- 1School of Chemistry and Chemical Engineering, Nantong University, Nantong, China
- 2Department of Chemistry, Southern University of Science and Technology, Shenzhen, China
Magnetocaloric effect (MCE) is one of the most promising features of molecular-based magnetic materials. We reported three Gd-based magnetic refrigerant materials, namely, Gd2(L)(NO3)(H2O)‧CH3CN‧H2O (1, H2L = (Z)-N-[(1E)-(2-hydroxy-3-methphenyl)methylidene]pyrazine-2-carbohydrazonic acid), {Gd6(L)6(CO3)2(CH3OH)2(H2O)3Cl}Cl‧4CH3CN (2), and Gd8(L)8(CO3)4(H2O)8‧2H2O (3). Complex 1 contains two GdIII ions linked by two η2:η1:η1:η1:μ2-L2- ligands, which are seven-coordinated in a capped trigonal prism, and complex 2 possesses six GdIII ions, contributing to a triangular prism configuration. For complex 3, eight GdIII ions form a distorted cube arrangement. Moreover, the large values of magnetic entropy in the three complexes prove to be excellent candidates as cryogenic magnetic coolants.
Introduction
Ln-based complexes play a critical role in molecular-based materials not only due to the charming geometrical structures but also because of the extensive applications such as luminescence, catalysis, especially for magnetic materials including magnetocaloric effect (MCE) (Wu D et al., 2020; Shang et al., 2021; Wei et al., 2021), and single-molecule magnets (SMMs) (Liu et al., 2014; Liu et al., 2016; Zhang and Cheng, 2016; Reis, 2020). As a member of the Ln elements, the Gd ion is a perfect candidate in the synthesis of molecular-based magnetic refrigeration materials because of the large magnetothermal effects (Evangelisti et al., 2011; Chen et al., 2013; Chen et al., 2014; Wang et al., 2020a; Li et al., 2021; Lin et al., 2021; Wu T et al., 2021; Zhou et al., 2021). Some of the reported magnetic materials even possess a large cryogenic MCE, which is comparable to that of the commercial coolant {Gd3Ga5O12} (Pecharsky and Gschneidner, 1997; Zhang S. et al., 2015; Zhang S.,-W. et al., 2015).
It is worth mentioning that in the pure 4f system, improving magnetic density is the ideal method to gain MCE performance (Zhang et al., 2016; Reis, 2020). Therefore, organic ligands play an important role in the building units of the complexes. In previous studies, various organic ligands (e. g. Schiff-based ligands (Aronica et al., 2006; Boulon et al., 2013; Mannini et al., 2014; Burgess et al., 2015; Nava et al., 2015; Wang et al., 2015; Lakma et al., 2019; Li et al., 2019; Wang et al., 2020b; Wang J. et al., 2021; Wang M. et al., 2021), carboxylates (Milios et al., 2007; Dermitzaki et al., 2015; Yin et al., 2015; Botezat et al., 2017; Feltham et al., 2017; Li et al., 2019; Zheng et al., 2020; Han et al., 2021; Zhou et al., 2021), diketones (Zhu et al., 2014; Yao et al., 2018; Wang et al., 2019a; Wang et al., 2019b; Shi et al., 2021), and diamines (Neves et al., 1992; Zhang et al., 2013; Cornia et al., 2014; Oyarzabal et al., 2014; Feltham et al., 2015; Luan et al., 2015; Lu et al., 2019) etc.) have been successfully utilized in the synthesis of MCE materials. Among them, Schiff-based ligands comprise rich O and N sites, which are widely used in the synthesis of many Ln complexes because of the simple synthesis and structural diversity.
In this work, three Gd-based magnetic refrigerant materials based on Schiff-based ligands (Z)-N-[(1E)-(2-hydroxy-3-methphenyl) methylidene]pyrazine-2-carbohydrazonic acid (H2L) were synthesized, namely, Gd2(L) (NO3) (H2O)‧CH3CN‧H2O (1), {Gd6(L)6(CO3)2(CH3OH)2(H2O)3Cl}Cl‧4CH3CN (2), and Gd8(L)8(CO3)4(H2O)8‧2H2O (3). Magnetic studies indicate that all complexes exhibit antiferromagnetic interactions between the spin centers and display large magnetic entropies.
Materials and methods
Materials
All reactions and manipulations were performed in the ambient atmosphere. The Schiff-based H2L ligand was prepared by condensation with o-vanillin and hydrazine-2-carbohydrazide in methanol according to the literature (Chandrasekhar et al., 2013; Chen et al., 2016). Metal salts and other reagents were commercially available and used without further purification.
Synthesis
Synthesis of Gd2(L)2(NO3)2(H2O)2‧CH3CN‧H2O (1): a mixture of H2L (0.1 mmol, 27.2 mg) and Gd(NO3)3·6H2O (0.1 mmol, 45.7 mg) was dissolved in CH3CN (5 ml) and CH3OH (2.5 ml). After stirring for 5 min, pyridine (0.04 ml) was added and stirred for another 10 min. The solution was filtered and left to slowly evaporate. Well-shaped orange crystals were obtained after 1 week. Yield: 20 mg, 36% based on Gd. Elemental analysis (EA) calc. (%) for Gd2C30H30N12O16, C: 31.91, H: 2.68, N: 14.89; found (%), C: 32.03, H: 2.61, N: 14.93.
{Gd6(L)6(CO3)2(CH3OH)2(H2O)3Cl}Cl‧4CH3CN (2): a mixture of H2L (0.2 mmol, 54.4 mg) and GdCl3.6H2O (0.2 mmol, 74.3 mg) was dissolved in CH3CN (10 ml) and CH3OH (5 ml). After stirring for 5 min, NaHCO3 (0.2 mmol, 33.6 mg) was added and stirred for another 3 h. Well-shaped orange crystals were obtained after 1 week. Yield: 32 mg, 32% based on Gd. Elemental analysis (EA) calc. (%) for Gd6C90H92N28O29Cl2, C: 35.51, H: 3.05, N: 12.88; found (%), C: 35.72, H: 2.99, N: 12.92.
Gd8(L)8(CO3)4(H2O)8‧2H2O (3): a mixture of H2L (0.2 mmol, 13.6 mg) and GdCl3.6H2O (0.2 mmol, 18.6 mg) was dissolved in CH3CN (5 ml) and CH3OH (2.5 ml). After stirring for 5 min, NaCO3 (0.2 mmol, 10.6 mg) was added and stirred for another 2 h. Well-shaped orange crystals were obtained after 1 week. Yield: 28 mg, 29% based on Gd. Elemental analysis (EA) calc. (%) for Gd8C108H100N32O46, C: 33.78, H: 2.62, N: 11.67; found (%), C: 33.83, H: 2.51, N: 11.84.
Physical measurements
The C, H, and N elemental analyses were performed using an Elementar Vario-EL CHNS elemental analyzer. The Fourier transform-infrared (FT-IR) spectra were carried out from KBr pellets in the range 4,000–400 cm−1 using an EQUINOX 55 spectrometer. Powder X-ray diffraction (PXRD) patterns were performed using the Bruker D8 Advance diffractometer (Cu–Kα, λ = 1.54056 Å). Magnetic susceptibility measurements were measured with a Quantum Design MPMS-XL7 SQUID. Polycrystalline samples were embedded in vaseline to prevent torquing. Data were corrected for the diamagnetic contribution calculated from Pascal constants.
Crystallographic study
Suitable single crystals for 1–3 were selected for single-crystal X-ray diffraction analysis. Data were collected using a Rigaku Oxford diffractometer with a Mo–Kα radiation (λ = 0.71073 Å) at 120 K. The structures were solved by direct methods and refined by least-squares on F2 utilizing the SHELXTL program suite and Olex2 (Dolomanov et al., 2009; Sheldrick, 2015a,b). The hydrogen atoms were set in calculated positions and refined as riding atoms with common fixed isotropic thermal parameters. EA was used to detect the content of C, H, and N atoms. Detailed information about the crystal data and structure refinements is summarized in Table 1. Selected bond lengths and angles of complexes 1–3 are listed in Supplementary Table S1–S3.
Results and discussion
Description of the structures of 1–3
Complexes 1–3 are synthesized by the evolution method with H2L and gadolinium salt in the solution of CH3CN/CH3OH (V1:V2 = 2:1) under the existence of alkali. The alkali is added to be conducive to protonate the ligand H2L, which is beneficial to incorporate GdIII ions. The H2L ligand in all complexes is completely dehydrogenated adopting the μ2:η2:η1:η1:η1-mode (Scheme 1A), which is similar to the reported literature (Chandrasekhar et al., 2013; Chen et al., 2016; Zhang et al., 2017; Zhang et al., 2016; Jiang et al., 2016).
Complex 1 is crystalized in the triclinic P-1 space group. As shown in Figure 1, the crystallography independent unit of 1 contains half of the molecule, including one GdIII ion, one L2- ligand, one NO3− anion, and half of CH3CN and H2O molecules. The metallic GdIII ions (Gd1 and Gd1A) are surrounded by two L2- ligands using the aforementioned mode, two NO3− anions and two H2O molecules located above and below the plane, respectively. The average bond lengths of Gd-O and Gd-N are 2.379 (5) Å and 2.460 (5) Å (Supplementary Table S1), respectively, which are in accordance with those of the reported Gd-based complexes (Chen et al., 1995; Zhao et al., 2017; Mayans and Escuer, 2021; Ren et al., 2021). In complex 1, the Gd ion is seven-coordinated to form a capped trigonal prism, which is confirmed by CShM calculations (Alvarez et al., 2005; Casanova et al., 2005) (Supplementary Figure S1, Supplementary Table S4).
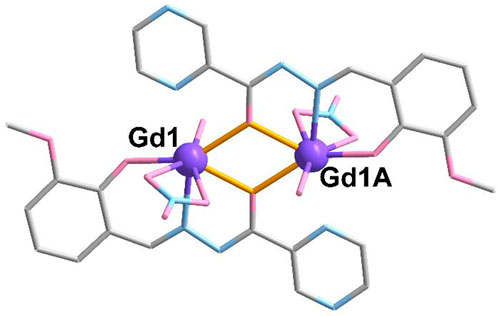
FIGURE 1. Crystal structure of complex 1. The hydrogen atoms are omitted for clarity. Color codes: Gd, purple; O, pink; N, blue; and C, grey. Symmetric code: A, 1-x, 1-year, and 1-z.
Complex 2 crystalizes in the same space group as complex 1, and the asymmetric unit comprises the whole molecule with six crystallographically independent GdIII ions (Figure 2A). The six Gd ions are held together to form a {Gd6} triangular prism metallic skeleton (Figure 2B). Therein, three Gd ions in the plane (Gd1, Gd2, and Gd3 or Gd4, Gd5, and Gd6) contribute a triangular configuration, which are bridged by one CO32- anion in μ3-η2:η2:η2-mode (Scheme 1B). The two triangular metallic skeletons are then linked together by six μ2-O bridges from ligands.
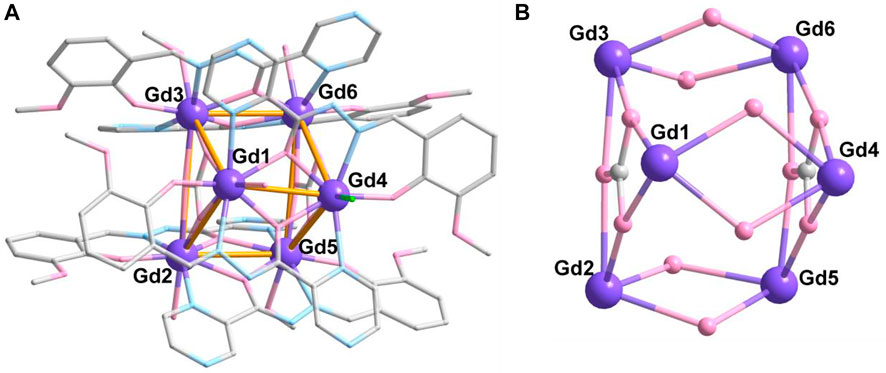
FIGURE 2. Crystal structure (A) and metallic core (B) of complex 2. The hydrogen atoms are omitted for clarity. Color codes: Gd, purple; O, pink; N, blue; and C, gray.
All Gd ions are eight coordinated, showing two kinds of coordination geometry confirmed by CShM calculations (Alvarez et al., 2005; Casanova et al., 2005) (Supplementary Table S5). The Gd1, Gd2, Gd3, Gd5, and Gd6 ions are in {O6N2} environment with six O atoms and two N atoms from two chelated L2- ligands, one CO32- anion and one CH3OH/H2O molecule, which display a biaugmented trigonal prism configuration (Supplementary Figure S2). The average Gd-O and Gd-N distances are 2.352 (4) Å and 2.475 (4) Å, respectively (Supplementary Table S2), which are consistent with those reported Gd-based complexes (Chen et al., 1995; Zhao et al., 2017; Mayans and Escuer, 2021; Ren et al., 2021). However, Gd4 has triangular dodecahedron coordination geometry and is located in an {O5N2Cl} environment with five O and two N atoms from two chelated L2- ligands and one Cl− anion. The bond length of Gd4-Cl1 is 2.746 (1) Å, which is longer than that of Gd-O and Gd-N.
For complex 3, the synthetic method is the same as complex 2; except NaHCO3 was used in place of Na2CO3. Surprisingly, complex 3 possesses an octa-nuclearity structure, which crystalizes in the triclinic P-1 space group. The asymmetric unit consists of a completed molecule, and there are eight crystallographically independent Gd atoms in the molecular structure (Figure 3A). As shown in Figure 3B, the eight GdIII ions contribute to a cubic trapezoid metallic core. Gd1, Gd4, Gd5, and Gd8 ions lie in the four vertices of the plane below the cubic trapezoid, while Gd2, Gd3, Gd6, and Gd7 ions situate in the upper plane. The metallic core is held together by four CO32- anions in μ3-η2:η2:η1-mode (Scheme 1C). The periphery of the metal core is ligated by eight L2- ligands, eight H2O molecules, and two lattice H2O molecules.
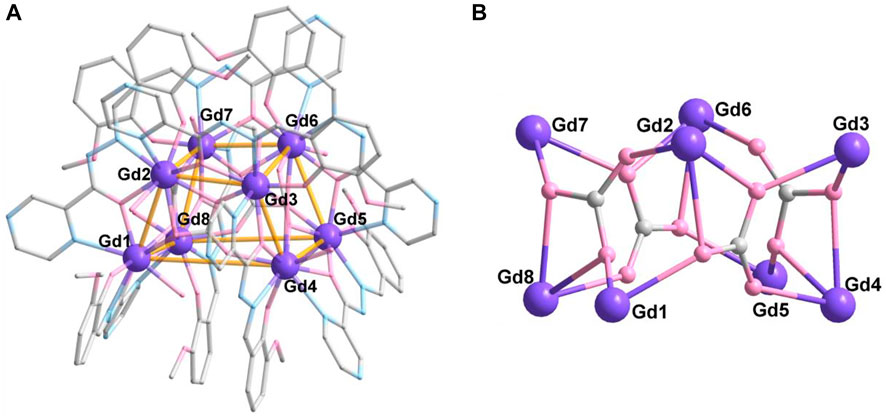
FIGURE 3. Crystal structure (A) and metallic core (B) of 3. The hydrogen atoms are omitted for clarity. Color codes: Gd, purple; O, pink; N, blue; and C, gray.
There are two coordination numbers of GdIII ions in complex 3 (Supplementary Figure S3). Gd1, Gd3, Gd5, and Gd7 are eight-coordinated ions in {O6N2} donor set from two L2- ligands, two CO32- anions, and one H2O molecule, while Gd2, Gd4, Gd6, and Gd8 ions are nine-coordinated in the {O7N2} donor set. The difference between the two kinds of Gd ions is the diverse coordination modes of the CO32- anion. There is only one coordination bond of O atom in CO32- anion, which is adopted in Gd1, Gd3, Gd5, and Gd7 ions. For Gd2, Gd4, Gd6, and Gd8 ions, the bonding mode of the CO32- anion is adopted in the bidentate mode. The eight metal ions exhibit three coordination geometries: biaugmented trigonal prism (Gd1), triangular dodecahedron (Gd3, Gd5, and Gd7), and muffin (Gd2, Gd4, Gd6, and Gd8) (Supplementary Tables S5,6). The average Gd-O distance is 2.361 (4) Å, which is shorter than that of Gd-N (2.564 (4) Å) lengths. The O/N-Gd-O/N angles are in the range of 60.99°–154.86°, which are in the normal range (Chen et al., 1995; Zhao et al., 2017; Mayans and Escuer, 2021; Ren et al., 2021).
It is worth mentioning that the use of different alkalis can affect the number of formed metal nuclearity. For the organic weak alkali triethylamine, which is used in complex 1, it only facilitates protonation of the ligand H2L but is not involved in the final formation of complex 1. However, for complexes 2 and 3, the inorganic alkalis not only deprotonate the ligand but also participate in the construction of the molecules. Compared to NaHCO3 in complex 2, the alkalinity of Na2CO3 is relatively strong. Moreover, mainly due to the degree of hydrolysis of carbonates being higher, there are more carbonate triangle skeletons in complex 3, making it easier to coordinate with Gd ions, thus forming an octa-nuclearity complex.
IR spectra and PXRD studies
The FT-IR spectra of complexes 1–3 were acquired (v = 4,000–500 cm−1), which are shown in Supplementary Figure S4. Powder X-ray diffraction (PXRD) measurements for complexes 1–3 were performed for the crystalline crystals (Supplementary Figure S5), and the experimental patterns are in good agreement with the simulated ones from the crystallographic data. The minor inconsistencies in the intensity and shape of the peaks indicate the phase purity of complexes 1–3.
Magnetic studies
The direct current magnetic susceptibilities of complexes 1–3 were studied for polycrystalline samples in the temperature range of 2–300 K at an external magnetic field of 1000 Oe (Figure 4A). At room temperature, the χMT values of complexes 1–3 are 15.77, 47.16, and 62.81 cm3 K mol−1, respectively, which is in good agreement with the expected spin-only values (GdIII ion: 7.875 cm3 K mol−1, g = 2). Upon cooling, the χMT values in all cases stay essentially unchanged until approximately 25 K and then followed by an obvious decrease to the minimum values of 13.29, 38.46, and 58.30 cm3 K mol−1, indicating antiferromagnetic interactions (Kahn et al., 2000). Fitting the curve of χM−1 vs. T with the Curie–Weiss Law (Figure 4B) gives the resulting C and θ values, which are listed in Supplementary Table S7. The negative θ values imply the presence of weak antiferromagnetic interaction within complexes 1–3.
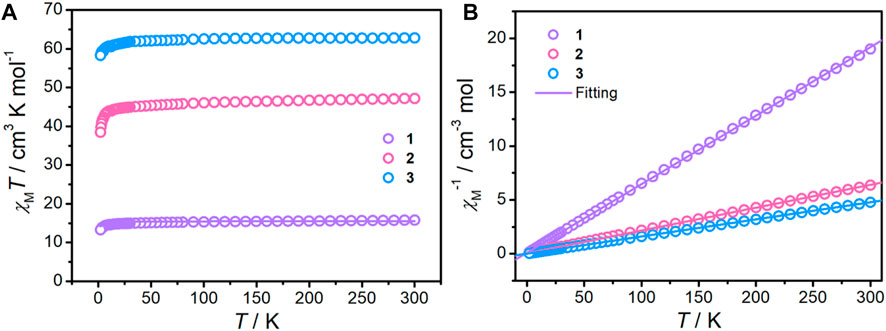
FIGURE 4. χMT products measured under a 1000 Oe DC applied field (A) and the plots of 1/χM vs. T (B) for complexes 1–3. The solid lines represent the best fitting.
The field dependence of the magnetization plots for complexes 1–3 was performed in the field range of 1–7 T at 2–8 K (Supplementary Figure S6). Magnetizations in all complexes are increased gradually at the entire field region, reaching saturation values of 13.81, 41.75, and 55.83 NμB at 7 T and 2 K, respectively, close to the theoretical value (1: 14 NμB; 2: 42 NμB; 3: 56 NμB). The reduced magnetization plots (M vs. HT−1) in all complexes are superposable due to the isotropic system (Supplementary Figure S7).
Due to the complicated systems in complexes 2 and 3, only complex 1 is attempted to analyze the magnetic interactions by using a simplified spin Hamiltonian with the PHI program (Eq. 1):
The best-fit parameters are J = -0.022 (2) cm−1 and g = 1.98 (Figure 4A; Supplementary Figure S8). The negative J value confirms the antiferromagnetic interactions between the GdIII ions, which is in accordance with the trend of the χMT product with cooling and the result of the Curie–Weiss Law.
The isothermal magnetization for complexes 1–3 was measured from 2 to 8 K in an applied DC field up to 7 T to calculate the magnetic entropy (-∆Sm) according to the Maxwell equation (Pecharsky and Gschneidner, 1999) (Eq. 2). It can be seen that the curves of -∆Sm of complexes 1–3 gradually increase with decreasing temperature and increasing of magnetic field without saturation, the maximum -∆Sm values are 25.05 J kg−1 K−1, 27.21 J kg−1 K−1, and 30.79 J kg−1 K−1 at 2 K, ∆H = 7 T, respectively (Figure 5). These values are smaller than the theoretical values of 34.57 J kg−1 K−1 for 1, 34.07 J kg−1 K−1 for 2, and 36.01 J kg−1 K−1 for 3, which are calculated using Eq 3, (n = 2, 6, and 8 for 1, 2, and 3, respectively; S = 7/2 and the R value is 8.314 J mol−1 K−1), owing to the existence of antiferromagnetic coupling. The maximum -∆Sm of 1 in di-nuclearity complex is among the highest observed to date for 4f clusters appeared at low temperature (Table 2). Although complexes 2 and 3 do not possess the highest -∆Sm values, they are still comparable in the same nuclear complexes.
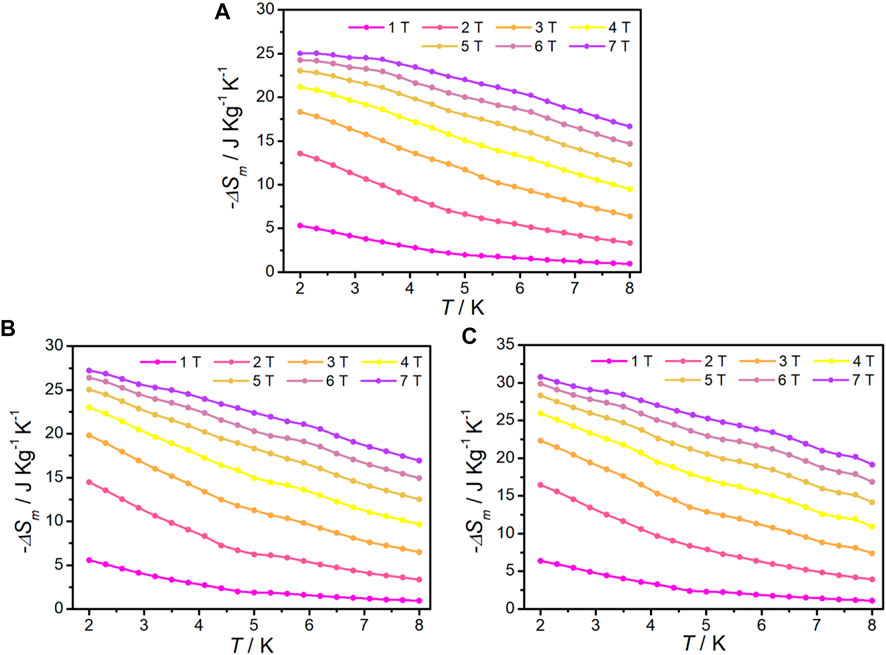
FIGURE 5. -∆Sm at various fields and temperatures, calculated from the magnetization data for 1(A), 2(B), and 3(C).
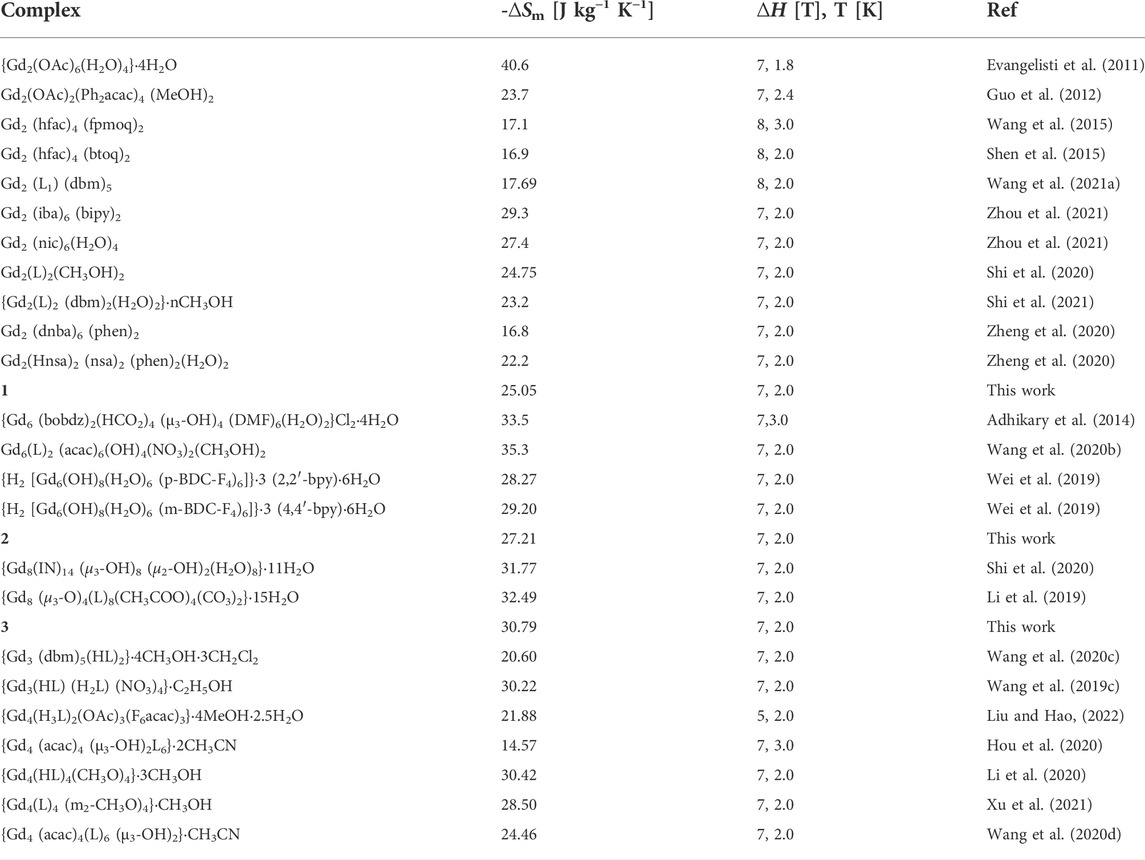
TABLE 2. Summary of -∆Sm in different ∆H at a given temperature for reported di-nuclearity, hexa-nuclearity, octa-nuclearity, and other multinuclear Gd-based complexes.
Conclusion
In conclusion, three clusters 1-{Gd2}, 2-{Gd6}, and 3-{Gd8} based on Schiff ligand H2L were synthesized. Complex 1 contains two GdIII ions, and magnetic measurement indicates antiferromagnetic interactions between the metal core, which is also confirmed by PHI fitting. Complexes 2 and 3 are hexa-nuclearity with a biaugmented trigonal prism configuration and octa-nuclearity with a cubic trapezoid structure. Magnetic investigations indicate the antiferromagnetic interactions between GdIII ions are observed in complexes 2 and 3. Magnetocaloric studies for complexes 1–3 show that the magnetic entropies of complexes 1–3 are smaller than the theoretical values, which is mainly caused by antiferromagnetic coupling. Furthermore, complex 1 exhibits a large magnetic entropy of 25.05 J kg−1 K−1 at 2.0 K in di-nuclearity magnetic refrigerant materials, while complexes 2 and 3 belong to the normal range in hexa-nuclearity and octa-nuclearity complexes, respectively, demonstrating that they are promising molecular magnetic coolants for low-temperature cooling applications.
Data availability statement
The datasets presented in this study can be found in online repositories. The names of the repository/repositories and accession number(s) can be found in the article/Supplementary Material.
Author contributions
MW and CS: writing—original draft; YG, HX, LH, and YZ: investigation and formal analysis; JW: project administration and funding acquisition; YP: measurement; YT: validation, editing, and funding acquisition.
Funding
This work was supported by the National Natural Science Foundation of China (Nos. 22075152 and 22101144), the Natural Science Foundation of Jiangsu Province (No. BK20210835), and the Science and Technology Project Fund of Nantong (Nos. JC2020130, JC2020133, and JC2020134).
Acknowledgments
We are very grateful to the Nantong University Analytical Testing Center for its support for testing.
Conflict of interest
The authors declare that the research was conducted in the absence of any commercial or financial relationships that could be construed as a potential conflict of interest.
Publisher’s note
All claims expressed in this article are solely those of the authors and do not necessarily represent those of their affiliated organizations, or those of the publisher, the editors, and the reviewers. Any product that may be evaluated in this article, or claim that may be made by its manufacturer, is not guaranteed or endorsed by the publisher.
Supplementary material
The Supplementary Material for this article can be found online at: https://www.frontiersin.org/articles/10.3389/fchem.2022.963203/full#supplementary-material
References
Adhikary, A., Sheikh, J. A., Biswas, S., and Konar, S. (2014). Synthesis, crystal structure and study of magnetocaloric effect and single molecular magnetic behaviour in discrete lanthanide complexes. Dalton Trans. 43, 9334–9343. doi:10.1039/C4DT00540F
Alvarez, S., Alemany, P., Casanova, D., Cirera, J., Llunell, M., and Avnir, D. (2005). Shape maps and polyhedral interconversion paths in transition metal chemistry. Coord. Chem. Rev. 249, 1693–1708. doi:10.1016/j.ccr.2005.03.031
Aronica, C., Pilet, G., Chastanet, G., Wernsdorfer, W., Jacquot, J.-F., and Luneau, D. (2006). A nonanuclear dysprosium(III)–copper(II) complex exhibiting single‐molecule magnet behavior with very slow zero‐field relaxation. Angew. Chem. Int. Ed. 45, 4659–4662. doi:10.1002/anie.200600513
Botezat, O., van Leusen, J., Kravtsov, V. C., Kogerler, P., and Baca, S. G. (2017). Ultralarge 3d/4f coordination wheels: From carboxylate/amino alcohol-supported {Fe4Ln2} to {Fe18Ln6} rings. Inorg. Chem. 56, 1814–1822. doi:10.1021/acs.inorgchem.6b02100
Boulon, M. E., Cucinotta, G., Luzon, J., Degl'Innocenti, C., Perfetti, M., Bernot, K., et al. (2013). Magnetic anisotropy and spin-parity effect along the series of lanthanide complexes with DOTA. Angew. Chem. Int. Ed. 52, 350–354. doi:10.1002/anie.201205938
Burgess, J. A., Malavolti, L., Lanzilotto, V., Mannini, M., Yan, S., Ninova, S., et al. (2015). Magnetic fingerprint of individual Fe4 molecular magnets under compression by a scanning tunnelling microscope. Nat. Commun. 6, 8216–8222. doi:10.1038/ncomms9216
Casanova, D., Llunell, M., Alemany, P., and Alvarez, S. (2005). The rich stereochemistry of eight-vertex polyhedra: A continuous shape measures study. Chem. Eur. J. 11, 1479–1494. doi:10.1002/chem.200400799
Chandrasekhar, V., Das, S., Dey, A., Hossain, S., and Sutter, J.-P. (2013). Tetranuclear lanthanide (III) complexes containing dimeric subunits: Single-molecule magnet behavior for the Dy4 analogue. Inorg. Chem. 52, 11956–11965. doi:10.1021/ic401652f
Chen, Q., Ma, F., Meng, Y.-S., Sun, H.-L., Zhang, Y.-Q., and Gao, S. (2016). Assembling dysprosium dimer units into a novel chain featuring slow magnetic relaxation via formate linker. Inorg. Chem. 55, 12904–12911. doi:10.1021/acs.inorgchem.6b02276
Chen, X.-M., Aubin, S. M. J., Wu, Y.-L., Yang, Y.-S., Mak, T. C. W., and Hendrickson, D. N. (1995). Polynuclear CuII12MIII6 (M = Y, Nd, or Gd) complexes encapsulating a ClO4- anion: [Cu12M6(OH)24(H2O)18(pyb)12(ClO4)](ClO4)17·nH2O (pyb = pyridine betaine). J. Am. Chem. Soc. 117, 9600–9601. doi:10.1021/ja00142a043
Chen, Y.-C., Guo, F.-S., Zheng, Y.-Z., Liu, J.-L., Leng, J.-D., Tarasenko, R., et al. (2013). Gadolinium(III)-hydroxy ladders trapped in succinate frameworks with optimized magnetocaloric effect. Chem. Eur. J. 19, 13504–13510. doi:10.1002/chem.201301221
Chen, Y.-C., Qin, L., Meng, Z.-S., Yang, D.-F., Wu, C., Fu, Z., et al. (2014). Study of a magnetic-cooling material Gd(OH)CO3. J. Mat. Chem. A 2, 9851–9858. doi:10.1039/C4TA01646G
Cornia, A., Rigamonti, L., Boccedi, S., Clerac, R., Rouzieres, M., and Sorace, L. (2014). Magnetic blocking in extended metal atom chains: A pentachromium(II) complex behaving as a single-molecule magnet. Chem. Commun. 50, 15191–15194. doi:10.1039/c4cc06693f
Dermitzaki, D., Raptopoulou, C. P., Psycharis, V., Escuer, A., Perlepes, S. P., and Stamatatos, T. C. (2015). Nonemployed simple carboxylate ions in well-investigated areas of heterometallic carboxylate cluster chemistry: A new family of {CuII4LnIII8} complexes bearing tert-butylacetate bridging ligands. Inorg. Chem. 54, 7555–7561. doi:10.1021/acs.inorgchem.5b01179
Dolomanov, O. V., Bourhis, L. J., Gildea, R. J., Howard, J. A. K., and Puschmann, H. (2009). OLEX2: A complete structure solution, refinement and analysis program. J. Appl. Crystallogr. 42, 339–341. doi:10.1107/S0021889808042726
Evangelisti, M., Roubeau, O., Palacios, E., Camón, A., Hooper, T. N., Brechin, E. K., et al. (2011). Cryogenic magnetocaloric effect in a ferromagnetic molecular dimer. Angew. Chem. Int. Ed. 50, 6606–6609. doi:10.1002/anie.201102640
Feltham, H. L. C., Dhers, S., Rouzières, M., Clérac, R., Powell, A. K., and Brooker, S. (2015). A family of fourteen soluble stable macrocyclic [NiIILnIII] heterometallic 3d–4f complexes. Inorg. Chem. Front. 2, 982–990. doi:10.1039/c5qi00130g
Feltham, H. L. C., Dumas, C., Mannini, M., Otero, E., Sainctavit, P., Sessoli, R., et al. (2017). Proof of principle: Immobilisation of robust CuII 3 TbIII-macrocycles on small, suitably pre-functionalised gold nanoparticles. Chem. Eur. J. 23, 2517–2521. doi:10.1002/chem.201604821
Guo, F.-S., Leng, J.-D., Liu, J.-L., Meng, Z.-S., and Tong, M.-L. (2012). Polynuclear and polymeric gadolinium acetate derivatives with large magnetocaloric effect. Inorg. Chem. 51, 405–413. doi:10.1021/ic2018314
Han, L.-J., Wang, W.-M., Wang, X.-W., Huai, L., Qiao, N., and Fang, M. (2021). A rhombic shaped {GdIII2CoII2} heterometallic cluster exhibiting larger cryogenic magnetocaloric effect. Inorganica Chim. Acta 514, 120020–120024. doi:10.1016/j.ica.2020.120020
Hou, Y.-L., Han, X., Hu, X.-Y., Shen, J.-X., Wang, J., Shi, Y., et al. (2020). Two LnIII4(LnIII = Gd and Dy) clusters constructed by 8-hydroxyquinoline schiff base and β-diketonate coligand: Magnetic refrigeration property and single-molecule magnet behavior. Inorganica Chim. Acta 502, 119290–119303. doi:10.1016/j.ica.2019.119290
Jiang, Y., Brunet, G., Holmberg, R. J., Habib, F., Korobkov, I., and Murugesu, M. (2016). Terminal solvent effects on the anisotropy barriers of Dy2 systems. Dalton Trans. 45, 16709–16715. doi:10.1039/C6DT03366K
Kahn, M. L., Sutter, J.-P., Golhen, S., Guionneau, P., Ouahab, L., Kahn, O., et al. (2000). Systematic investigation of the nature of the coupling between a ln(III) ion (ln = Ce(III) to Dy(III)) and its aminoxyl radical ligands. Structural and magnetic characteristics of a series of {Ln(organic radical)2} compounds and the related {Ln(Nitrone)2} derivatives. J. Am. Chem. Soc. 122, 3413–3421. doi:10.1021/ja994175o
Lakma, A., Hossain, S. M., van Leusen, J., Kögerler, P., and Singh, A. K. (2019). Tetranuclear MnII, CoII, CuII and ZnII grid complexes of an unsymmetrical ditopic ligand: Synthesis, structure, redox and magnetic properties. Dalton Trans. 48, 7766–7777. doi:10.1039/C9DT01041F
Li, J. N., Li, N. F., Wang, J. L., Liu, X. M., Ping, Q. D., Zang, T. T., et al. (2021). A new family of boat-shaped Ln8 clusters exhibiting the magnetocaloric effect and slow magnetic relaxation. Dalton Trans. 50, 13925–13931. doi:10.1039/d1dt02390j
Li, L.-F., Kuang, W.-W., Li, Y.-M., Zhu, L.-L., Xu, Y., and Yang, P.-P. (2019). A series of new octanuclear Ln8 clusters: Magnetic studies reveal a significant cryogenic magnetocaloric effect and slow magnetic relaxation. New J. Chem. 43, 1617–1625. doi:10.1039/C8NJ04231D
Li, L., Yang, L.-R., Qiao, N., Xue, M.-M., Fang, Z.-X., Ren, J., et al. (2020). A novel tetranuclear Gd(III)-based cluster showing larger magnetic refrigeration property. J. Mol. Struct. 1222, 128906–128911. doi:10.1016/j.molstruc.2020.128906
Lin, Q.-F., Ding, L.-L., Xu, Z.-H., Wang, Q.-q., Li, N.-F., Xu, Y., et al. (2021). Three 3D Lanthanide coordination polymers: Synthesis, luminescence and magnetic properties. J. Mol. Struct. 1234, 130167–130173. doi:10.1016/j.molstruc.2021.130167
Liu, C.-M., and Hao, X. (2022). Asymmetric assembly of chiral lanthanide(III) tetranuclear cluster complexes using achiral mixed ligands: Single-molecule magnet behavior and magnetic entropy change. ACS Omega 7, 20229–20236. doi:10.1021/acsomega.2c02155
Liu, J.-L., Chen, Y.-C., Guo, F.-S., and Tong, M.-L. (2014). Recent advances in the design of magnetic molecules for use as cryogenic magnetic coolants. Coord. Chem. Rev. 281, 26–49. doi:10.1016/j.ccr.2014.08.013
Liu, J.-L., Chen, Y.-C., and Tong, M.-L. (2016). Molecular design for cryogenic magnetic coolants. Chem. Rec. 16, 825–834. doi:10.1002/tcr.201500278
Lu, G., Wang, J., Zhang, C.-J., Bala, S., Chen, Y.-C., Huang, G.-Z., et al. (2019). Single-ion magnet and luminescent properties in a Dy(III) triangular dodecahedral complex. Inorg. Chem. Commun. 102, 16–19. doi:10.1016/j.inoche.2019.02.003
Luan, F., Liu, T., Yan, P., Zou, X., Li, Y., and Li, G. (2015). Single-molecule magnet of a tetranuclear dysprosium complex disturbed by a salen-type ligand and chloride counterions. Inorg. Chem. 54, 3485–3490. doi:10.1021/acs.inorgchem.5b00061
Mannini, M., Bertani, F., Tudisco, C., Malavolti, L., Poggini, L., Misztal, K., et al. (2014). Magnetic behaviour of TbPc2 single-molecule magnets chemically grafted on silicon surface. Nat. Commun. 5, 4582–4589. doi:10.1038/ncomms5582
Mayans, J., and Escuer, A. (2021). Correlating the axial zero field splitting with the slow magnetic relaxation in GdIII SIMs. Chem. Commun. 57, 721–724. doi:10.1039/D0CC07474H
Milios, C. J., Inglis, R., Vinslava, A., Bagai, R., Wernsdorfer, W., Parsons, S., et al. (2007). Toward a magnetostructural correlation for a family of Mn6 SMMs. J. Am. Chem. Soc. 129, 12505–12511. doi:10.1021/ja0736616
Nava, A., Rigamonti, L., Zangrando, E., Sessoli, R., Wernsdorfer, W., and Cornia, A. (2015). Redox-controlled exchange bias in a supramolecular chain of Fe4 single-molecule magnets. Angew. Chem. Int. Ed. 54, 8777–8782. doi:10.1002/anie.201500897
Neves, A., Erthal, S. M. D., Vencato, I., Ceccato, A. S., Mascarenhas, Y. P., Nascimento, O. R., et al. (1992). Synthesis, crystal structure, electrochemical, and spectroelectrochemical properties of the new manganese(III) complex [MnIII(BBPEN)] [PF6] [H2BBPEN = N, N'-bis(2-hydroxybenzyl)-N, N'-bis(2-methylpyridyl)ethylenediamine]. Inorg. Chem. 31, 4749–4755. doi:10.1021/ic00049a008
Oyarzabal, I., Ruiz, J., Seco, J. M., Evangelisti, M., Camon, A., Ruiz, E., et al. (2014). Rational electrostatic design of easy-axis magnetic anisotropy in a Zn(II)-Dy(III)-Zn(II) single-molecule magnet with a high energy barrier. Chem. Eur. J. 20, 14262–14269. doi:10.1002/chem.201403670
Pecharsky, V. K., and Gschneidner, J. K. A. (1997). Giant magnetocaloric effect in Gd5{Si2Ge2. Phys. Rev. Lett. 78, 4494–4497. doi:10.1103/PhysRevLett.78.4494
Pecharsky, V. K., and Gschneidner, K. A. (1999). Magnetocaloric effect and magnetic refrigeration. J. Magn. Magn. Mat. 200, 44–56. doi:10.1016/S0304-8853(99)00397-2
Reis, M. S. (2020). Magnetocaloric and barocaloric effects of metal complexes for solid state cooling: Review, trends and perspectives. Coord. Chem. Rev. 417, 213357. doi:10.1016/j.ccr.2020.213357
Ren, J., Wei, X. Q., Xu, R. S., Chen, Z. Y., Wang, J., Wang, M., et al. (2021). A potential ferromagnetic lanthanide‒transition heterometallic molecular‒based bacteriostatic agent. J. Mol. Struct. 1229, 129783. doi:10.1016/j.molstruc.2020.129783
Shang, Y., Cao, Y., Xie, Y., Zhang, S., and Cheng, P. (2021). A 1D Mn-based coordination polymer with significant magnetocaloric effect. Polyhedron 202, 115173–115179. doi:10.1016/j.poly.2021.115173
Sheldrick, G. M. (2015a). Crystal structure refinement with SHELXL. Acta Crystallogr. C Struct. Chem. C71, 3–8. doi:10.1107/S2053229614024218
Sheldrick, G. M. (2015b). Shelxt - integrated space-group and crystal-structure determination. Acta Crystallogr. A Found. Adv. A71, 3–8. doi:10.1107/S2053273314026370
Shen, H.-Y., Wang, W.-M., Bi, Y.-X., Gao, H.-L., Liu, S., and Cui, J.-Z. (2015). Luminescence, magnetocaloric effect and single-molecule magnet behavior in lanthanide complexes based on a tridentate ligand derived from 8-hydroxyquinoline. Dalton Trans. 44, 18893–18901. doi:10.1039/c5dt02894a
Shi, Q.-H., Xue, C.-L., Fan, C.-J., Yan, L.-L., Qiao, N., Fang, M., et al. (2021). Magnetic refrigeration property and slow magnetic relaxation behavior of five dinuclear Ln(III)-based compounds. Polyhedron 194, 114938. doi:10.1016/j.poly.2020.114938
Shi, X.-H., Hao, S.-S., Wang, M.-J., Zhang, L., Liang, W.-H., Gao, H.-M., et al. (2020). Two Ln(III)2 (Ln = Gd and Dy) compounds showing magnetic refrigeration and slow magnetic relaxation. J. Mol. Struct. 1210, 127997. doi:10.1016/j.molstruc.2020.127997
Wang, J., Wu, Z.-L., Yang, L.-R., Xue, M.-M., Fang, Z.-X., Luo, S.-C., et al. (2021a). Two lanthanide-based dinuclear clusters (Gd2 and Dy2) with schiff base derivatives: Synthesis, structures and magnetic properties. Inorganica Chim. Acta 514, 120015. doi:10.1016/j.ica.2020.120015
Wang, M., Lu, L., Song, W., Wang, X., Sun, T., Zhu, J., et al. (2021b). AIE-active Schiff base compounds as fluorescent probe for the highly sensitive and selective detection of Al3+ ions. J. Lumin. 233, 117911. doi:10.1016/j.jlumin.2021.117911
Wang, W.-M., Gao, Y., Yue, R.-X., Qiao, N., Wang, D.-T., Shi, Y., et al. (2020c). Construction of a family of Ln3 clusters using multidentate schiff base and β-diketonate ligands: Fluorescence properties, magnetocaloric effect and slow magnetic relaxation. New J. Chem. 44, 9230–9237. doi:10.1039/d0nj01172j
Wang, W.-M., He, L.-Y., Wang, X.-X., Shi, Y., Wu, Z.-L., and Cui, J.-Z. (2019b). Linear-shaped LnIII 4 and LnIII 6 clusters constructed by a polydentate schiff base ligand and a β-diketone co-ligand: Structures, fluorescence properties, magnetic refrigeration and single-molecule magnet behavior. Dalton Trans. 48, 16744–16755. doi:10.1039/c9dt03478a
Wang, W.-M., Huai, L., Wang, X.-W., Jiang, K.-J., Shen, H.-Y., Gao, H.-L., et al. (2020d). Structures, magnetic refrigeration and single molecule-magnet behavior of five rhombus-shaped tetranuclear Ln(III)-based clusters. New J. Chem. 44, 10266–10274. doi:10.1039/d0nj01969k
Wang, W.-M., Wang, M.-J., Hao, S.-S., Shen, Q.-Y., Wang, M.-L., Liu, Q.-L., et al. (2020a). ‘Windmill’-shaped LnIII 4 (LnIII = Gd and Dy) clusters: Magnetocaloric effect and single-molecule-magnet behavior. New J. Chem. 44, 4631–4638. doi:10.1039/c9nj05317d
Wang, W.-M., Xue, C.-L., Jing, R.-Y., Ma, X., Yang, L.-N., Luo, S.-C., et al. (2020b). Two hexanuclear lanthanide Ln6III clusters featuring remarkable magnetocaloric effect and slow magnetic relaxation behavior. New J. Chem. 44, 18025–18030. doi:10.1039/D0NJ03442H
Wang, W.-M., Yue, R.-X., Gao, Y., Wang, M.-J., Hao, S.-S., Shi, Y., et al. (2019a). Large magnetocaloric effect and remarkable single-molecule-magnet behavior in triangle-assembled LnIII 6 clusters. New J. Chem. 43, 16639–16646. doi:10.1039/C9NJ03921J
Wang, W.-M., Zhang, H.-X., Wang, S.-Y., Shen, H.-Y., Gao, H.-L., Cui, J.-Z., et al. (2015). Ligand field affected single-molecule magnet behavior of lanthanide(III) dinuclear complexes with an 8-hydroxyquinoline Schiff base derivative as bridging ligand. Inorg. Chem. 54, 10610–10622. doi:10.1021/acs.inorgchem.5b01404
Wang, Y. -X., Xu, Q., Ren, P., Shi, W., and Cheng, P. (2019c). Solvent-induced formation of two gadolinium clusters demonstrating strong magnetocaloric effects and ferroelectric properties. Dalton Trans. 48, 2228–2233. doi:10.1039/c8dt04267e
Wei, W., Wang, X., Zhang, K., Tian, C.-B., and Du, S.-W. (2019). Tuning the topology from fcu to pcu: Synthesis and magnetocaloric effect of metal–organic frameworks based on a hexanuclear Gd(III)-hydroxy cluster. Cryst. Growth Des. 19, 55–59. doi:10.1021/acs.cgd.8b01566
Wei, W., Xie, R.-K., Du, S.-W., Tian, C.-B., and Chai, G.-L. (2021). Synthesis, structure, magnetocaloric effect and DFT calculations of a MnII cluster-based inorganic coordination polymer. J. Alloys Compd. 878, 160353–160361. doi:10.1016/j.jallcom.2021.160353
Wu, D. Q., Zhang, M. L., Li, Z. Y., Zhang, P. P., Zhang, M. Y., Wu, C. F., et al. (2021). A two dimensional GdIII coordination polymer based on isonicotinic acid N‐oxide with large magnetocaloric effect. Z. Anorg. Allg. Chem. 647, 1542–1546. doi:10.1002/zaac.202100033
Wu, T. X., Tao, Y., He, Q. J., Li, H. Y., Bian, H. D., and Huang, F. P. (2021). Constructing an unprecedented {MnII 38} matryoshka doll with a [Mn 18(CO3 ) 9] inorganic core and magnetocaloric effect. Chem. Commun. 57, 2732–2735. doi:10.1039/d0cc07884k
Xu, C., Wu, Z., Fan, C., Yan, L., Wang, W., and Ji, B. (2021). Synthesis of two lanthanide clusters LnIII 4 (Gd 4 and Dy 4) with [2×2] square grid shape: Magnetocaloric effect and slow magnetic relaxation behaviors. J. Rare Earths 39, 1082–1088. doi:10.1016/j.jre.2020.08.015
Yao, X., Yan, P., An, G., Li, Y., Li, W., and Li, G. (2018). Investigation of magneto-structural correlation based on a series of seven-coordinated beta-diketone Dy(iii) single-ion magnets with C2v and C3v local symmetry. Dalton Trans. 47, 3976–3984. doi:10.1039/c7dt04764a
Yin, D.-D., Chen, Q., Meng, Y.-S., Sun, H.-L., Zhang, Y.-Q., and Gao, S. (2015). Slow magnetic relaxation in a novel carboxylate/oxalate/hydroxyl bridged dysprosium layer. Chem. Sci. 6, 3095–3101. doi:10.1039/c5sc00491h
Zhang, L., Jung, J., Zhang, P., Guo, M., Zhao, L., Tang, J., et al. (2016). Site-resolved two-step relaxation process in an asymmetric Dy2 single-molecule magnet. Chem. Eur. J. 22, 1392–1398. doi:10.1002/chem.201503422
Zhang, L., Zhang, Y.-Q., Zhang, P., Zhao, L., Guo, M., and Tang, J. (2017). Single-molecule magnet behavior enhanced by synergic effect of single-ion anisotropy and magnetic interactions. Inorg. Chem. 56, 7882–7889. doi:10.1021/acs.inorgchem.7b00625
Zhang, P., Zhang, L., Lin, S.-Y., and Tang, J. (2013). Tetranuclear [MDy]2 compounds and their dinuclear [MDy] (M = Zn/Cu) building units: Their assembly, structures, and magnetic properties. Inorg. Chem. 52, 6595–6602. doi:10.1021/ic400620j
Zhang, S., -W., Duan, E., and Cheng, P. (2015b). An exceptionally stable 3D GdIII-organic framework for use as a magnetocaloric refrigerant. J. Mat. Chem. A Mat. 3, 7157–7162. doi:10.1039/c4ta06209d
Zhang, S., and Cheng, P. (2016). Coordination-cluster-based molecular magnetic refrigerants. Chem. Rec. 16, 2077–2126. doi:10.1002/tcr.201600038
Zhang, S., Duan, E., Han, Z., Li, L., and Cheng, P., (2015a). Lanthanide coordination polymers with 4, 4'-azobenzoic acid: Enhanced stability and magnetocaloric effect by removing guest solvents. Inorg. Chem. 54, 6498–6503. doi:10.1021/acs.inorgchem.5b00797
Zhao, X. Q., Wang, J., Bao, D. X., Xiang, S., Liu, Y. J., and Li, Y. C. (2017). The ferromagnetic [Ln2Co6] heterometallic complexes. Dalton Trans. 46, 2196–2203. doi:10.1039/c6dt04375e
Zheng, T.-F., Tian, X.-M., Ji, J., Luo, H., Yao, S.-L., Liu, S.-J., et al. (2020). Two Gd2 cluster complexes with monocarboxylate ligands displaying significant magnetic entropy changes. J. Mol. Struct. 1200, 127094. doi:10.1016/j.molstruc.2019.127094
Zhou, M., Wu, L.-H., Wu, X.-Y., Yao, S.-L., Zheng, T.-F., Xie, X., et al. (2021). Two dinuclear GdIII clusters based on isobutyric acid and nicotinic acid with large magnetocaloric effects. J. Mol. Struct. 1227, 129689. doi:10.1016/j.molstruc.2020.129689
Keywords: magnetocaloric effect, polynuclear, lanthanide, Schiff-based ligand, magnetic entropy
Citation: Wang M, Sun C, Gao Y, Xue H, Huang L, Xie Y, Wang J, Peng Y and Tang Y (2022) Three Gd-based magnetic refrigerant materials with high magnetic entropy: From di-nuclearity to hexa-nuclearity to octa-nuclearity. Front. Chem. 10:963203. doi: 10.3389/fchem.2022.963203
Received: 07 June 2022; Accepted: 09 September 2022;
Published: 29 September 2022.
Edited by:
Wei Zeng, Northwest Normal University, ChinaReviewed by:
Shaowei Zhang, Hunan University of Science and Technology, ChinaCai-Ming Liu, Institute of Chemistry (CAS), China
Copyright © 2022 Wang, Sun, Gao, Xue, Huang, Xie, Wang, Peng and Tang. This is an open-access article distributed under the terms of the Creative Commons Attribution License (CC BY). The use, distribution or reproduction in other forums is permitted, provided the original author(s) and the copyright owner(s) are credited and that the original publication in this journal is cited, in accordance with accepted academic practice. No use, distribution or reproduction is permitted which does not comply with these terms.
*Correspondence: Jin Wang, d2FuZ2ppbjExMEBudHUuZWR1LmNu; Yuanyuan Peng, cGVuZ3l5QHN1c3RlY2guZWR1LmNu; Yanfeng Tang, dGFuZ3lmQG50dS5lZHUuY24=
†These authors have contributed equally to this work