- Laser Research Centre, Faculty of Health Sciences, University of Johannesburg, Johannesburg, South Africa
In recent years, the role of biocompatible nanocarriers (BNs) and their cancer cell targeting efficiency in photodynamic therapy (PDT) holds potential benefits for cancer treatment. Biocompatible and biodegradable nanoparticles are successfully used as carrier molecules to deliver cancer drugs and photosensitizers due to their material safety in the drug delivery system. Biocompatible nanocarriers are non-toxic and ensure high-level biocompatibility with blood, cells, and physiological conditions. The physicochemical properties of BNs often enable them to modify their surface chemistry, which makes conjugating specific ligands or antibodies to achieve cancer cell targeting drug delivery in PDT. This review article focuses on the various types of BNs used in targeted drug delivery, physicochemical properties, and surface chemistry of BNs in targeted drug delivery, advantages of BNs in drug delivery systems, and the targeting efficiency of BNs on some specific targeting receptors for cancer therapy. Furthermore, the review briefly recaps the nanocarrier-based targeted approaches in cancer PDT.
1 Introduction
Cancer is a leading cause of death worldwide and the World Health Organization (WHO) reports that nearly 10 million deaths from cancer by 2020. According to the WHO report, the most common types of cancer-related deaths are breast, lung, colon and rectal, prostate, skin, and stomach cancers (WHO, 2022). Currently, several conventional cancer treatment options available including surgery, radiotherapy, and chemotherapy (Arruebo et al., 2011). As part of a cancer chemotherapeutic regimen, various anticancer drugs are used. Although these drugs have good cytotoxic properties, they also have acute side effects and cancer recurrence complications. The most common adverse effects of DNA-damaging drugs are occurred in the skin, gastrointestinal tract, and bone marrow (Weber, 2014). Debela et al., recently investigated new cancer treatment approaches and procedures, including stem cell therapy, targeted therapy, ablation therapy, gene therapy, and natural antioxidants (Debela et al., 2021). However, they have their own advantages and disadvantages when it comes to cancer treatments, and some of them have really supplied new potential for the benefit of cancer patients.
Nanocarrier-based drug delivery has been extensively studied in recent years, and it has shown promising results in cancer treatment. Nanocarrier-mediated drug delivery system protects loaded drugs from degradation, allows drugs to circulate in the bloodstream for longer periods, and efficiently targets cancer cells (Din et al., 2017; Wang et al., 2020). Nanoengineering or the fabrication of nanomaterial processes, will play a key role in designing functionalized nanocarriers with appropriate ligands to deliver the drugs to the target site (Patra et al., 2018). Several types of nanomaterials can be formulated for targeting cancer therapy which includes polymeric nanoparticles (Prabhu et al., 2014), liposomes (Riaz et al., 2016), dendrimers (Baher, 2009), inorganic nanomaterials (Sun et al., 2021), polymeric micelles (Oerlemans et al., 2010), hydrogel (Narayanaswamy and Torchilin, 2019), and carbon-based nanomaterials (Sajjadi et al., 2021). Wang et al., 2020 discussed the advancement of nanoparticle-based combination therapy and dual drug delivery, which facilitate achieving the synergistic potential of the drugs and overcoming drug resistance (Wang and Huang, 2020). Co-delivery of synergistic drugs using nanomaterials has more significant advantages such as drug protection, sustained drug release pattern, and enhancing drug mechanism of action in the target site (Afsharzadeh et al., 2017). Nanoparticle-based drug delivery systems can effectively deliver drugs using dual-targeting, and multiple targeting approaches for cancer cell targeting and imaging functions. Multiple targeting approaches are frequently used to improve therapeutic efficacy by targeting the subcellular molecules such as mitochondria, nucleus, and endoplasmic reticulum (Fu et al., 2020).
Photodynamic therapy has gained attention in cancer treatment in recent years owing to their benefits, such as the ability to kill cancer cells without causing any injury. In a nutshell, PDT is a minimally invasive cancer treatment that includes three main components: photosensitizers, light, and oxygen. Light-activated photosensitizers initiate a photochemical reaction that promotes the formation of reactive oxygen species, which can result in cell death via apoptosis or necrosis (Agostinis et al., 2011). Nanomedicine combined with photodynamic therapy can significantly improve the antitumor efficacy through immunogenic cell death (Jin et al., 2021). Park et al. recently discussed the limitations of clinically available nanomedicine for PDT. Poor water solubility, low yield of singlet oxygen quantum, high doses required to achieve therapeutic efficacy, and poor targeting efficiency on tumor site are some of the limitations of photosensitizers. The advancement of nanotechnology in drug delivery made it possible to overcome the limitations of photosensitizers for PDT therapy (Dabrowski and Arnaut, 2015; Park et al., 2021). Kumar et al., recently investigated the use of various biocompatible nanocarriers for cancer PDT applications, and it has shown positive outcomes in in vitro cell line studies and in vivo animal studies, as well as discussing the status of global clinical trials for various types of cancers in PDT (Kumar and Abrahamse, 2021). Li et al. recently discussed the development of next-generation nanomedicine delivery systems for targeted therapy, including stimuli-responsive systems for active targeting, tissue microenvironment reprogramming strategies, immunotherapy and transcytosable nanomedicine (Li and Kataoka, 2021). There is significant potential for using the above-mentioned next-generation delivery systems for PDT therapy to achieve more promising cancer treatment outcomes.
This review article explores the recent advances in the development of biocompatible nanocarriers and their targeting efficiency in cancer photodynamic therapy. Thus, this review article is divided into six different sections. Section 1 briefly explains the introduction to the study. Section 2 studied the different targeting approaches in cancer treatment. In Section 3, discussed the commonly used biocompatible nanocarriers in photodynamic cancer therapy and our primary focus is on polymeric nanocarriers, liposomes and dendrimers are discussed in detail. Section 4 describes the different types of targeting receptors for cancer therapy, and some of the recently reported nanocarrier targeted approaches to a cancer cell in PDT. In Section 5, future perspectives of this work are briefly discussed.
2 Targeting approaches in cancer treatment
A targeted drug delivery system is an emerging cancer treatment platform that protects healthy cells from cytotoxic cancer drugs, reduces dose-related adverse effects, and prevents the formation of drug-resistant cancer cells (Attia et al., 2019; Yao et al., 2020). In cancer treatment, the physicochemical properties of nanomaterials and the physiological conditions of the tumor microenvironment play an important role in drug delivery to the target region (Uthaman et al., 2018). The targeting approaches are classified into two types: passive targeting mechanisms and active targeting mechanisms. These two approaches offer the ideal solution for increasing delivered nanoparticle accumulation at the target site (Kleinstreuer et al., 2014).
2.1 Passive targeting mechanism
Nano-sized materials with long systemic circulation properties frequently accumulate in the tumor site via a passive targeting mechanism which can be accomplished through an enhanced permeability and retention (EPR) effect. Nanomaterial accumulation in tumor cells via a passive targeting mechanism is entirely dependent on the permeability of leaky vasculature and decreased lymphatic drainage in tumor cells (Bazak et al., 2014; Nakamura et al., 2016; Wu, 2021). Figure 1 depicts schematic illustrations of passive targeting mechanisms.
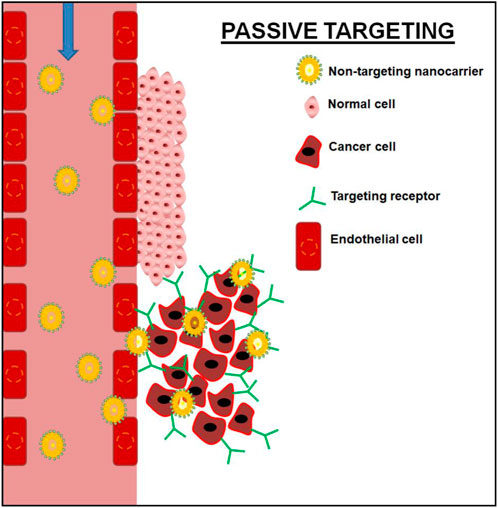
FIGURE 1. Schematic illustration of passive targeting of cancer cell using non-targeting nanocarrier.
Tsujimoto et al. (2021) synthesized fully polyethylene glycol-modified dendrimers with passive tumor targeting ability, and the hydration state of the dendrimer is important in drug carrier biodistribution. In vivo imaging revealed that the fully pegylated dendrimers accumulated in the tumor region of 8-week-old female balb/c mice injected with 4T1 (breast cancer cells). The presence of fully pegylated dendrimers in the tumor was also confirmed by ex vivo imaging results, as opposed to partly pegylated dendrimers. Several passively targeted nanomaterials are currently being tested in clinical trials for cancer treatment. Some of them are Doxil (a pegylated liposomal material for doxorubicin delivery, approved by FDA), DaunoXome (a liposomal material for daunorubicin delivery, approved by FDA), Marqibo (a liposomal material for vincristine sulfate delivery, approved by FDA), Onivyde or MM-398 (a pegylated liposomal material for Irinotecan delivery, approved by FDA), Abraxane (albumin-based nanoparticles for paclitaxel delivery, approved by FDA), Myocet (a liposomal material for doxorubicin delivery, approved in Europe and Canada), Mepact (a liposomal material for Muramyl tripeptide phosphatidyl-ethanolamine delivery, approved in Europe), SMANCS (a polymer conjugate for the delivery of neocarzinostatin, approved in Japan), and Genexol-PM (a polymeric micelle-based material for paclitaxel delivery, approved in Korea) (Shi et al., 2017).
2.2 Active targeting mechanisms
Selective targeting ligands/antibodies functionalized nanomaterials can target their appropriate receptors on cancer cells and facilitate the accumulation of active pharmaceutical ingredients in the tumor cell via an active targeting mechanism (Yoo et al., 2019). Figure 2 depicts schematic illustrations of active targeting mechanisms. Mehnath et al. (2020) synthesized and delivered paclitaxel for breast cancer treatment using active targeting polymeric micelles-based nanofibers, and it demonstrated high cytotoxicity against MCF-7 cells when compared to paclitaxel alone (Mehnath et al., 2020). Several active targeted nanomaterials are being tested in clinical trials for cancer treatment. Some of them are MM-302 (a HER2 receptor targeting liposomal material for doxorubicin delivery in Phase II/III clinical trial); BIND-014 (PSMA-targeting polymeric nanoparticles for docetaxel delivery in Phase II clinical trial); MBP-426 (a TfR targeting liposomal material for oxaliplatin delivery, in Phase I/II clinical trial); and Anti-EGFR immunoliposomes loaded with doxorubicin for solid tumors, in Phase I clinical trials (Shi et al., 2017).
3 Biocompatible nanocarriers in cancer photodynamic therapy
Biocompatible nanocarriers are widely used for various biomedical applications, including cancer PDT. Various types of biocompatible nanocarriers have been well reported for cancer PDT applications (Kumar and Abrahamse, 2021). This review focuses on three types of biocompatible nanocarriers that are commonly used for targeted approaches: polymeric nanoparticles, liposomes, and dendrimers. Figure 3 depicts schematic illustrations of biocompatible nanocarriers in cancer photodynamic therapy, and Table 1 discusses the various types of biocompatible nanocarriers used for cancer PDT in in vitro models.
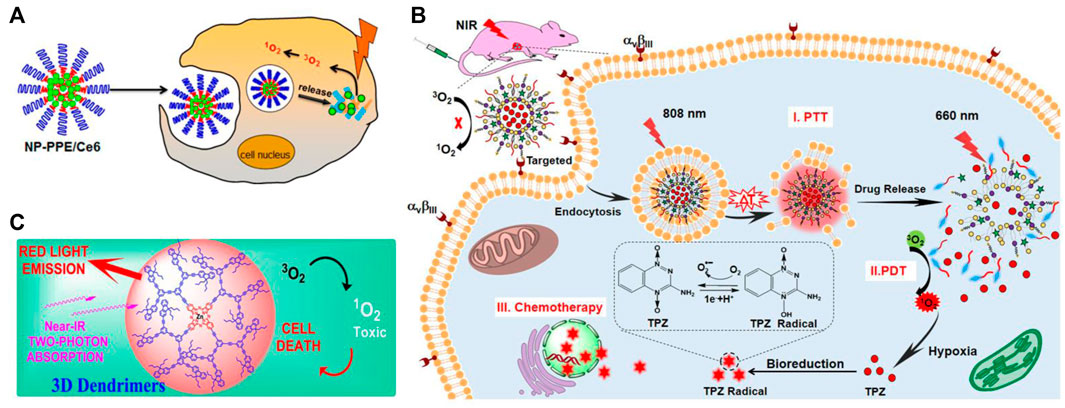
FIGURE 3. Schematic illustrations of biocompatible nanocarriers in cancer photodynamic therapy: (A) Polymeric nanoparticles—reprinted with permission from reference (Ding et al., 2015), Copyright 2015, American Chemical Society; (B) Liposomes—reprinted with permission from reference (Dai et al., 2019), Copyright 2019, American Chemical Society; and (C) Dendrimers—reprinted with permission from reference (Abid et al., 2021), Copyright 2021, American Chemical Society.
3.1 Polymeric nanocarriers
Polymeric nanocarriers show great promise as a drug-loading molecule in cancer PDT (Li and Huh, 2014). It has a wide range of drug delivery applications, including high biocompatibility, surface functionalization, targeted drug delivery, high stability, biodegradation, and an interesting bio-mimetic character (Avramovic et al., 2020; Zhang et al., 2020). Polymer-based drug delivery is frequently used to achieve sustained and effective controlled release of loaded drugs at the site of action. The molecular structure of the polymer is important in the drug release mechanism. Furthermore, it improves the physicochemical properties of the loaded drugs indirectly (Liechty et al., 2010; Herdiana et al., 2022). Although polymeric nanocarriers have many advantages in drug delivery, they do have some disadvantages. Some of them include poor hydrophilic drug encapsulation, drug leakage before reaching target cells, the use of toxic solvents in the fabrication process, and the production of toxic monomers as byproducts of the degradation process (Kamaly et al., 2016). Polymeric nanocarriers can be prepared from either natural polymers or synthetic polymers. Natural polymers are biopolymers that are broadly classified into two types: proteins and polysaccharides. Chitosan, cyclodextrin, cellulose, hyaluronic acid, and starch are some of the natural polymers that are actively used as nanocarriers in cancer PDT. Synthetic polymer-based nanoparticles are widely used in drug delivery applications, including cancer PDT. Poly (D,L-lactic-co-glycolic acid) (PLGA), Poly-N-isopropylacrylamide (PNIPAAM), Polylactic acid (PLA) and polycaprolactone (PCL) are a few examples. In both in vitro and in vivo studies, natural and synthetic polymers demonstrated excellent biocompatibility and biodegradability (Sundar et al., 2016).
Porphyrins-loaded fluorescent polymeric nanoparticles were synthesized using the electrostatic interaction self-assembly method and were successfully tested for their dual function approach, including in vivo imaging and PDT effects. The synthesized nanoparticles are highly biocompatible and demonstrated excellent synergistic effects with 650 nm laser irradiation, such as tumor growth inhibition and fluorescence imaging-guided phototherapy against CT-26 tumor-bearing mice (Hou et al., 2022). Cong et al., synthesized 200 nm-sized near-infrared cationic polymer-based nanoparticles using the ring-opening polymerization method. An in vivo PDT effects of synthesized nanoparticles + light were studied using a 4T1-bearing nude mice model, and the study confirmed the effective inhibition of tumor size compared to the control, PBS with light, and the nanoparticles without light (Cong et al., 2022). Biodegradable silicon naphthalocyanine (SiNc) nanoparticles have been synthesized for use in bioimaging and cancer phototherapy. The nanoparticles were intravenously injected into the A2780/AD tumor-bearing nude mice, and the results showed that the tumors were completely eradicated in 3–5 days, with no recurrence of tumors detected (Taratula et al., 2015). In vivo photodynamic therapeutic effect of Chlorin e6 (Ce6) loaded nanomaterials were tested in BcPC-3 tumor-bearing mice. The synthesized Ce6-loaded nanomaterials were intravenously injected into tumor-bearing mice, and NIR light (660 nm, 0.5 W/cm2) was used to irradiate tumors; control mice were kept without laser irradiation. It has been confirmed that Ce6-loaded nanoparticles and NIR irradiation significantly inhibited tumor cell proliferation and apoptosis in tumor tissues (Ding et al., 2015). Li et al., synthesized chlorin e6 loaded hydrogen peroxide and poly (amidoamine) coassembled with triblock copolymer based polymeric vesicles for effective PDT treatment for hypopxic tumor and these nanocarriers not only facilitate delivery of photosensitizer but also have other functions like hypoxia alleviation (Li et al., 2017).
3.2 Liposomes
Liposomes are non-toxic, biodegradable spherical vesicles composed of a phospholipid bilayer, and it offers a promising carrier molecule in drug delivery (Akbarzadeh et al., 2013). Liposomes improve bioavailability and reduce the toxicity of loaded active pharmaceutical ingredients. Some of the pH-responsive liposomes provide numerous benefits in site-specific drug release (Lee and Thompson, 2017). Liposomes are commonly used as a carrier molecule for the delivery of photosensitizers in cancer PDT applications (Moghassemi et al., 2021). Liposomes have some drawbacks in drug delivery, including poor stability, low solubility, a short half-life, drug leakage and high production costs (Leitgeb et al., 2020).
In A549 tumor-bearing nude mice, the therapeutic efficacy of Indocyanine green (ICG), hypoxia-activated prodrug tirapazamine (TPZ), and Ce6-loaded multifunctional theranostic liposomes was investigated. Time-dependent fluorescence imaging studies confirmed that synthesized liposomes were actively targeting and accumulated in the tumor region. An in vivo activation of PDT and immunofluorescence staining study demonstrated that laser irradiation increases strong hypoxic signals due to the continuous oxygen consumption, and this leads to the activation of TPZ, facilitating achieving tumor-selective combination therapy (Dai et al., 2019). The synthesized long-circulating liposomes were injected intravenously into Meth-A sarcoma-bearing mice and irradiated using the following laser conditions: 689 nm laser, 150 J/cm2, 0.25W, and the study results show that the tumor growth was strongly suppressed (Ichikawa et al., 2005). Zhang et al., synthesized a novel liposome encapsulated with Ce6, Tirapazamine and gene probe for photodynamic and chemotherapy applications. An in vivo study was conducted using MCF-7 cells injected into a nude mouse, and the mice were then allowed to develop ∼75 mm3 tumors. After 4 h, the nanoparticle was injected into tumor-bearing mice and exposed to laser irradiation (670 nm laser). The results of the study revealed that the tumor was almost completely eliminated when compared to the control, indicating the synergistic effects of synthesized liposomes (Zhang et al., 2018).
3.3 Dendrimers
Dendrimers are multifunctional, hyperbranched polymers that have the potential to be used as functional materials in cancer photomedicine. It has a wide range of biological applications, such as theranostics, combination therapy, molecular imaging, and PDT/PTT (Ouyang et al., 2021). Dendrimer-based nanocarriers have shown promise in protecting the nature of phototherapeutic drugs in PDT applications. Dendrimer’s structural advantages enable easier surface modification of targeting ligands or functional moieties for targeted cancer therapy, and it improves therapeutic compound accumulation in the targeted site (Gupta et al., 2010; Zhu et al., 2019; Gao et al., 2020). At the same time, dendrimers have their own limitations in terms of proven toxicity issues, but there are several approaches, such as surface modifications using biocompatible materials, that can overcome dendrimer limitations (Chis et al., 2020).
Xu et al. (2019) investigated the efficacy of Ce6-loaded dendrimers in U14 tumor-bearing nude mice with the following in vivo PDT conditions: 670 nm laser (10 mW/cm2) for 30 min. An in vivo fluorescence imaging study revealed that Ce6-loaded dendrimers (with MnO2) completely inhibited tumor growth in mice when compared to free Ce6. Nishiyama et al., synthesized a dendrimer phthalocyanine encapsulated polymeric micelle for improved photodynamic cancer therapy. In vitro photocytotoxicity of a dendrimer-based polymer micelle was tested against A549 cells after laser irradiation using 670 nm diode laser, and it demonstrated fluence-rate dependent phototoxicity and induced photodamage in the mitochondria. An in vivo animal experiment was carried out using A549 lung cancer cells bearing mice, and the study results show the effective antitumor activity compared to the clinically used Photofrin (Nishiyama et al., 2009). Kojima et al., prepared rosebengal (RB) and protoporphyrin IX (PpIX) encapsulated in pegylated poly (amido amine) and poly (propylene imine) for PDT applications. The results of an in vitro cytotoxicity study on HeLa cells confirmed that RB alone and RB loaded dendrimers were equally toxic. Similar results were obtained in protoporphyrin IX-loaded dendrimers, confirming the easy release pattern of photosensitizers from dendrimers. Furthermore, the study found that hydrophilic RB releases faster than hydrophobic PpIX in PBS (Kojima et al., 2007).
4 Different types of targeting receptors for cancer therapy
Currently, there are several types of targeting receptors or ligands are currently available for nanocarrier-based targeted cancer therapy. Here are some examples: folate receptor, asialoglycoprotein receptor, CD 44 receptor, Her2—Herceptin receptor, CD 20 antigen; CD 19 antigen, EGFR receptor, CD 326 receptor, VEGF receptor, transferrin receptor, luteinizing hormone-releasing hormone receptor, and αvβ3 receptor (Yu et al., 2010). Table 2 lists the most commonly used targeting ligands and receptors for nanocarrier-based drug delivery systems in cancer therapy.
4.1 Nanocarrier-based targeted approaches in cancer PDT
In cancer PDT research, nanocarrier-based targeted approaches show some promising results. Figure 4 shows schematic illustrations of confocal laser scanning microscopy images of various types of nanoparticles and their targeting efficiency with different receptors.
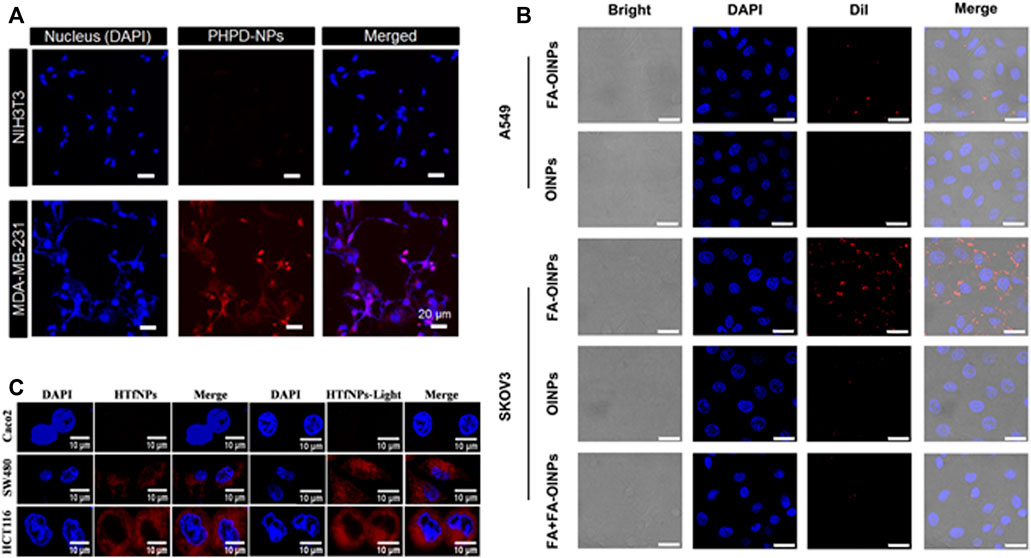
FIGURE 4. Schematic illustrations of confocal laser scanning microscopy images of different types of nanoparticles and their targeting efficiency with different receptors. (A) CD 44 receptor—reprinted with permission from reference (Han et al., 2016), Copyright 2016, American Chemical Society; (B) Folate receptor—reprinted with permission from reference (Liu et al., 2019), Copyright 2019, American Chemical Society and (C) Transferrin receptor—reprinted with permission from reference (Sardoiwala et al., 2020), Copyright 2020, American Chemical Society.
Core-shell nanoparticles in the size range of ∼ 130 nm were synthesized to target CD 44 receptor on the MDA-MB 231 cell line, which is CD 44 receptor overexpressing cell line. A conventional small-molecule photosensitizer (PS) loaded polydopamine nanoparticles serve as a shell, and PS conjugated hyaluronic acid serves as a shell molecule. An in vitro cancer-targeting efficiency of synthesized core-shell nanoparticles was tested with both CD44 high expressing (MDA-MB 231 cells) and CD44 low expressing cells (NIH3T3 cells) using confocal laser scanning microscopy. Because of the presence of hyaluronic acid on the shell, the fluorescence signals in MDA-MB 231 cells were significantly higher than in NIH3T3 cells (Han et al., 2016). Lipid-based nanoparticles are used to target folate receptors (FRs) overexpressing SKOV3 cell line (human ovarian cancer cells), the synthesized nanoparticles are in the size range of 301.08 ± 11.12 nm. Multifunctional theranostic nanoparticles (folate-targeted and oxygen/indocyanine green-loaded lipid nanoparticles) are synthesized using a two-step emulsion method. The confocal microscopy study found that folic acid (FA) loaded nanoparticles treated SKOV3 cells had a stronger fluorescence signal than A549 cells. A quantitative flow cytometry study using SKOV3 treated cells revealed that the fluorescence intensity of FA conjugated lipid nanoparticles was 2.3 times higher than that of FA unloaded nanoparticles (Liu et al., 2019).
Sardoiwala et al. (2020) synthesized hypericin-loaded transferrin nanoparticles with the size range of ∼65 ± 5 nm and aimed to target transferrin receptor in three different colon cancer cells {Caco2 [low expression of CD71 (transferrin receptor)], SW480, and HCT116 cells [higher expression of CD71 (transferrin receptor)]}. Cellular internalization studies revealed that higher expression of synthesized nanoparticles in HCT116 and SW480 cells than in Caco2 cells, and this was confirmed with flow cytometric studies, and the observed percentages are higher in HCT116 (80–90%) and SW480 (70–80%) cells than Caco2 cells (10–20%) . Cai et al., synthesized dual-targeted organic nanoparticles with two targeting ligands: folate and cRGD peptide in different ratios. The nanoparticles are ∼ 20 nm in size and are designed to target both folate and αvβ3 integrin receptors. An in vitro cellular uptake study was performed with NIH/3T3 normal cells and U87MG glioblastoma cells. Surface density of 75% cRGD and 25% FA showed a better cellular uptake on U87MG glioblastoma cells than NIH/3T3 cells (Cai et al., 2019).
5 Future perspectives and conclusion
In this review article, we discussed the most recent advancements in the use of biocompatible nanoparticles for targeted PDT cancer therapy. We focused on three types of biocompatible nanoparticles: polymeric nanoparticles, liposomes and dendrimers. It demonstrates promising potential and distinct advantages on surface modification using various target ligands/antibodies for cancer therapy. The results of an in vitro and in vivo study confirmed the benefits of using those biocompatible nanocarriers for cancer PDT. Both passive and active targeting mechanisms show significant advantages on using biocompatible nanocarriers to deliver therapeutic drugs/photosensitizers with enhancing the accumulation of loaded compounds to the targeted site of action. Furthermore, we reported the most used targeting ligands and targeting receptors in nanomedicine for various types of targeted cancer therapy. The advancement of nanoengineering provides excellent opportunities in targeted cancer PDT for various types of cancers. Some advanced nanoengineering applications, such as dual receptor targeting strategies, intracellular organelle targeting, and multifunctional nanocarrier will have a significant impact on current treatment modalities.
Author contributions
SD: conceptualization, investigation, and writing of the original draft. HA: revising critically for intellectual content. All authors have read and agreed to the published version of the manuscript.
Funding
This work is based on the research supported by the South African Research Chairs Initiative of the Department of Science and Technology and the National Research Foundation of South Africa (Grant No 98337), as well as grants received from the University of Johannesburg (URC), the National Research Foundation (NRF), and the CSIR (Council for Scientific and Industrial Research)—NLC (National Laser Centre) Laser Rental Pool Programme.
Conflict of interest
The authors declare that the research was conducted in the absence of any commercial or financial relationships that could be construed as a potential conflict of interest.
Publisher’s note
All claims expressed in this article are solely those of the authors and do not necessarily represent those of their affiliated organizations, or those of the publisher, the editors and the reviewers. Any product that may be evaluated in this article, or claim that may be made by its manufacturer, is not guaranteed or endorsed by the publisher.
Abbreviations
BNs, Biocompatible nanocarriers; 4T1, Breast cancer cells; CD44, Cell surface adhesion receptor 44; Ce6, Chlorin e6; DNA, Deoxyribonucleic acid; EPR, Enhanced Permeation and Retention; FDA, Food and Drug Administration, FRs, Folate receptors; HER, Herceptin; ICG, Indocyanine green; MCF-7, Breast cancer cells; mg, Miligram; mTHPC, m-tetrahydroxyphenylchlorin; NIR, Nearinfrared; nm, Nanometer; PBS, Phosphate buffered saline; PCL, Polycaprolactone; PDT, Photodynamic therapy; PNIPAAM, Poly-N-isopropylacrylamide; PpIX, Protoporphyrin IX; PTT, Photothermal therapy; RB, Rosebengal; SiNc, Silicon naphthalocyanine; TBD, 1,5,7-triazabicylo [4.4.0] dec-5-ene; TPZ, Tirapazamine; WHO, World Health Organization.
References
Abid, S., Hassine, S. B., Sun, Z., Richy, N., Camerel, F., Jamoussi, B., et al. (2021). Impact of changing the core in tetrapyrrolic dendrimers designed for oxygen sensitization: New fluorescent phthalocyanine-based dendrimers with high two-photon absorption cross-sections. Macromolecules 54, 6726–6744. doi:10.1021/acs.macromol.1c00830
Afsharzadeh, M., Hashemi, M., Mokhtarzadeh, A., Abnous, K., and Ramezani, M. (2017). Recent advances in co-delivery systems based on polymeric nanoparticle for cancer treatment. Artif. Cells Nanomed. Biotechnol. 46, 1095–1110. doi:10.1080/21691401.2017.1376675
Agostinis, P., Berg, K., Cengel, K. A., Foster, T. H., Girotti, A. W., Gollnick, S. O., et al. (2011). Photodynamic therapy of cancer: An update. CA A Cancer J. Clin. 61, 250–281. doi:10.3322/caac.20114
Ahmed, M. S. U., Salam, A. B., Yates, C., Willian, K., Jaynes, J., Turner, T., et al. (2017). Double-receptor-targeting multifunctional iron oxide nanoparticles drug delivery system for the treatment and imaging of prostate cancer. Int. J. Nanomedicine 12, 6973–6984. doi:10.2147/ijn.s139011
Akbarzadeh, A., Rezaei-sadabady, R., Davaran, S., Joo, S. W., Zarghami, N., Hanifehpour, Y., et al. (2013). Liposome: Classification, preparation, and applications. Nanoscale Res. Lett. 8, 102. doi:10.1186/1556-276X-8-102
Amreddy, N., Babu, A., Panneerselvam, J., Srivastava, A., Muralidharan, R., Chen, A., et al. (2018). Chemo-biologic combinatorial drug delivery using folate receptor-targeted dendrimer nanoparticles for lung cancer treatment. Nanomedicine Nanotechnol. Biol. Med. 14, 373–384. doi:10.1016/j.nano.2017.11.010
Arruebo, M., Vilaboa, N., Saez-Gutierrez, B., Lambea, J., Tres, A., Valladares, M., et al. (2011). Assessment of the evolution of cancer treatment therapies. Cancers 3, 3279–3330. doi:10.3390/cancers3033279
Attia, M. F., Anton, N., Wallyn, J., Omran, Z., and Vandamme, T. F. (2019). An overview of active and passive targeting strategies to improve the nanocarriers efficiency to tumour sites. J. Pharm. Pharmacol. 71, 1185–1198. doi:10.1111/jphp.13098
Avramovic, N., Mandic, B., Savic-Radojevic, A., and Simic, T. (2020). Polymeric nanocarriers of drug delivery systems in cancer therapy. Pharmaceutics 12, 298. doi:10.3390/pharmaceutics12040298
Ayoub, A. M., Amin, M. U., Ambreen, G., Dayyih, A. A., Abdelsalam, A. M., Somaida, A., et al. (2021). Photodynamic and antiangiogenic activities of parietin liposomes in triple negative breast cancer. Biomater. Adv. 134, 112543. doi:10.1016/j.msec.2021.112543
Baher, J. R. (2009). Dendrimer-based nanoparticles for cancer therapy. Hematol. Am. Soc. Hematol. Edu Program 709, 708–719. doi:10.1182/asheducation-2009.1.708
Bazak, R., Houri, M., Achy, S. E., Hussein, W., and Refaat, T. (2014). Passive targeting of nanoparticles to cancer: A comprehensive review of the literature. Mol. Clin. Oncol. 2, 904–908. doi:10.3892/mco.2014.356
Cai, X., Bandla, A., Chuan, C. K., Magarajah, G., Liao, L-D., Teh, D. B. L., et al. (2019). Identifying glioblastoma margins using dual-targeted organic nanoparticles for efficient in vivo fluorescence image-guided photothermal therapy. Mat. Horiz. 6, 311–317. doi:10.1039/c8mh00946e
Chis, A. A., Dobrea, C., Morgovan, C., Arseniu, A. M., Rus, L. L., Butuca, A., et al. (2020). Applications and limitations of dendrimers in biomedicine. Molecules 25, 3982. doi:10.3390/molecules25173982
Cong, Z., Xie, S., Jiang, Z., Zheng, S., Wang, W., Wang, W., et al. (2022). In vivo photodynamic therapy based on Near-Infrared AIE cationic polymers. Chem. Eng. J. 431, 133748. doi:10.1016/j.cej.2021.133748
Dabrowski, J. M., and Arnaut, L. G. (2015). Photodynamic therapy (PDT) of cancer: From local to systemic treatment. Photochem. Photobiol. Sci. 14, 1765–1780. doi:10.1039/c5pp00132c
Dai, Y., Wang, B., Sun, Z., Cheng, J., Zhao, H., Wu, K., et al. (2019). Multifunctional theranostic liposomes loaded with a hypoxia activated prodrug for cascade-activated tumor selective combination therapy. ACS Appl. Mat. Interfaces 11, 39410–39423. doi:10.1021/acsami.9b11080
Debela, D. T., Muzazu, S. G. Y., Heraro, K. D., Ndalama, M. T., Mesele, B. W., Haile, D. C., et al. (2021). New approaches and procedures for cancer treatment: Current perspectives. SAGE Open Med. 9, 20503121211034366. doi:10.1177/20503121211034366
Diao, L., Shen, A., Yang, Y., Tao, J., and Hu, Y. (2019). CD44-targeted hyaluronic acid–curcumin reverses chemotherapeutics resistance by inhibiting P-gp and anti-apoptotic pathways. RSC Adv. 9, 40873–40882. doi:10.1039/c9ra08202f
Din, F., Aman, W., Ullah, I., Qureshi, O. S., Mustapha, O., Shafique, S., et al. (2017). Effective use of nanocarriers as drug delivery systems for the treatment of selected tumors. Int. J. Nanomedicine 12, 7291–7309. doi:10.2147/ijn.s146315
Ding, F., Li, H-J., Wang, J-X., Tao, W., Zhu, Y-H., Yu, Y., et al. (2015). Chlorin e6-Encapsulated Polyphosphoester Based Nanocarriers with Viscous Flow Core for Effective Treatment of Pancreatic Cancer. ACS Appl. Mat. Interfaces 7, 18856–18865. doi:10.1021/acsami.5b05724
Dixit, S., Novak, T., Miller, K., Zhu, Y., Kenney, M. E., and Broome, A. M. (2015). Transferrin receptor-targeted theranostic gold nanoparticles for photosensitizer delivery in brain tumors. Nanoscale 7, 1782–1790. doi:10.1039/c4nr04853a
Duwa, R., Banstola, A., Emami, F., Jeong, J-H., Lee, S., and Yook, S. (2020). Cetuximab conjugated temozolomide-loaded poly (lactic-co-glycolic acid) nanoparticles for targeted nanomedicine in EGFR overexpressing cancer cells. J. Drug Deliv. Sci. Technol. 60, 101928. doi:10.1016/j.jddst.2020.101928
Feuser, P. E., Cordeiro, A. P., de Bem Silverira, G., Eduarda, M., Correa, A. B., Silveira, P. C. L., et al. (2021). Co-encapsulation of sodium diethyldithiocarbamate (DETC) and zinc phthalocyanine (ZnPc) in liposomes promotes increases phototoxic activity against (MDA-MB 231) human breast cancer cells. Colloids Surfaces B Biointerfaces 197, 111434. doi:10.1016/j.colsurfb.2020.111434
Fu, X., Shi, Y., Qi, T., Qiu, S., Huang, Y., Zhao, X., et al. (2020). Precise design strategies of nanomedicine for improving cancer therapeutic efficacy using subcellular targeting. Signal Transduct. Target. Ther. 5, 262. doi:10.1038/s41392-020-00342-0
Gao, D., Guo, X., Zhang, X., Chen, S., Wang, Y., Chen, T., et al. (2020). Multifunctional phototheranostic nanomedicine for cancer imaging and treatment. Mat. Today Bio 5, 100035. doi:10.1016/j.mtbio.2019.100035
Gupta, U., Dwivedi, S. K., Bid, H. K., Konwar, R., and Jain, N. K. (2010). Ligand anchored dendrimers based nanoconstructs for effective targeting to cancer cells. Int. J. Pharm. X. 393, 186–197. doi:10.1016/j.ijpharm.2010.04.002
Han, J., Park, W., and Na, K. (2016). Photosensitizer-conjugated hyaluronic acid-shielded polydopamine nanoparticles for targeted photomediated tumor therapy. ACS Appl. Mat. Interfaces 8, 7739–7747. doi:10.1021/acsami.6b01664
Herdiana, Y., Wathoni, N., Shamsuddin, S., and Muchtaridi, M. (2022). Drug release study of the chitosan-based nanoparticles. Heliyon 8, e08674. doi:10.1016/j.heliyon.2021.e08674
Hou, M., Chen, W., Zhao, J., Dai, D., Yang, M., and Yi, C. (2022). Facile synthesis and in vivo bioimaging applications of porphyrin derivative-encapsulated polymer nanoparticles. Chin. Chem. Lett. 33, 4101–4106. doi:10.1016/j.cclet.2022.01.049
Ichikawa, K., Hikita, T., Maeda, N., Yonezawa, S., Takeuchi, Y., Asai, T., et al. (2005). Antiangiogenic photodynamic therapy (PDT) by using long-circulating liposomes modified with peptide specific to angiogenic vessels. Biochimica Biophysica Acta - Biomembr. 1669, 69–74. doi:10.1016/j.bbamem.2005.02.003
Jenkins, S. V., Nima, Z. A., Vang, K. B., Kannarpady, G., Nedosekin, D. A., Zharov, V. P., et al. (2017). Triple-negative breast cancer targeting and killing by EpCAM-directed, plasmonically active nanodrug systems. NPJ Precis. Oncol. 1, 27. doi:10.1038/s41698-017-0030-1
Jiang, S., Wang, X., Zhang, Z., Sun, L., Pu, Y., Yao, H., et al. (2016). CD20 monoclonal antibody targeted nanoscale drug delivery system for doxorubicin chemotherapy: An in vitro study of cell lysis of CD20-positive raji cells. Int. J. Nanomedicine 11, 5505–5518. doi:10.2147/ijn.s115428
Jin, F., Liu, D., Xu, X., Ji, J., and Du, Y. (2021). Nanomaterials-based photodynamic therapy with combined treatment improves antitumor efficacy through boosting immunogenic cell death. Int. J. Nanomedicine 16, 4693–4712. doi:10.2147/ijn.s314506
Kamaly, N., Yameen, B., Wu, J., and Farokhzad, O. C. (2016). Degradable controlled-release polymers and polymeric nanoparticles: Mechanisms of controlling drug release. Chem. Rev. 116, 2602–2663. doi:10.1021/acs.chemrev.5b00346
Kleinstreuer, C., Feng, Y., and Childress, E. (2014). Drug-targeting methodologies with applications: A review. World J. Clin. Cases 2, 742–756. doi:10.12998/wjcc.v2.i12.742
Kojima, C., Toi, Y., Harada, A., and Kono, K. (2007). Preparation of poly(ethylene glycol)-attached dendrimers encapsulating photosensitizers for application to photodynamic therapy. Bioconjug. Chem. 18, 663–670. doi:10.1021/bc060244u
Krishnan, V., Xu, X., Kelly, D., Snook, A., Waldman, S. A., Mason, R. W., et al. (2015). CD19-Targeted nanodelivery of doxorubicin enhances therapeutic efficacy in B-cell acute lymphoblastic leukemia. Mol. Pharm. 12, 2101–2111. doi:10.1021/acs.molpharmaceut.5b00071
Kumar, S. S. D., and Abrahamse, H. (2021). Biocompatible nanocarriers for enhanced cancer photodynamic therapy applications. Pharmaceutics 13, 1933. doi:10.3390/pharmaceutics13111933
Kumar, S. S. D., Mahesh, A., Antoniraj, M. G., Rathore, H. S., Houreld, N. N., and Kandasamy, R. (2018). Cellular imaging and folate receptor targeting delivery of gum kondagogu capped gold nanoparticles in cancer cells. Int. J. Biol. Macromol. 109, 220–230. doi:10.1016/j.ijbiomac.2017.12.069
Lee, Y., and Thompson, D. H. (2017). Stimuli-responsive liposomes for drug delivery. Wiley Interdiscip. Rev. Nanomed Nanobiotechnol 9, 1450. doi:10.1002/wnan.1450
Leitgeb, M., Knez, Z., and Primozic, M. (2020). Sustainable technologies for liposome preparation. J. Supercrit. Fluids 165, 104984. doi:10.1016/j.supflu.2020.104984
Li, J., and Kataoka, K. (2021). Chemo-physical strategies to advance the in vivo functionality of targeted nanomedicine: The next generation. J. Am. Chem. Soc. 143, 538–559. doi:10.1021/jacs.0c09029
Li, J., Wei, K., Zuo, S., Xu, Y., Zha, Z., Ke, W., et al. (2017). Light-Triggered clustered vesicles with self-supplied oxygen and tissue penetrability for photodynamic therapy against hypoxic tumor. Adv. Funct. Mat. 27, 1702108. doi:10.1002/adfm.201702108
Li, L., and Huh, K. M. (2014). Polymeric nanocarrier systems for photodynamic therapy. Biomater. Res. 18, 19. doi:10.1186/2055-7124-18-19
Liang, S., Sun, C., Yang, P., Ma, P., Huang, S., Cheng, Z., et al. (2020). Core-shell structured upconversion nanocrystal-dendrimer composite as a carrier for mitochondria targeting and catalase enhanced anti-cancer photodynamic therapy. Biomaterials 240, 119850. doi:10.1016/j.biomaterials.2020.119850
Liechty, W. B., Kryscio, D. R., Slaughter, B. V., and Peppas, N. A. (2010). Polymers for drug delivery systems. Annu. Rev. Chem. Biomol. Eng. 1, 149–173. doi:10.1146/annurev-chembioeng-073009-100847
Liu, Y., Chen, S., Sun, J., Zhu, S., Chen, C., Xie, W., et al. (2019). Folate-targeted and oxygen/indocyanine green-loaded lipid nanoparticles for dual-mode imaging and photo-sonodynamic/photothermal therapy of ovarian cancer in vitro and in vivo. Mol. Pharm. 16, 4104–4120. doi:10.1021/acs.molpharmaceut.9b00339
Mehnath, S., Chitra, K., Karthikeyan, K., and Jeyaraj, M. (2020). Localized delivery of active targeting micelles from nanofibers patch for effective breast cancer therapy. Int. J. Pharm. X. 584, 119412. doi:10.1016/j.ijpharm.2020.119412
Moghassemi, S., Dadashzadeh, A., Azevedo, R. B., Feron, O., and Amorim, C. A. (2021). Photodynamic cancer therapy using liposomes as an advanced vesicular photosensitizer delivery system. J. Control. Release 339, 75–90. doi:10.1016/j.jconrel.2021.09.024
Nakamura, Y., Mochida, A., Choyke, P. L., and Kobayashi, H. (2016). Nano-drug delivery: Is the enhanced permeability and retention (EPR) effect sufficient for curing cancer? Bioconjug. Chem. 27, 2225–2238. doi:10.1021/acs.bioconjchem.6b00437
Narayanaswamy, R., and Torchilin, V. P. (2019). Hydrogels and their applications in targeted drug delivery. Molecules 24, 603. doi:10.3390/molecules24030603
Nishiyama, N., Nakagishi, Y., Morimoto, Y., Lai, P. S., Miyazaki, K., Urano, K., et al. (2009). Enhanced photodynamic cancer treatment by supramolecular nanocarriers charged with dendrimer phthalocyanine. J. Control. Release 133, 245–251. doi:10.1016/j.jconrel.2008.10.010
Oerlemans, C., Bult, W., Bos, M., Storm, G., Nijsen, J. F. W., and Hennink, W. E. (2010). Polymeric micelles in anticancer therapy: Targeting, imaging and triggered release. Pharm. Res. 27, 2569–2589. doi:10.1007/s11095-010-0233-4
Ouyang, Z., Gao, Y., Shen, M., and Shi, X. (2021). Dendrimer-based nanohybrids in cancer photomedicine. Mat. Today Bio 10, 100111. doi:10.1016/j.mtbio.2021.100111
Park, J., Lee, Y-K., Park, I-K., and Hwang, S. R. (2021). Current limitations and recent progress in nanomedicine for clinically available photodynamic therapy. Biomedicines 9, 85. doi:10.3390/biomedicines9010085
Patra, J. K., Das, G., Fraceto, L. F., Campos, E. V. R., Rodriguez-Torres, M., Acosta-Torres, L. S., et al. (2018). Nano based drug delivery systems: Recent developments and future prospects. J. Nanobiotechnology 16, 71. doi:10.1186/s12951-018-0392-8
Prabhu, R., Patravale, V., and Joshi, M. D. (2014). Polymeric nanoparticles for targeted treatment in oncology: Current insights. Int. J. Nanomedicine 10, 1001–1018. doi:10.2147/ijn.s56932
Pranatharthiharan, S., Patel, M. D., Malshe, V. C., Pujari, V., Gorakshakar, A., Madkaikar, M., et al. (2017). Asialoglycoprotein receptor targeted delivery of doxorubicin nanoparticles for hepatocellular carcinoma. Drug Deliv. (Lond). 24, 20–29. doi:10.1080/10717544.2016.1225856
Riaz, M. K., Riaz, M. A., Zhang, X., Lin, C., Wong, K. H., Chen, X., et al. (2016). Surface functionalization and targeting strategies of liposomes in solid tumor therapy: A review. Int. J. Mol. Sci. 19, 195. doi:10.3390/ijms19010195
Sajjadi, M., Nasrollahzadeh, M., Jaleh, B., Soufi, G. J., and Iravani, S. (2021). Carbon-based nanomaterials for targeted cancer nanotherapy: Recent trends and future prospects. J. Drug Target. 29, 716–741. doi:10.1080/1061186x.2021.1886301
Sardoiwala, M. N., Kushwaha, A. C., Dev, A., Shrimali, N., Guchhait, P., Karmakar, S., et al. (2020). Hypericin-loaded transferrin nanoparticles induce PP2A-regulated BMI1 degradation in colorectal cancer-specific chemo-photodynamic therapy. ACS Biomater. Sci. Eng. 6, 3139–3153. doi:10.1021/acsbiomaterials.9b01844
Schoppa, T., Jung, D., Rust, T., Mulac, D., Kuckling, D., and Langer, K. (2021). Light-responsive polymeric nanoparticles based on a novel nitropiperonal based polyester as drug delivery systems for photosensitizers in PDT. Int. J. Pharm. X. 597, 120326. doi:10.1016/j.ijpharm.2021.120326
Shan, D., Li, J., Cai, P., Prasad, P., Liu, F., Rauth, A. M., et al. (2015). RGD-conjugated solid lipid nanoparticles inhibit adhesion and invasion of αvβ3 integrin-overexpressing breast cancer cells. Drug Deliv. Transl. Res. 5, 15–26. doi:10.1007/s13346-014-0210-2
Shi, J., Kantoff, P. W., Wooster, R., and Farokhzad, O. C. (2017). Cancer nanomedicine: Progress, challenges and opportunities. Nat. Rev. Cancer 17, 20–37. doi:10.1038/nrc.2016.108
Su, T., Cheng, F., Pu, Y., Cao, J., Lin, S., Zhu, G., et al. (2021). Polymeric micelles amplify tumor oxidative stresses through combining PDT and glutathione depletion for synergistic cancer chemotherapy. Chem. Eng. J. 411, 128561. doi:10.1016/j.cej.2021.128561
Sun, M., Wang, T., Li, L., Li, X., Zhai, Y., Zhang, J., et al. (2021). The application of inorganic nanoparticles in molecular targeted cancer therapy: EGFR targeting. Front. Pharmacol. 12, 702445. doi:10.3389/fphar.2021.702445
Sundar, D. S., Antoniraj, M. G., Kumar, C. S., Mohapatra, S. S., Houreld, N. N., and Ruckmani, K. (2016). Recent trends of biocompatible and biodegradable nanoparticles in drug delivery: A review. Curr. Med. Chem. 23, 3730–3751. doi:10.2174/0929867323666160607103854
Taratula, O., Doddapaneni, B. S., Schumann, C., Li, X., Bracha, S., Milovancev, M., et al. (2015). Naphthalocyanine-based biodegradable polymeric nanoparticles for image-guided combinatorial phototherapy. Chem. Mat. 17, 6155–6165. doi:10.1021/acs.chemmater.5b03128
Tsujimoto, A., Uehara, H., Yoshida, H., Nishio, M., Furuta, K., Inui, T., et al. (2021). Different hydration states and passive tumor targeting ability of polyethylene glycol-modified dendrimers with high and low PEG density. Mater. Sci. Eng. C 126, 112159. doi:10.1016/jmsec.2021.112159
Uthaman, S., Huh, K. M., and Park, I-K. (2018). Tumor microenvironment-responsive nanoparticles for cancer theragnostic applications. Biomater. Res. 22, 22. doi:10.1186/s40824-018-0132-z
Wang, H., and Huang, Y. (2020). Combination therapy based on nano codelivery for overcoming cancer drug resistance. Med. Drug Discov. 6, 100024. doi:10.1016/j.medidd.2020.100024
Wang, S-Y., Hu, H-Z., Qing, X-C., Zhang, Z-C., and Shao, Z-W. (2020). Recent advances of drug delivery nanocarriers in osteosarcoma treatment. J. Cancer 11, 69–82. doi:10.7150/jca.36588
Weber, G. F. (2014). DNA damaging drugs. Mol. Ther. Cancer 8, 9–112. doi:10.1007/978-3-319-13278-5_2
WHO (2022). World Health organization. AvaliableAt: https://www.who.int/news-room/fact-sheets/detail/cancer.
Wu, J. (2021). The enhanced permeability and retention (EPR) effect: The significance of the concept and methods to enhance its application. J. Pers. Med. 11, 771. doi:10.3390/jpm11080771
Xu, K-F., Jia, H-R., Zhu, Y-X., Liu, X., Gao, G., Li, Y-H., et al. (2019). Cholesterol-modified dendrimers for constructing a tumor microenvironment-responsive drug delivery system. ACS Biomater. Sci. Eng. 5, 6072–6081. doi:10.1021/acsbiomaterials.9b01386
Yao, Y., Zhou, Y., Liu, L., Xu, Y., Chen, Q., Wang, Y., et al. (2020). Nanoparticle-based drug delivery in cancer therapy and its role in overcoming drug resistance. Front. Mol. Biosci. 7, 193. doi:10.3389/fmolb.2020.00193
Yoo, J., Park, C., Yi, G., Lee, D., and Koo, H. (2019). Active targeting strategies using biological ligands for nanoparticle drug delivery systems. Cancers (Basel). 11, 640. doi:10.3390/cancers11050640
Yu, B., Tai, H. C., Xue, W., Lee, L. J., and Lee, R. J. (2010). Receptor-targeted nanocarriers for therapeutic delivery to cancer. Mol. Membr. Biol. 27, 286–298. doi:10.3109/09687688.2010.521200
Zhang, K., Zhang, Y., Meng, X., Lu, H., Chang, H., Dong, H., et al. (2018). Light-triggered theranostic liposomes for tumor diagnosis and combined photodynamic and hypoxia-activated prodrug therapy. Biomaterials 185, 301–309. doi:10.1016/j.biomaterials.2018.09.033
Zhang, M., Du, Y., Wang, S., and Chen, B. (2020). A review of biomimetic nanoparticle drug delivery systems based on cell membranes. Drug Des. devel. Ther. 14, 5495–5503. doi:10.2147/dddt.s282368
Zhang, X., Liu, J., Li, X., Li, F., Lee, R. J., Sun, F., et al. (2019). Trastuzumab-coated nanoparticles loaded with docetaxel for breast cancer therapy. Dose-Response 17, 155932581987258. doi:10.1177/1559325819872583
Zhu, R., Wang, Z., Liang, P., He, X., Zhuang, X., Huang, R., et al. (2017). Efficient VEGF targeting delivery of DOX using Bevacizumab conjugated SiO2@LDH for anti-neuroblastoma therapy. Acta Biomater. 63, 163–180. doi:10.1016/j.actbio.2017.09.009
Keywords: nanocarriers, photodynamic therapy, photosensitizers, targeted drug delivery, cancer, surface modification
Citation: Dhilip Kumar SS and Abrahamse H (2022) Recent advances in the development of biocompatible nanocarriers and their cancer cell targeting efficiency in photodynamic therapy. Front. Chem. 10:969809. doi: 10.3389/fchem.2022.969809
Received: 15 June 2022; Accepted: 25 July 2022;
Published: 15 August 2022.
Edited by:
Tien Duc Pham, Vietnam National University, VietnamReviewed by:
Dongfang Zhou, Southern Medical University, ChinaJunjie Li, Innovation Centre of Nano Medicine (iCONM), Japan
Copyright © 2022 Dhilip Kumar and Abrahamse. This is an open-access article distributed under the terms of the Creative Commons Attribution License (CC BY). The use, distribution or reproduction in other forums is permitted, provided the original author(s) and the copyright owner(s) are credited and that the original publication in this journal is cited, in accordance with accepted academic practice. No use, distribution or reproduction is permitted which does not comply with these terms.
*Correspondence: Sathish Sundar Dhilip Kumar, c2F0aGlzaGRAdWouYWMuemE=