021/ic900985w
Woodruff, D. N., Winpenny, R. E., and Layfield, R. A. (2013). Lanthanide single-molecule magnets. Chem. Rev. 113 (7), 5110–5148. doi:10.1021/cr400018q
Wu, Y., Zhou, Y., Cao, S., Cen, P., Zhang, Y. Q., Yang, J., et al. (2020). Lanthanide metal-organic frameworks assembled from unexplored imidazolylcarboxylic acid: Structure and field-induced two-step magnetic relaxation. Inorg. Chem. 59 (17), 11930–11934. doi:10.1021/acs.inorgchem.0c01855
Yaghi, O. M., O'Keeffe, M., Ockwig, N. W., Chae, H. K., Eddaoudi, M., and Kim, J. (2003). Reticular synthesis and the design of new materials. Nature 423 (6941), 705–714. doi:10.1038/nature01650
Yamada, T., Otsubo, K., Makiura, R., and Kitagawa, H. (2013). Designer coordination polymers: Dimensional crossover architectures and proton conduction. Chem. Soc. Rev. 42 (16), 6655–6669. doi:10.1039/c3cs60028a
Yang, S. L., Li, G., Guo, M. Y., Liu, W. S., Bu, R., and Gao, E. Q. (2021). Positive cooperative protonation of a metal-organic framework: pH-responsive fluorescence and proton conduction. J. Am. Chem. Soc. 143 (23), 8838–8848. doi:10.1021/jacs.1c03432
Ye, Y., Gong, L., Xiang, S., Zhang, Z., and Chen, B. (2020). Metal-organic frameworks as a versatile platform for proton conductors. Adv. Mat. 32 (21), e1907090. doi:10.1002/adma.201907090
Yuan, N., Zhang, X., and Wang, L. (2020). The marriage of metal–organic frameworks and silica materials for advanced applications. Coord. Chem. Rev. 421, 213442. doi:10.1016/j.ccr.2020.213442
Yuan, S., Feng, L., Wang, K., Pang, J., Bosch, M., Lollar, C., et al. (2018). Stable metal-organic frameworks: Design, synthesis, and applications. Adv. Mat. 30 (37), e1704303. doi:10.1002/adma.201704303
Zhang, X., Vieru, V., Feng, X., Liu, J. L., Zhang, Z., Na, B., et al. (2015). Influence of guest exchange on the magnetization dynamics of dilanthanide single-molecule-magnet nodes within a metal-organic framework. Angew. Chem. Int. Ed. 54 (34), 9861–9865. doi:10.1002/anie.201503636
Zhong, L., Chen, W. B., Li, X. H., OuYang, Z. J., Yang, M., Zhang, Y. Q., et al. (2020). Four dinuclear and one-dimensional-chain dysprosium and terbium complexes based on 2-Hydroxy-3-methoxybenzoic acid: Structures, fluorescence, single-molecule-magnet, and ab initio investigation. Inorg. Chem. 59 (7), 4414–4423. doi:10.1021/acs.inorgchem.9b03555
Zhong, X., Hu, J.-J., Yao, S.-L., Zhang, R.-J., Wang, J.-J., Cai, D.-G., et al. (2022). Gd(III)-Based inorganic polymers, metal–organic frameworks and coordination polymers for magnetic refrigeration. CrystEngComm 24 (13), 2370–2382. doi:10.1039/d1ce01633d
Zhou, Y., Yu, F., Su, J., Kurmoo, M., and Zuo, J. L. (2020). Tuning electrical- and photo-conductivity by cation exchange within a redox-active tetrathiafulvalene-based metal-organic framework. Angew. Chem. Int. Ed. 59 (42), 18763–18767. doi:10.1002/anie.202008941
Zhu, M.-C., Huang, Y.-Y., Ma, J.-P., Hu, S.-M., Wang, Y., Guo, J., et al. (2018). Coordination polymers based on organic–inorganic hybrid rigid rod comprising a backbone of anderson-evans POMs. Cryst. Growth Des. 19 (2), 925–931. doi:10.1021/acs.cgd.8b01467
Keywords: coordination polymer, dysprosium, slow magnetic relaxation, proton conduction, multifunctional
Citation: Chen J-F, Ge Y-L, Wu D-H, Cui H-T, Mu Z-L, Xiao H-P, Li X and Ge J-Y (2022) Two-dimensional dysprosium(III) coordination polymer: Structure, single-molecule magnetic behavior, proton conduction, and luminescence. Front. Chem. 10:974914. doi: 10.3389/fchem.2022.974914
Received: 21 June 2022; Accepted: 12 July 2022;
Published: 08 August 2022.
Edited by:
Zhao-Bo Hu, Jiangxi University of Science and Technology, ChinaReviewed by:
Biing-Chiau Tzeng, National Chung Cheng University, TaiwanShin-Ichiro Noro, Hokkaido University, Japan
Longsheng Wang, Hubei University of Technology, China
Copyright © 2022 Chen, Ge, Wu, Cui, Mu, Xiao, Li and Ge. This is an open-access article distributed under the terms of the Creative Commons Attribution License (CC BY). The use, distribution or reproduction in other forums is permitted, provided the original author(s) and the copyright owner(s) are credited and that the original publication in this journal is cited, in accordance with accepted academic practice. No use, distribution or reproduction is permitted which does not comply with these terms.
*Correspondence: Jing-Yuan Ge, Z2VqaW5neXVhbjkwQDEyNi5jb20=; Hong-Ping Xiao, aHBfeGlhb0AxMjYuY29t; Xinhua Li, bGl4aW5odWEwMUAxMjYuY29t