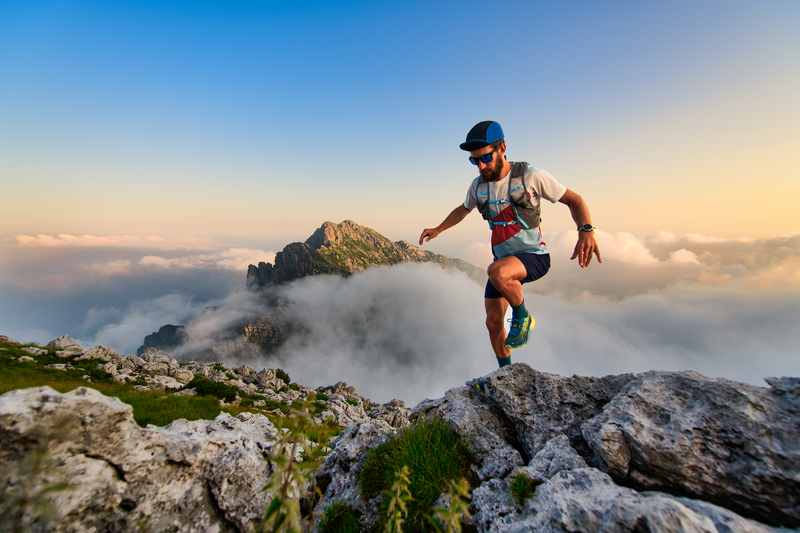
95% of researchers rate our articles as excellent or good
Learn more about the work of our research integrity team to safeguard the quality of each article we publish.
Find out more
REVIEW article
Front. Chem. , 22 August 2022
Sec. Green and Sustainable Chemistry
Volume 10 - 2022 | https://doi.org/10.3389/fchem.2022.962579
This article is part of the Research Topic Biorefinery chemicals: trend, sources and metrics View all 5 articles
Catalytic transformation of low-cost glycerol to value-added lactic acid (LA) is considered as one of the most promising technologies for the upgradation of glycerol into renewable products. Currently, research studies reveal that anaerobic transformation of glycerol to LA could also obtain green H2 with the same yield of LA. However, the combined value-added utilization of released H2 with high selectivity of LA during glycerol conversion under mild conditions still remains a grand challenge. In this perspective, for the first time, we conducted a comprehensive and critical discussion on current strategies for combined one-pot/tandem dehydrogenation of glycerol to LA with catalytic transfer hydrogenation of H2 acceptors (such as CO2) to other chemicals. The aim of this overview was to provide a general guidance on the atomic economic reaction pathway for upgrading low-cost glycerol and CO2 to LA as well as other chemicals.
Rapid consumption of fossil-based energy and materials has released major pollutants such as carbon dioxide, nitrogen oxides, and sulfur oxides, leading to significant environmental issues such as air pollution and global warming in our society. To address this challenge, renewable fuels and chemicals from catalytic conversion of biomass-derived feedstocks have gained increasing attention in the past decades. Among various renewable energies, biodiesel is considered as a good candidate for petroleum diesel due to its biodegradability, higher cetane number and engine lubricity, clean and environmental friendly nature. Biodiesel composed of mono-alkyl esters of long-chain fatty acids is derived from vegetable oils, animal fat, microalgae, and even waste cooking oils by the triglyceride-methanol or ethanolysis transesterification reaction (Abidin et al., 2012; Aboelazayem et al., 2018; Aboelazayem et al., 2019). In general, the production of biodiesel also yields a large amount of glycerol, about 10 wt% of the total biodiesel production. Rapid growth in the biodiesel industry due to its cleanness, high efficiency, and sustainability has resulted in excessive glycerol (about 4,000,000 tons per year, Figure 1), leading to a sharp drop in glycerol price (Ayoub and Abdullah, 2012; Nguyen et al., 2018). Furthermore, the current cost of biodiesel is still not competitive with diesel fuel. Therefore, economical optimization of biodiesel industry also motivated us to upgrade glycerol to valuable chemicals. In general, as a chemical building block, the glycerol can be converted to a series of value-added chemicals, such as lactic acid (LA), propanediol (PDO), ethylene glycol (EG), glyceric acid, dihydroxyacetone, glycolic acid, and tartronic acid. (Zhang et al., 2019; Meng et al., 2020; Liu et al., 2021; Sun et al., 2021; Yan et al., 2021; Zhang et al., 2021; Md Rahim et al., 2022). These products are widely used in food, medicine, organic synthesis, chemical industry, and other fields.
Lactic acid, a α-hydroxyl carboxylic acid, is considered as an important bio-based platform chemical with great application prospects (nWim Groot et al., 2010). It has been widely used in many fields, such as food, cosmetic, leather, pharmaceutical, and textile industries. It is important to highlight that LA can be applied as a monomer to synthesize biodegradable poly-(LA). Due to its biocompatibility and biodegradability, poly-(LA) is considered as a potential candidate for conventional petroleum-based polymers, such as polyethylene terephthalate, polystyrene, and polypropylene (Jamshidian et al., 2010; Djukić-Vuković et al., 2019; Luo et al., 2020). Non-degradable plastic has become a significant environmental issue on cultivated land and marine organism. To address this challenge, poly-(LA), a sustainable biodegradable polymer, has gained increasing attention in recent years (Figure 2). The annual production of poly-(LA) is estimated to be 830,000 tons in 2020 (Dreschke et al., 2015), which means a high demand for lactic acid monomers in the future. The demand for LA exceeds the supply, which drives us to increase the production efficiency of LA.
Up to date, conventional bio-fermentation of readily available sugars with microorganisms is still the major LA source, which displays advantages of utilization of renewable substrates, low processing temperature, low energy consumption, and production of optically pure D- or L-LA in the appropriate bacteria (Budhavaram and Fan, 2009; Nguyen et al., 2012; Abdel-Rahman et al., 2013; Tang et al., 2016; Djukić-Vuković et al., 2019). However, several bottlenecks limited its development to satisfy the fast-growing LA market (Figure 3) (Wang et al., 2015; Zhang et al., 2019). One limiting factor is the high cost because hydrolyzing renewable materials to remove their lignin is difficult in pretreatment processes. In addition, the difficult purification of complex fermentation productions also hampers downstream processes. Another bottleneck is very low efficiency and productivity of the fermentation method due to a long fermentation time, low concentration of substrates, and complex separation and purification. Therefore, the fermentation method may not meet the increasing market demand of LA in the future. In addition, another important method is the chemical synthesis of LA using acetaldehyde and HCN, showing a high productivity and efficiency. However, it is of less interest currently because of safety and environmental concerns (Shen et al., 2019). Hence, it is urgent to develop new technical routes for environmental friendly, cost-effective, and large-scale production of LA from abundant biomass with less energy and capital intensity.
FIGURE 3. Comparison of various LA production processes (Zhang et al., 2019).
In the past few decades, both experimental and theoretical studies have demonstrated that biomass and derived carbohydrates, including cellulose, glucose, fructose, hexose, and glycerol can be transformed into high-valued LA and other chemicals (Dusselier et al., 2013; Maki-Arvela et al., 2014; Razali and Abdullah, 2017; Lari et al., 2018; Li et al., 2018; Nda-Umar et al., 2018; Zavrazhnov et al., 2018; Kim and Moon, 2019; Li et al., 2019; Maki-Arvela et al., 2020). Among these biomass feedstocks, glycerol, as a byproduct of biodiesel production, has attracted most attention in the catalytic transformation of biomass to LA. Catalytic conversion of glycerol to LA is a promising candidate route to replace the bio-fermentation technique due to the advantages of its green nature, high efficiency, and productivity as well as cost-effectiveness, which can both upgrade the cheap glycerol and meet the growing demand for the LA market. Many reaction systems including aerobic and anaerobic have been developed in the past decades for the conversion of glycerol to LA. Both experimental and theoretical studies have confirmed that three main steps are involved in the catalytic conversion of glycerol into LA (Scheme 1), including 1) the C–H and O–H bond cleavage to glyceraldehyde or dihydroxyacetone and H2O or H2, 2) the C–O bond cleavage to pyruvaldehyde, and 3) intramolecular Cannizzaro rearrangement of pyruvaldehyde to LA (Jin et al., 2013; Zhang et al., 2019). It is generally known that the activation of C–H bond is regarded as the key reaction step in these cascade reactions (Li et al., 2019; Zhang et al., 2019). To improve the activity of C–H bond cleavage, many reaction systems, including aerobic and anaerobic, and a series of homogenous and heterogeneous catalysts have been developed in the past decades (Razali and Abdullah, 2017; Zavrazhnov et al., 2018; Li et al., 2019).
Recent reviews have detailed and summarized various catalyst types, compositions, performances, stability, and reaction parameters including the base promoter and gas atmosphere, as well as their reaction networks. For example, Razali and Abdullah (2017) provided an extensive overview on the production of LA from glycerol by elucidating the roles of metal particle sizes and distribution, base promoters, metal and support as well as reaction atmosphere. In the last year, Wang et al. summarized alkali-promoted and alkali-free catalytic systems in detail, and discussed the effect of H2 (released from dehydrogenation of glycerol) on product distribution (Li et al., 2019). However, there is lack of systematic summary on the atomic economic design of the reaction system with regard to H2 released from dehydrogenation of glycerol. To our best knowledge, anaerobic transformation of glycerol to LA could also obtain H2 with the same yield of LA at the same time, while the released hydrogen finally generated worthless H2O under O2 pressure, which is an atomic uneconomic reaction pathway. The released hydrogen in the hydrogenation reaction could participate in converting glycerol to value-added propanediol and ethylene glycol, because the metallic catalysts are active for both dehydrogenation and hydrogenation, which is not desirable due to the original intention of producing LA. Several research studies have demonstrated that adding hydrogen acceptor to the reaction system is feasible for preventing the hydrogenation reaction of intermediate such as pyruvaldehyde with in situ generated H2. Recent years have witnessed the development of combined dehydrogenation and catalytic transfer hydrogenation between glycerol and H2 acceptors. Therefore, in this review, we will focus on combined dehydrogenation of glycerol with catalytic transfer hydrogenation of H2 acceptors to value-added chemicals.
Selective oxidation of glycerol has been demonstrated to be thermodynamically more favorable for C–H bond activation under mild reaction conditions (e.g., lower operating temperature and alkali concentration), which greatly reduces energy consumption (Shen et al., 2010; Lakshmanan et al., 2013; Tao et al., 2017; Evans et al., 2020; Tao et al., 2020; Torres et al., 2021). Furthermore, low reaction temperature can also significantly inhibit C–C bond cleavage by alkalis, thus good selectivity of C3 products. Selective oxidation of glycerol to LA is generally carried out with the promotion of various noble metal and some non-noble metal catalysts (e.g., Au, Pt, Pd, and polyoxometalate ) (Shen et al., 2010; Lakshmanan et al., 2013; Xu et al., 2013; Cho et al., 2014; Purushothaman et al., 2014; Zhang et al., 2016a; Zhang et al., 2016b; Arcanjo et al., 2017; Tao et al., 2017; Zhang et al., 2017; Douthwaite et al., 2020; Evans et al., 2020; Tao et al., 2020; Torres et al., 2021; Wang et al., 2021). In the first important advances, Shen et al. (2010) reported that the bimetallic Au–Pt catalysts exhibit excellent performances with a high yield of 86% in the presence of alkali and O2 at 90°C. Much lower reaction temperature significantly limits the C–C bond cleavage, leading to favorable lower selectivity of C2 and C1 products. However, some of the glyceric acid as the main byproduct was formed due to the over-oxidation reaction. Mechanism studies reveal that oxidative dehydrogenation of glycerol to intermediates, including dihydroxyacetone and glyceraldehyde, is the key step during selective oxidation of glycerol to LA (Li et al., 2019; Zhang et al., 2019). Strong interaction and synergism effect in Au and Pt play a great role in promoting oxidative dehydrogenation of glycerol to dihydroxyacetone and glyceraldehyde. After that, the resulting intermediates undergo dehydration and subsequently benzylic acid rearrangement (some research studies proposed an internal Cannizzaro reaction) (Yin et al., 2016; Yin et al., 2017; Li et al., 2019) to LA in the presence of NaOH. As the main by-product, glyceric acid could be generated by further oxidation of glyceraldehyde over bimetallic Au–Pt catalyst under high O2 pressure. In addition, the deep-oxidation products, including tatronic acid, glycolic acid, oxalic acid, and formic acid (FA), could also be inevitably formed in the presence of metal catalysts and O2, which reduce the selectivity of LA (Wang et al., 2013; Douthwaite et al., 2020; Yan et al., 2020).
Despite fast progress in this research field, the mechanism for the formation of LA is still a subject of contention, especially the competitive pathway in dehydration of glyceraldehyde and C–C bond cleavage as well as the nature of rearrangement reaction. Recently, Evans et al. (2020) thoroughly studied the formation mechanism of LA from glycerol by conducting a series of isotopic labeling experiments with 1.3–13C glycerol using a model AuPt/TiO2 catalyst. The reaction conditions, including reaction temperature, pH, and O2 pressure, are highly influential on both the conversion rate of glycerol and product distribution (Scheme 2A). They found that catalyst, high reaction temperature, and high O2 pressure are favorable for oxidative dehydrogenation of glycerol to mixture intermediate products of dihydroxyacetone and glyceraldehyde, while pH is independent for this rate-determining step. Then, the resulting dihydroxyacetone and glyceraldehyde could undergo dehydration to 2-hydroxypropenal and isomer of pyruvaldehyde. Meanwhile, an additional competitive reaction pathway of sequential oxidation of glyceraldehyde and C–C bond cleavage occurs under O2 pressure. Notably, selectivity of LA can be significantly enhanced with the increase of the base content in the reaction system, indicating that the dehydration of glyceraldehyde to pyruvaldehyde is favored over its sequential oxidation and C–C bond cleavage reaction (Purushothaman et al., 2014). Hence, high yield of LA over glyceric acid was achieved during selective oxidation of glycerol to LA. Furthermore, isotopic labeling experiments with 1.3–13C glycerol are conducted to elucidate the formation mechanism of LA from the intermediate of pyruvaldehyde (Scheme 2B). They found that 13C signals could be detected in both the carboxylic acid and methyl groups in LA with similar quantities, suggesting that the formation of LA from pyruvaldehyde undergo a base-catalyzed 1,2-hydride shift (intramolecular Cannizzaro reaction) rather than 2,1-methide shift (benzylic acid rearrangement).
SCHEME 2. Mechanisms under alkaline conditions: (A) transformation of glycerol; (B) transformation of pyruvaldehyde.
Different from selective oxidation strategy, anaerobic transformation of glycerol to LA can avoid the over-oxidation reaction, and release H2 (in almost the same mole yield as LA) rather than a worthless H2O molecule. Thus, it could provide a higher LA yield and atomic economy, which is consistent with (Razali and Abdullah, 2017) the evolution of the modern chemical industry. It is known that H2 is an important chemical raw material, widely used in the ammonia synthesis, petrochemical, Fishcher–Tropsch process, and clean energy industry. Hence, several strategies have been developed to value-added utilization of hydrogen produced from C–H and O–H bond cleavage of glycerol (Cortright et al., 2002; Davda et al., 2005; Wen et al., 2008). Currently, various homogeneous or solid metal catalysts have been developed to catalyze glycerol dehydrogenation to LA, and release H2 at the same time (Tang et al., 2019a; Zhang et al., 2019; Ainembabazi et al., 2020; Bharath et al., 2020; Feng et al., 2020; Heltzel et al., 2020; Valekar et al., 2021; Zhang et al., 2021). For example, in alkali-catalyzed hydrothermal conversion systems, the C–H and O–H groups of glycerol can undergo a nucleophilic attack by OH− to form intermediates of glyceraldehyde or dihydroxyacetone. The intermediates subsequently undergo C–O bond cleavage and rearrangement affording lactate (Hisanori et al., 2005; Shen et al., 2009; Xu et al., 20112011). Alkali-catalyzed conversion of glycerol to LA can be carried out in several hours, addressing the low efficiency and low productivity of bio-fermentation method. A typical example, Hisanori et al. (2005) reported that hydrothermal transformation of glycerol catalyzed by NaOH showed a LA yield of 90% in 1.5 h at 300°C. However, harsh reaction conditions, such as high reaction temperature (e.g., 300°C) and high concentration of alkali (e.g., 4 mol/L), are generally needed, because the C–H bond activation is an energy-demanding process. In addition, under the harsh reaction conditions, C–C bond cleavage is favorable, leading to the formation of side products reducing selectivity of LA.
Currently, a series of homogeneous or solid metal catalysts, including Ir- (Sharninghausen et al., 2014; Lu et al., 2016; Finn et al., 2018), Pt- (Jin et al., 2013; Ftouni et al., 2015; Oberhauser et al., 2016; Tang et al., 2019b; Zhang et al., 2019), Pd- (Marques et al., 2015; Shen et al., 2019), Ru (Deng et al., 2021), Au- (Shen et al., 2017a; Palacio et al., 2019), Cu- (Roy et al., 2011; Moreira et al., 2016; Yang et al., 2016; Yin et al., 2016; Shen et al., 2017b; Li et al., 2017; Yin et al., 2017; Palacio et al., 2018a), Ni- (Qiu et al., 2018; Yin et al., 2018; Abdullah et al., 2020; Tang et al., 2020; Xiu et al., 2020), and Co-based (Palacio et al., 2018b) systems, have been developed to promote the rate-determining step under relatively mild reaction conditions (lower reaction temperature and alkali concentration). For example, our previous report (Zhang et al., 2019) indicates that Pt–Co bimetallic catalysts significantly enhance the rate of C–H and O–H bond cleavage, showing a good dehydrogenation activation for glycerol transformation at 200°C (glycerol conversion: 85%, LA selectivity: 88%). Same as aerobic transformation of glycerol, the alkalis or other solid acid/base sites exhibit a strong promotion effect for sequential dehydration and intramolecular Cannizzaro reaction. However, at such high reaction temperature, the base could catalyze retro-aldolization reaction of glyceraldehyde, leading to C–C bond cleavage, which reduces the final yield of LA. Based on the detailed studies of reaction pathways in previous works (Jin et al., 2013; Yfanti and Lemonidou, 2018), it is clear that the released hydrogen in the hydrogenation reaction forms value-added propanediol and ethylene glycol, because the metallic catalysts are active for both dehydrogenation and hydrogenation, thus showing good atomic efficiency. However, in previous works, the formation of by-products, including propanediol, ethylene glycol, and deep reduction products such as various alkanes, significantly reduces the LA selectivity, which is not desirable due to the original intention of producing LA.
To improve the LA yield, the hydrogen produced by C–H and O–H cleavage need to be consumed in time. Several research studies have demonstrated that adding hydrogen acceptor to the reaction system is feasible for preventing the hydrogenation reaction between intermediate such as pyruvaldehyde with released H2 from glycerol dehydrogenation (Figure 4) (Sharninghausen et al., 2015; Oberhauser et al., 2016; Tang et al., 2019b; Ainembabazi et al., 2020; Heltzel et al., 2020; Tang et al., 2020; Deng et al., 2021; Valekar et al., 2021). We will give a detailed overview about one-pot dehydrogenation and catalytic transfer hydrogenation between glycerol and H2 acceptors.
In the first important advances, Tang et al. (2019b) reported that adding an organic phase of cyclohexene to glycerol aqueous solution can consume the released H2 from glycerol dehydrogenation, preventing undesired hydrogenation reaction (Scheme 3A). They synthesized a series of highly dispersed Pt-based catalysts (atomically dispersed Pt species, sub-nanometer Pt clusters, and extra-fine Pt nanoparticles) supported by nanosized ZrO2 via optimization of the loading of Pt and calcination as well as reduction temperature. The high dispersed 2Pt/ZrO2-550-R250 catalysts with a narrow size distribution centered at 1.4 nm and a relatively large loading (2 wt%) of Pt nanoparticles showed an unsurpassed 95% yield of LA at 96% conversion of glycerol at 160°C in 4.5 h under 20 bar N2 pressure. This is the highest LA selectivity (∼99%) in the previous works. The novel catalytic system also leads to a selectivity of 36% in catalytic transfer hydrogenation from glycerol to cyclohexene. Apart from cyclohexene, 1-decene was also used as an H2 acceptor achieving similarly remarkable LA selectivity of 99% at glycerol conversion of 97%, while giving a significantly higher selectivity in catalytic transfer hydrogenation (92%). However, a partial deactivation of the Pt-based catalyst occurs following the aggregation of high dispersed Pt nanoparticles into larger ones (ca. 5 nm).
SCHEME 3. Catalytic reaction routes from glycerol to lactic acid with various H2 acceptors: (A) cyclohexene, (B) high pressure ethylene, and (C) acetophenone.
In order to find a significantly cheaper alternative to precious Pt-based catalysts, they investigated a series of Ni-based bimetallic catalysts for conversion of glycerol to LA, which have both good dehydrogenation and hydrogenation capacities (Tang et al., 2020). The bimetallic NiCo catalyst supported on CeO2 gave a much higher catalytic activity than the monometallic Ni/CeO2 or Co/CeO2 catalysts, during the conversion of glycerol to LA with concomitant transfer hydrogenation of various H2 acceptors (including cyclohexene, 1-decene, levulinic acid, nitrobenzene, and benzene). Combining characterization and reaction data proved that the Ni species are major active sites, but the incorporation of Co could promote dispersion and stability of Ni species on CeO2, thus leading to a remarkable LA yield of 93% at glycerol conversion of 97% at 160°C and 6.5 h under 20 bar N2 pressure. Furthermore, compared with other cheap metal catalysts, the bimetallic NiCo/CeO2 catalyst showed a remarkable catalytic performance in dehydrogenation of glycerol to LA under relatively milder reaction conditions. In addition, the recycle study revealed that the NiCo/CeO2 catalyst showed a good reusability, no loss of the original activity after three runs.
To enhance the formation of LA, supplying ethylene gas rather than liquid phase H2 acceptor to the one-pot dehydrogenation and catalytic transfer hydrogenation systems has also been demonstrated to be feasible during conversion of glycerol (Scheme 3B). Recently, Oberhauser et al. (2016) synthesized a series of Pt-based nanoparticle catalysts supported on Ketjenblack (CK) with a high surface area (∼1,400 m2/g), via the metal vapor synthesis method, used in conversion of glycerol to LA. The Pt@CK with small-sized Pt nanoparticles (mean size of 1.5 nm) showed a high LA selectivity of 95% at a glycerol conversion of near 100% at 140°C and 6 h under 875 psi ethylene pressure. Adding ethylene gas to the reaction system not only consumes the released H2 from glycerol dehydrogenation, preventing the undesired hydrogenation reaction, but also improves the conversion of glycerol. In the absence of ethylene, the Pt@CK catalyst showed poor catalytic performances with a low glycerol conversion (44%) and LA selectivity (64%), but high 1,2-PDO selectivity (36%) at 140°C and 3 h. However, with ethylene gas as an H2 acceptor (875 psi), the Pt@CK catalyst showed a significantly enhanced LA selectivity (95%) and 1,2-PDO was not observed at an increased glycerol conversion of 59%. Combining characterization and reaction data proved that the ethylene gas can stabilize together with high dispersed Pt nanoparticles (∼1.5 nm) through reversible metal atom coordination, inhibiting sintering of Pt nanoparticles. In addition, the recycle study revealed that the Pt@CK catalyst showed a good reusability, no loss of the original activity after three runs.
As mentioned earlier, various H2 acceptors, especially ethylene and cyclohexene, significantly enhance the formation of LA during one-pot tandem dehydrogenation and catalytic transfer hydrogenation of glycerol, which is greatly consistent with our original intention for producing LA from dehydrogenation of glycerol. However, the ethylene and cyclohexene were transformed into the cheaper alkane, which is undesirable. To obtain the more valuable hydrogenation products, several other unsaturated compounds have been selected as H2 acceptors replacing undesirable olefin (Scheme 3C). In the first important advances, Sharninghausen et al. (2015) synthesized a series of iron complexes of PNP pincer ligands for homogeneous conversion of glycerol at 140 °C and 6 h, leading to LA selectivity of 88% at glycerol conversion of 39%. Meanwhile, several studies have demonstrated that the Fe-PNP complex catalysts showed good activity for the hydrogenation of alcohols, esters, and N-heterocycles (Chakraborty et al., 2014a; Chakraborty et al., 2014b; Qu et al., 2014). Given the hydrogenation capacity of the Fe-PNP complexes for several unsaturated compounds, they studied combined dehydrogenation and catalytic transfer hydrogenation between glycerol and acetophenone. Surprisingly, the acetophenone was hydrogenated to 1-phenylethanol with a high yield of 95% at 120°C for 22 h. Notably, the hydrogenation product of 1-phenylethanol is an upgraded chemical than acetophenone, which is favorable in the economic area. However, the reaction performances of glycerol in this system are not analyzed in more detail.
One-pot dehydrogenation and catalytic transfer hydrogenation of glycerol and CO2/carbonate/bicarbonate to afford LA and formic acid (FA) is another attractive path to upgrading both low-value feedstocks, given the abundance of glycerol and CO2 as renewable materials (Kovács et al., 2006; Shen et al., 2012; Shen et al., 2014; Su et al., 2014; Wang et al., 2016; Heltzel et al., 2018). In the first important advances, Jin et al. (Shen et al., 2012; Shen et al., 2014; Wang et al., 2016) reported a non-catalyzed transfer hydrogenation of CO2/NaHCO3 with glycerol under alkaline hydrothermal conditions to co-production of LA and FA. In this process, the glycerol was used as a reducing agent and converted to LA with a high yield of about 90%, while the NaHCO3 was converted to FA with a same excellent yield such as LA at 300°C in 1.5 h. The effects of various parameters, for example, CO2, D2O solvent effect, reactor materials effect, and H2O molecule catalysis were investigated in detail to disclose the possible reaction mechanism. Based on the experimental data and theoretical analysis, they proposed a plausible reaction pathway as shown in Scheme 4. They claimed that the glycerol is first converted to hydroxyacetone via a dehydration and keto-enol tautomerization reaction. Subsequently, the resulting hydroxyacetone, H2O and CO2 could form an eight-membered cyclic transition state via two hydrogen bonds. Following, an intramolecular hydride shift occurs in the cyclic transition state to form pyruvaldehyde and FA, accompanied by the release of a water molecule. Finally, the resulting pyruvaldehyde undergoes a benzylic acid rearrangement to form the LA. In the proposed pathway, the water molecules are connected with the substrate molecules via the hydrogen bond for the formation of the eight-membered ring network, which is the key step of the reaction for co-production of LA and FA from glycerol and CO2. In their works, one-pot hydrogen transfer of glycerol to CO2 for affording LA and FA has been demonstrated to be feasible. However, it is necessary to further optimize the reaction system to avoid the harsh reaction conditions (300°C).
SCHEME 4. Proposed pathway of the hydrogen-transfer reduction of NaHCO3 with glycerol (Shen et al., 2012).
Recently, Heltzel et al. (2018) compared the
TABLE 1. Calculated free energies of reaction (
Su et al. (2014) reported solid Pd/AC (AC: activated carbon) catalyzed one-pot tandem dehydrogenation and catalytic transfer hydrogenation of glycerol and carbonate/bicarbonate to value-added carboxylic acids (Scheme 5). High yield of LA (55%) and FA (29%) were achieved in 12 h at 240°C under 400 psi N2 pressure. A general controversy about the one-pot hydrogen transfer reaction is the pathway for the formation of FA. They carried out a series of control reactions in the absence of glycerol or HCO3−. No FA was observed in reaction products, indicating that FA is formed by the hydrogenation of HCO3− instead of the degradation of glycerol. Notably, in their work, both CO32- and HCO3− were much easier to be hydrogenated than CO2 gas, which is different from electrochemical reduction of CO2. The highest FA yield reached 42%, while using CO32- as an H2 acceptor. However, only few FA (yield of 1.2%) and 22 turnovers were actually obtained in 12 h at 240°C, while directly using CO2 as an H2 acceptor. They also studied the possible hydrogen transfer routes in detail. Combined XRD and XPS analysis with experimental data, they proposed the plausible direct hydrogen transfer mechanism for the one-pot tandem dehydrogenation and catalytic transfer hydrogenation between glycerol and CO2 to LA as well as FA. The aforementioned tandem reaction would be strongly limited by the active sites of the Pd nanoparticles, because the co-adsorption of glycerol and HCO3− could be rate limiting. Their work proved that the one-pot catalytic transfer hydrogenation is feasible combined with the dehydrogenation of glycerol and hydrogenation of carbonate/bicarbonate. High yield of LA (∼85%) and FA (∼40%) as a value-added hydrogenation product was finally obtained under certain reaction conditions.
One-pot dehydrogenation and catalytic transfer hydrogenation of glycerol with H2 acceptor is a greatly complex parallel reaction, needing a good balance in dehydrogenation and hydrogenation reaction in a synchronized time. Thus, it is difficult to obtain a high yield of LA and FA at the same time (Heltzel et al., 2018). Two-pot reaction, separating dehydrogenation and hydrogenation processes, maybe a good strategy for efficient recovery of valuable hydrogen while achieving a high yield of LA. Recently, Siddiki et al. (2017) reported that the LA yield would be significantly enhanced via rapid removal of the released H2 from dehydrogenation of glycerol (Figure 5A). They compared the conversion of glycerol with O2 flow and static O2 pressure as well as N2 flow and static N2 pressure under the same reaction conditions (0.03 mol% Pt/AC for glycerol, 1.1 equiv. KOH, 160°C, 18 h). Under O2 or N2 flow conditions, the Pt/AC catalyzed reaction gave a significantly enhanced yield of LA (75% and 93%, respectively), but greatly reduced the hydrogenation yield (6% and less than 2%, respectively). However, under static O2 or N2 pressure in a closed reactor, the LA yield was only 56% and 59%, respectively, while the yield of hydrogenation products including 1,2-PDO, EG, and other alcohols reached up to 20%. These results indicate that the rapid removal of the released H2 from dehydrogenation of glycerol could obviously suppress the undesirable hydrogenation reaction for conversion of glycerol to LA. Compared with oxidation of H2 by O2, it is clear that purging the H2 by flowing N2 before it goes into the hydrogenation reaction is more effective for producing LA. Furthermore, the released H2 can be collected and used in many fields, including the ammonia synthesis, petrochemical, Fishcher–Tropsch process, and clean energy industry. Even more, we can design the two-pot catalytic transfer hydrogenation system by connecting partial dehydrogenation of glycerol and hydrodeoxygenation reaction with various biomass-derived substrates.
FIGURE 5. (A) Conversion of glycerol to LA under N2 flow. (B) Carbon cycles with biochemical as reductants via M0/MOx redox cycles (Jin et al., 2011).
In a typical case, glycerol can be converted into value-added chemicals via aqueous-phase hydrodeoxygenation (APH) reaction (Jin et al., 2019). Hence, we can design a two-pot tandem dehydrogenation and APH reaction for converting glycerol to achieve both good activity and selectivity for LA and 1,2-PDO. Nevertheless, a recycling system is still needed to separate released H2 from dehydrogenation of glycerol, which demands further consideration for industrial applications. Furthermore, APH of glycerol requires relatively high H2 pressure and temperature to increase the hydrogenation rate, leading to undesirable methanation reaction (Roy et al., 2010). From a molecular point of view, the difficult dissolution of molecular H2 would also reduce the intrinsic kinetics of hydrogenation reactions (Jin et al., 2019). Therefore, it is clear that there is a strong impetus to improve overall atomic and energy efficiency of tandem dehydrogenation and catalytic transfer hydrogenation technologies for achieving both high LA yield and valuable utilization of H2 released from dehydrogenation of glycerol.
Jin et al. (2011) (Yao et al., 2017) disclosed a strategy for achieving both dehydrogenation of glycerol to LA and reduction of CO2 to FA via a two-pot tandem redox reaction catalyzed by the transition metal (Figure 5B). A cycle can be achieved using the oxidative potential of zero-valent metals to reduce CO2 to FA in the presence of water and the reductive potential of glycerol to reduce the metal oxides to their zero-valent state. As an oxidation product, LA is produced in reduction of MOx to M0. Furthermore, the H2 for hydrogenation of CO2 is formed from water. For example, Fe metal first reacts with CO2 and H2O to release H2, following the resulting FeCO3 which undergoes hydrolysis to form Fe2O3 and another molecule of H2 (Scheme 6). Metals including Zn, Al, and Mn have been demonstrated to be feasible for producing H2 under similar mechanism, where they reported that a maximum H2 yield of 99% was achieved. The MOx could be reduced by glycerol to M0, and LA with a high selectivity of 95% was produced at the same time. The principle of the tandem redox reaction in CO2, glycerol and metal/metal oxide is schemed in Figure 5B. In their work, the dehydrogenation of glycerol to LA and the hydrogenation of CO2 to FA are connected by the redox reaction of a series of metal/metal oxide. The valuable utilization of H2 released from glycerol dehydrogenation is also achieved by a medium of metal–metal oxide pairs.
SCHEME 6. Possible mechanism for hydrogen generation with Fe0 (Jin et al., 2011).
Due to the intense interest in the reaction pathways of atomic economy during process development, experimental, and theoretical studies on combined dehydrogenation of glycerol to LA and catalytic transfer hydrogenation of H2 acceptors to chemicals are receiving increased interest. In this review, plausible reaction pathways and mechanisms for catalytic upgradation of glycerol into LA under both aerobic and anaerobic conditions, one-pot/tandem dehydrogenation and catalytic transfer hydrogenation between glycerol and H2 acceptors have been critically reviewed with the aim to provide insights into future development of the reaction pathways of atomic economy during process development in catalytic upgradation of unconventional resources to value-added fuels and chemicals. A variety of different H2 acceptors have been proposed with remarkable performance for transfer hydrogenation with released H2 from dehydrogenation of glycerol. Plausible reaction pathways and mechanisms have been well documented in the current work.
However, two challenges still need to be resolved for catalytic conversion of glycerol to LA with atomic economic reaction pathways:
1) Matching the reaction rates of H2 release and consumption during dehydrogenation of glycerol to LA and catalytic transfer hydrogenation of H2 acceptors. One-pot dehydrogenation and catalytic transfer hydrogenation of glycerol with H2 acceptor is a greatly complex parallel reaction, needing a good balance in dehydrogenation and hydrogenation reactions in a synchronized time. However, there is still demand for a dual-function catalyst with more activity of catalytic transfer hydrogenation of H2 acceptors to match the reaction rates of H2 release and consumption. It is expected that the novel catalyst can simultaneously improve the yield of LA and hydrogenation products.
2) Main stream research efforts have still been focused on enhancement of the yield of catalytic conversion of glycerol to LA, rather than the yield of hydrogenation products. So far, various H2 acceptors, especially cyclohexene, 1-decene, levulinic acid, nitrobenzene, benzene, and ethylene gas, significantly enhance the formation of LA during catalytic conversion of glycerol. However, these H2 acceptors are transformed into undesirable cheaper chemicals. Using CO2 and its derivatives as H2 acceptors is a good solution, because the hydrogenation products of these H2 acceptors are general value-added chemicals. In addition, catalytic transformation of CO2 to value-added chemicals or fuels provides the possibility for the carbon neutrality and sustainable development of human society. To improve hydrogenation activity and yield, it is necessary to understand H species generation from glycerol, transfer and hydrogenation with H2 acceptors. Moreover, the rational design of dual-functional (dehydrogenation and hydrogenation) catalysts still demands further experimental efforts in future studies.
GZ, JZ, XJ, YQ, and MZ drafted the manuscript. GZ, JZ, and XJ collected information. FS, JJ, WX, and BS provided comments and funding.
This work was supported by the Key Laboratory Project of Ministry of Science and Technology, SINOPEC (KL22679).
Authors GZ, JZ, YQ, MZ, XJ, FS, JJ, WX, and BS were employed by SINOPEC Research Institute of Safety Engineering Co., Ltd.
The remaining author declares that the research was conducted in the absence of any commercial or financial relationships.
The authors declare that this study received funding from SINOPEC Research Institute of Safety Engineering Co., Ltd. The funder had the following involvement in the study: the decision to submit it for publication.
All claims expressed in this article are solely those of the authors and do not necessarily represent those of their affiliated organizations, or those of the publisher, the editors, and the reviewers. Any product that may be evaluated in this article, or claim that may be made by its manufacturer, is not guaranteed or endorsed by the publisher.
Abdel-Rahman, M. A., Tashiro, Y., and Sonomoto, K. (2013). Recent advances in lactic acid production by microbial fermentation processes. Biotechnol. Adv. 31 (6), 877–902. doi:10.1016/j.biotechadv.2013.04.002
Abdullah, A. Z., Yaacob, M. H., and Basir, N. I. (2020). Synergy between oxides of Ni and Ca for selective catalytic lactic acid synthesis from glycerol in a single step process. J. Industrial Eng. Chem. 85, 282–288. doi:10.1016/j.jiec.2020.02.011
Abidin, S. Z., Haigh, K. F., and Saha, B. (2012). Esterification of free fatty acids in used cooking oil using ion-exchange resins as catalysts: An efficient pretreatment method for biodiesel feedstock. Ind. Eng. Chem. Res. 51 (45), 14653–14664. doi:10.1021/ie3007566
Aboelazayem, O., El-Gendy, N. S., Abdel-Rehim, A. A., Ashour, F., and Sadek, M. A. (2018). Biodiesel production from castor oil in Egypt: Process optimisation, kinetic study, diesel engine performance and exhaust emissions analysis. Energy 157, 843–852. doi:10.1016/j.energy.2018.05.202
Aboelazayem, O., Gadalla, M., and Saha, B. (2019). Derivatisation-free characterisation and supercritical conversion of free fatty acids into biodiesel from high acid value waste cooking oil. Renew. Energy 143, 77–90. doi:10.1016/j.renene.2019.04.106
Ainembabazi, D., Wang, K., Finn, M., Ridenour, J., and Voutchkova-Kostal, A. (2020). Efficient transfer hydrogenation of carbonate salts from glycerol using water-soluble iridium N-heterocyclic carbene catalysts. Green Chem. 22, 6093–6104. doi:10.1039/d0gc01958e
Arcanjo, M. R. A., Silva, I. J., Rodríguez-Castellón, E., Infantes-Molina, A., and Vieira, R. S. (2017). Conversion of glycerol into lactic acid using Pd or Pt supported on carbon as catalyst. Catal. Today 279, 317–326. doi:10.1016/j.cattod.2016.02.015
Ayoub, M., and Abdullah, A. Z. (2012). Critical review on the current scenario and significance of crude glycerol resulting from biodiesel industry towards more sustainable renewable energy industry. Renew. Sustain. Energy Rev. 16 (5), 2671–2686. doi:10.1016/j.rser.2012.01.054
Bharath, G., Rambabu, K., Hai, A., Taher, H., and Banat, F. (2020). Development of Au and 1D hydroxyapatite nanohybrids supported on 2D boron nitride sheets as highly efficient catalysts for dehydrogenating glycerol to lactic acid. ACS Sustain. Chem. Eng. 8, 7278–7289. doi:10.1021/acssuschemeng.9b06997
Budhavaram, N. K., and Fan, Z. (2009). Production of lactic acid from paper sludge using acid-tolerant, thermophilic Bacillus coagulan strains. Bioresour. Technol. 100 (23), 5966–5972. doi:10.1016/j.biortech.2009.01.080
Chakraborty, S., Brennessel, W. W., and Jones, W. D. (2014). A molecular iron catalyst for the acceptorless dehydrogenation and hydrogenation of N-heterocycles. J. Am. Chem. Soc. 136 (24), 8564–8567. doi:10.1021/ja504523b
Chakraborty, S., Lagaditis, P. O., Förster, M., Bielinski, E. A., Hazari, N., Holthausen, M. C., et al. (2014). Well-defined iron catalysts for the acceptorless reversible dehydrogenation-hydrogenation of alcohols and ketones. ACS Catal. 4 (11), 3994–4003. doi:10.1021/cs5009656
Cho, H. J., Chang, C.-C., and Fan, W. (2014). Base free, one-pot synthesis of lactic acid from glycerol using a bifunctional Pt/Sn-MFI catalyst. Green Chem. 16 (7), 3428–3433. doi:10.1039/c4gc00723a
Cortright, R., Davda, R., and Dumesic, J. A. (2002). Hydrogen from catalytic reforming of biomass-derived hydrocarbons in liquid water. Nature 418, 964–967. doi:10.1038/nature01009
Davda, R. R., Shabaker, J. W., Huber, G. W., Cortright, R. D., and Dumesic, J. A. (2005). A review of catalytic issues and process conditions for renewable hydrogen and alkanes by aqueous-phase reforming of oxygenated hydrocarbons over supported metal catalysts. Appl. Catal. B Environ. 56 (1-2), 171–186. doi:10.1016/j.apcatb.2004.04.027
Deng, L., Liu, X., Xu, J., Zhou, Z., Feng, S., Wang, Z., et al. (2021). Transfer hydrogenation of CO2 into formaldehyde from aqueous glycerol heterogeneously catalyzed by Ru bound to LDH. Chem. Commun. 57, 5167–5170. doi:10.1039/d1cc01299a
Djukić-Vuković, A., Mladenović, D., Ivanović, J., Pejin, J., and Mojović, L. (2019). Towards sustainability of lactic acid and poly-lactic acid polymers production. Renew. Sustain. Energy Rev. 108, 238–252. doi:10.1016/j.rser.2019.03.050
Douthwaite, M., Powell, N., Taylor, A., Ford, G., López, J. M., Solsona, B., et al. (2020). Glycerol selective oxidation to lactic acid over AuPt nanoparticles; enhancing reaction selectivity and understanding by support modification. ChemCatChem 12 (11), 3097–3107. doi:10.1002/cctc.202000026
Dreschke, G., Probst, M., Walter, A., Pumpel, T., Walde, J., and Insam, H. (2015). Lactic acid and methane: Improved exploitation of biowaste potential. Bioresour. Technol. 176, 47–55. doi:10.1016/j.biortech.2014.10.136
Dusselier, M., Van Wouwe, P., Dewaele, A., Makshina, E., and Sels, B. F. (2013). Lactic acid as a platform chemical in the biobased economy: The role of chemocatalysis. Energy Environ. Sci. 6 (5), 1415. doi:10.1039/c3ee00069a
Evans, C., Douthwaite, M., Carter, J., Pattisson, S., Kondrat, S., Bethell, D., et al. (2020). Enhancing the understanding of the glycerol to lactic acid reaction mechanism over AuPt-TiO2 under alkaline conditions. J. Chem. Phys. 152, 134705. doi:10.1063/1.5128595
Feng, S., Takahashi, K., Miura, H., and Shishido, T. (2020). One-pot synthesis of lactic acid from glycerol over a Pt-L-Nb2O5 catalyst under base-free conditions. Fuel Process. Technol. 197, 106202. doi:10.1016/j.fuproc.2019.106202
Finn, M., Ridenour, J. A., Heltzel, J., Cahill, C., and Voutchkova-Kostal, A. (2018). Next-generation water-soluble homogeneous catalysts for conversion of glycerol to lactic acid. Organometallics 37 (9), 1400–1409. doi:10.1021/acs.organomet.8b00081
Ftouni, J., Villandier, N., Auneau, F., Besson, M., Djakovitch, L., and Pinel, C. (2015). From glycerol to lactic acid under inert conditions in the presence of platinum-based catalysts: The influence of support. Catal. Today 257, 267–273. doi:10.1016/j.cattod.2014.09.034
Heltzel, J., Finn, M., Ainembabazi, D., Wang, K., and Voutchkova-Kostal, A. (2020). Transfer hydrogenation of carbon dioxide and bicarbonate from glycerol under aqueous conditions. Chem. Commun. 56, 6184–6187. doi:10.1039/c8cc03157f
Heltzel, J. M., Finn, M., Ainembabazi, D., Wang, K., and Voutchkova-Kostal, A. M. (2018). Transfer hydrogenation of carbon dioxide and bicarbonate from glycerol under aqueous conditions. Chem. Commun. 54 (48), 6184–6187. doi:10.1039/c8cc03157f
Hisanori, K., Jin, F., Zhou, Z., Takehiko, M., and Heiji, E. (2005). Conversion of glycerin into lactic acid by alkaline hydrothermal reaction. Chem. Lett. 34 (11), 1560–1561. doi:10.1246/cl.2005.1560
Jamshidian, M., Tehrany, E. A., Imran, M., Jacquot, M., and Desobry, S. (2010). Poly-lactic acid: Production, applications, nanocomposites, and release studies. Compr. Rev. Food Sci. Food Saf. 9 (5), 552–571. doi:10.1111/j.1541-4337.2010.00126.x
Jin, F., Gao, Y., Jin, Y., Zhang, Y., Cao, J., Wei, Z., et al. (2011). High-yield reduction of carbon dioxide into formic acid by zero-valent metal/metal oxide redox cycles. Energy Environ. Sci. 4 (3), 881. doi:10.1039/c0ee00661k
Jin, X., Roy, D., Thapa, P. S., Subramaniam, B., and Chaudhari, R. V. (2013). Atom economical aqueous-phase conversion (APC) of biopolyols to lactic acid, glycols, and linear alcohols using supported metal catalysts. ACS Sustain. Chem. Eng. 1 (11), 1453–1462. doi:10.1021/sc400189d
Jin, X., Yin, B., Xia, Q., Fang, T., Shen, J., Kuang, L., et al. (2019). Catalytic transfer hydrogenation of biomass-derived substrates to value-added chemicals on dual-function catalysts: Opportunities and challenges. ChemSusChem 12 (1), 71–92. doi:10.1002/cssc.201801620
Kim, Y. C., and Moon, D. J. (2019). Sustainable process for the synthesis of value-added products using glycerol as a useful raw material. Catal. Surv. Asia 23 (1), 10–22. doi:10.1007/s10563-018-09263-z
Kovács, G., Schubert, G., Joó, F., and Pápai, I. (2006). Theoretical investigation of catalytic HCO3- hydrogenation in aqueous solutions. Catal. Today 115 (1-4), 53–60. doi:10.1016/j.cattod.2006.02.018
Lakshmanan, P., Upare, P. P., Le, N.-T., Hwang, Y. K., Hwang, D. W., Lee, U. H., et al. (2013). Facile synthesis of CeO2-supported gold nanoparticle catalysts for selective oxidation of glycerol into lactic acid. Appl. Catal. A General 468, 260–268. doi:10.1016/j.apcata.2013.08.048
Lari, G. M., Pastore, G., Haus, M., Ding, Y., Papadokonstantakis, S., Mondelli, C., et al. (2018). Environmental and economical perspectives of a glycerol biorefinery. Energy Environ. Sci. 11 (5), 1012–1029. doi:10.1039/c7ee03116e
Li, K.-T., Li, J.-Y., and Li, H.-H. (2017). Conversion of glycerol to lactic acid over Cu-Zn-Al and Cu-Cr catalysts in alkaline solution. J. Taiwan Inst. Chem. Eng. 79, 74–79. doi:10.1016/j.jtice.2017.03.029
Li, S., Deng, W., Li, Y., Zhang, Q., and Wang, Y. (2019). Catalytic conversion of cellulose-based biomass and glycerol to lactic acid. J. Energy Chem. 32, 138–151. doi:10.1016/j.jechem.2018.07.012
Li, S., Deng, W., Wang, S., Wang, P., An, D., Li, Y., et al. (2018). Catalytic transformation of cellulose and its derivatives into functionalized organic acids. ChemSusChem 11 (13), 1995–2028. doi:10.1002/cssc.201800440
Liu, X., Yin, B., Zhang, W., Yu, X., Du, Y., Zhao, S., et al. (2021). Catalytic transfer hydrogenolysis of glycerol over heterogeneous catalysts: A short review on mechanistic studies. Chem. Rec. 21 (7), 1792–1810. doi:10.1002/tcr.202100037
Lu, Z., Demianets, I., Hamze, R., Terrile, N. J., and Williams, T. J. (2016). A prolific catalyst for selective conversion of neat glycerol to lactic acid. ACS Catal. 6 (3), 2014–2017. doi:10.1021/acscatal.5b02732
Luo, F., Fortenberry, A., Ren, J., and Qiang, Z. (2020). Recent progress in enhancing poly(lactic acid) stereocomplex formation for material property improvement. Front. Chem. 8, 688. doi:10.3389/fchem.2020.00688
Maki-Arvela, P., Aho, A., and Murzin, D. Y. (2020). Heterogeneous catalytic synthesis of methyl lactate and lactic acid from sugars and their derivatives. ChemSusChem 13 (18), 4833–4855. doi:10.1002/cssc.202001223
Maki-Arvela, P., Simakova, I. L., Salmi, T., and Murzin, D. Y. (2014). Production of lactic acid/lactates from biomass and their catalytic transformations to commodities. Chem. Rev. 114 (3), 1909–1971. doi:10.1021/cr400203v
Marques, F. L., Oliveira, A. C., Mendes Filho, J., Rodríguez-Castellón, E., Cavalcante, C. L., and Vieira, R. S. (2015). Synthesis of lactic acid from glycerol using a Pd/C catalyst. Fuel Process. Technol. 138, 228–235. doi:10.1016/j.fuproc.2015.05.032
Md Rahim, S. A. N., Lee, C. S., Aroua, M. K., Wan Daud, W. M. A., Abnisa, F., Cognet, P., et al. (2022). Glycerol electrocatalytic reduction using an activated carbon composite electrode: Understanding the reaction mechanisms and an optimization study. Front. Chem. 10, 845614. doi:10.3389/fchem.2022.845614
Meng, K., Zhang, G., Ding, C., Zhang, T., Yan, H., Zhang, D., et al. (2020). Recent advances on purification of lactic acid. Chem. Rec. 20 (11), 1236–1256. doi:10.1002/tcr.202000055
Moreira, A. B. F., Bruno, A. M., Souza, M. M. V. M., and Manfro, R. L. (2016). Continuous production of lactic acid from glycerol in alkaline medium using supported copper catalysts. Fuel Process. Technol. 144, 170–180. doi:10.1016/j.fuproc.2015.12.025
Nda-Umar, U., Ramli, I., Taufiq-Yap, Y., and Muhamad, E. (2018). An overview of recent research in the conversion of glycerol into biofuels, fuel additives and other bio-based chemicals. Catalysts 9 (1), 15. doi:10.3390/catal9010015
Nguyen, C. M., Kim, J. S., Hwang, H. J., Park, M. S., Choi, G. J., Choi, Y. H., et al. (2012). Production of l-lactic acid from a green microalga, Hydrodictyon reticulum, by Lactobacillus paracasei LA104 isolated from the traditional Korean food, makgeolli. Bioresour. Technol. 110, 552–559. doi:10.1016/j.biortech.2012.01.079
Nguyen, H. T. D., Tran, Y. B. N., Nguyen, H. N., Nguyen, T. C., Gandara, F., and Nguyen, P. T. K. (2018). A series of metal-organic frameworks for selective CO2 capture and catalytic oxidative carboxylation of olefins. Inorg. Chem. 57 (21), 13772–13782. doi:10.1021/acs.inorgchem.8b02293
nWim Groot, J. V. K., Sliekersl, Olav, and Aan Sicco De Vos, (2010). Production and purification of lactic acid and lactide. Chem. Rec.
Oberhauser, W., Evangelisti, C., Tiozzo, C., Vizza, F., and Psaro, R. (2016). Lactic acid from glycerol by ethylene-stabilized platinum-nanoparticles. ACS Catal. 6 (3), 1671–1674. doi:10.1021/acscatal.5b02914
Palacio, R., López, D., and Hernández, D. (2019). Bimetallic AuCu nanoparticles supported on CeO2 as selective catalysts for glycerol conversion to lactic acid in aqueous basic medium. J. Nanopart. Res. doi:10.1007/s11051-019-4594-2
Palacio, R., Torres, S., Lopez, D., and Hernandez, D. (2018). Selective glycerol conversion to lactic acid on Co3O4/CeO2 catalysts. Catal. Today 302, 196–202. doi:10.1016/j.cattod.2017.05.053
Palacio, R., Torres, S., Royer, S., Mamede, A. S., Lopez, D., and Hernandez, D. (2018). CuO/CeO2 catalysts for glycerol selective conversion to lactic acid. Dalton Trans. 47 (13), 4572–4582. doi:10.1039/c7dt04340f
Purushothaman, R. K. P., van Haveren, J., van Es, D. S., Melián-Cabrera, I., Meeldijk, J. D., and Heeres, H. J. (2014). An efficient one pot conversion of glycerol to lactic acid using bimetallic gold-platinum catalysts on a nanocrystalline CeO2 support. Appl. Catal. B Environ. 147, 92–100. doi:10.1016/j.apcatb.2013.07.068
Qiu, L., Yin, H., Yin, H., and Wang, A. (2018). Catalytic conversion of glycerol to lactic acid over hydroxyapatite-supported metallic Ni(0) nanoparticles. J. Nanosci. Nanotechnol. 18 (7), 4734–4745. doi:10.1166/jnn.2018.15327
Qu, S., Dai, H., Dang, Y., Song, C., Wang, Z.-X., and Guan, H. (2014). Computational mechanistic study of Fe-catalyzed hydrogenation of esters to alcohols: Improving catalysis by accelerating precatalyst activation with a lewis base. ACS Catal. 4 (12), 4377–4388. doi:10.1021/cs501089h
Razali, N., and Abdullah, A. Z. (2017). Production of lactic acid from glycerol via chemical conversion using solid catalyst: A review. Appl. Catal. A General 543, 234–246. doi:10.1016/j.apcata.2017.07.002
Roy, D., Subramaniam, B., and Chaudhari, R. V. (2010). Aqueous phase hydrogenolysis of glycerol to 1, 2-propanediol without external hydrogen addition. Catal. Today 156 (1-2), 31–37. doi:10.1016/j.cattod.2010.01.007
Roy, D., Subramaniam, B., and Chaudhari, R. V. (2011). Cu-based catalysts show low temperature activity for glycerol conversion to lactic acid. ACS Catal. 1 (5), 548–551. doi:10.1021/cs200080j
Sharninghausen, L. S., Campos, J., Manas, M. G., and Crabtree, R. H. (2014). Efficient selective and atom economic catalytic conversion of glycerol to lactic acid. Nat. Commun. 5 (1), 5084. doi:10.1038/ncomms6084
Sharninghausen, L. S., Mercado, B. Q., Crabtree, R. H., and Hazari, N. (2015). Selective conversion of glycerol to lactic acid with iron pincer precatalysts. Chem. Commun. 51 (90), 16201–16204. doi:10.1039/c5cc06857f
Shen, L., Yin, H., Yin, H., Liu, S., and Wang, A. (2017). Conversion of glycerol to lactic acid catalyzed by different-sized Cu2O nanoparticles in NaOH aqueous solution. J. Nanosci. Nanotechnol. 17 (1), 780–787. doi:10.1166/jnn.2017.12395
Shen, L., Yu, Z., Zhang, D., Yin, H., Wang, C., and Wang, A. (2019). Glycerol valorization to lactic acid catalyzed by hydroxyapatite-supported palladium particles. J. Chem. Technol. Biotechnol. 94 (1), 204–215. doi:10.1002/jctb.5765
Shen, L., Zhou, X., Wang, A., Yin, H., Yin, H., and Cui, W. (2017). Hydrothermal conversion of high-concentrated glycerol to lactic acid catalyzed by bimetallic CuAux (x = 0.01-0.04) nanoparticles and their reaction kinetics. RSC Adv. 7 (49), 30725–30739. doi:10.1039/c7ra04415a
Shen, Y., Zhang, S., Li, H., Ren, Y., and Liu, H. (2010). Efficient synthesis of lactic acid by aerobic oxidation of glycerol on Au-Pt/TiO2 catalysts. Chem. Eur. J. 16 (25), 7368–7371. doi:10.1002/chem.201000740
Shen, Z., Gu, M., Zhang, M., Sang, W., Zhou, X., Zhang, Y., et al. (2014). The mechanism for production of abiogenic formate from CO2 and lactate from glycerine: Uncatalyzed transfer hydrogenation of CO2 with glycerine under alkaline hydrothermal conditions. RSC Adv. 4 (29), 15256–15263. doi:10.1039/c4ra00777h
Shen, Z., Jin, F., Zhang, Y., Wu, B., Kishita, A., Tohji, K., et al. (2009). Effect of alkaline catalysts on hydrothermal conversion of glycerin into lactic acid. Ind. Eng. Chem. Res. 48 (19), 8920–8925. doi:10.1021/ie900937d
Shen, Z., Zhang, Y., and Jin, F. (2012). The alcohol-mediated reduction of CO2 and NaHCO3 into formate: A hydrogen transfer reduction of NaHCO3 with glycerine under alkaline hydrothermal conditions. RSC Adv. 2 (3), 797–801. doi:10.1039/c1ra00886b
Siddiki, S. M. A. H., Touchy, A. S., Kon, K., Toyao, T., and Shimizu, K. I. (2017). Oxidant‐free dehydrogenation of glycerol to lactic acid by heterogeneous platinum catalysts. ChemCatChem 9, 2816–2821. doi:10.1002/cctc.201700099
Su, J., Yang, L., Yang, X., Lu, M., Luo, B., and Lin, H. (2014). Simultaneously converting carbonate/bicarbonate and biomass to value-added carboxylic acid salts by aqueous-phase hydrogen transfer. ACS Sustain. Chem. Eng. 3 (1), 195–203. doi:10.1021/sc5007158
Sun, P., Zhang, W., Yu, X., Zhang, J., Xu, N., Zhang, Z., et al. (2021). Hydrogenolysis of glycerol to propylene glycol: Energy, tech-economic, and environmental studies. Front. Chem. 9, 778579. doi:10.3389/fchem.2021.778579
Tang, J., Wang, X., Hu, Y., Zhang, Y., and Li, Y. (2016). Lactic acid fermentation from food waste with indigenous microbiota: Effects of pH, temperature and high OLR. Waste Manag. 52, 278–285. doi:10.1016/j.wasman.2016.03.034
Tang, Z., Cao, H., Tao, Y., Heeres, H. J., and Pescarmona, P. P. (2020). Transfer hydrogenation from glycerol over a Ni-Co/CeO2 catalyst: A highly efficient and sustainable route to produce lactic acid. Appl. Catal. B Environ. 263, 118273. doi:10.1016/j.apcatb.2019.118273
Tang, Z., Huatang, P., Cao, H., Bals, S., Heeres, H., and Pescarmona, P. (2019). Pt/ZrO2 prepared by atomic trapping an efficient catalyst for the conversion of glycerol to lactic acid with concomitant transfer hydrogenation of cyclohexene. ACS Catal. 9, 9953–9963. doi:10.1021/acscatal.9b02139
Tang, Z., Liu, P., Cao, H., Bals, S., Heeres, H. J., and Pescarmona, P. P. (2019). Pt/ZrO2 prepared by atomic trapping: An efficient catalyst for the conversion of glycerol to lactic acid with concomitant transfer hydrogenation of cyclohexene. ACS Catal. 9 (11), 9953–9963. doi:10.1021/acscatal.9b02139
Tao, M., Sun, N., Li, Y., Tong, T., Wielicako, M., Wang, S., et al. (2017). Heteropolyacids embedded in a lipid bilayer covalently bonded to graphene oxide for the facile one-pot conversion of glycerol to lactic acid. J. Mat. Chem. A Mat. 5 (18), 8325–8333. doi:10.1039/c7ta01334e
Tao, M., Sun, N., Li, Y., Wang, S., and Wang, X. (2020). The fabrication of trifunctional polyoxometalate hybrids for the cascade conversion of glycerol to lactic acid. Catal. Sci. Technol. 10 (1), 207–214. doi:10.1039/c9cy01851d
Torres, S., Palacio, R., and Lopez, D. (2021). Support effect in Co3O4-based catalysts for selective partial oxidation of glycerol to lactic acid. Appl. Catal. A General 621, 118199. doi:10.1016/j.apcata.2021.118199
Valekar, A., Oh, K., Lee, S., and Hwang, Y. (2021). Simultaneous production of lactate and formate from glycerol and carbonates over supported Pt catalysts. J. Industrial Eng. Chem. 101, 66–77. doi:10.1016/j.jiec.2021.06.023
Wang, C., Zhang, X., Li, J., Qi, X., Guo, Z., Wei, H., et al. (2021). Gold nanoparticles on nanosheets derived from layered rare-earth hydroxides for catalytic glycerol-to-lactic acid conversion. ACS Appl. Mat. Interfaces 13, 522–530. doi:10.1021/acsami.0c17732
Wang, D., Villa, A., Su, D., Prati, L., and Schlögl, R. (2013). Carbon-supported gold nanocatalysts: Shape effect in the selective glycerol oxidation. ChemCatChem 5 (9), 2717–2723. doi:10.1002/cctc.201200535
Wang, Y., Tashiro, Y., and Sonomoto, K. (2015). Fermentative production of lactic acid from renewable materials: Recent achievements, prospects, and limits. J. Biosci. Bioeng. 119 (1), 10–18. doi:10.1016/j.jbiosc.2014.06.003
Wang, Y., Wang, F., Li, C., and Jin, F. (2016). Kinetics and mechanism of reduction of CO2 by glycerol under alkaline hydrothermal conditions. Int. J. Hydrogen Energy 41 (21), 9128–9134. doi:10.1016/j.ijhydene.2016.02.009
Wen, G., Xu, Y., Ma, H., Xu, Z., and Tian, Z. (2008). Production of hydrogen by aqueous-phase reforming of glycerol. Int. J. Hydrogen Energy 33 (22), 6657–6666. doi:10.1016/j.ijhydene.2008.07.072
Xiu, Z., Wang, H., Cai, C.-L., Li, C., Yan, L., Wang, C., et al. (2020). Ultra-fast glycerol conversion to lactic acid over magnetically recoverable Ni-NiOx@C catalysts. Industrial Eng. Chem. Res.
Xu, J., Zhang, H., Zhao, Y., Yu, B., Chen, S., Li, Y., et al. (2013). Selective oxidation of glycerol to lactic acid under acidic conditions using AuPd/TiO2 catalyst. Green Chem. 15 (6), 1520. doi:10.1039/c3gc40314a
Xu, Z., Zhen, W., and Jin, F. (20112011). Catalytic conversion of glycerin with NaOH under mild temperature. Int. Conf. Electr. Control Eng., 4405–4407.
Yan, H., Yao, S., Liang, W., Zhao, S., Jin, X., Feng, X., et al. (2020). Ni-Co oxide catalysts with lattice distortions for enhanced oxidation of glycerol to glyceric acid. J. Catal. 381, 248–260. doi:10.1016/j.jcat.2019.11.001
Yan, H., Yao, S., Zhao, S., Liu, M., Zhang, W., Zhou, X., et al. (2021). Insight into the basic strength-dependent catalytic performance in aqueous phase oxidation of glycerol to glyceric acid. Chem. Eng. Sci., 116191. doi:10.1016/j.ces.2020.116191
Yang, G.-Y., Ke, Y.-H., Ren, H.-F., Liu, C.-L., Yang, R.-Z., and Dong, W.-S. (2016). The conversion of glycerol to lactic acid catalyzed by ZrO2-supported CuO catalysts. Chem. Eng. J. 283, 759–767. doi:10.1016/j.cej.2015.08.027
Yao, G., Duo, J., Jin, B., Zhong, H., Lyu, L., Ma, Z., et al. (2017). Highly-efficient and autocatalytic reduction of NaHCO3 into formate by in situ hydrogen from water splitting with metal/metal oxide redox cycle. J. Energy Chem. 26 (5), 881–890. doi:10.1016/j.jechem.2017.08.011
Yfanti, V. L., and Lemonidou, A. A. (2018). Mechanistic study of liquid phase glycerol hydrodeoxygenation with in-situ generated hydrogen. J. Catal. 368, 98–111. doi:10.1016/j.jcat.2018.09.036
Yin, H., Yin, H., Wang, A., and Shen, L. (2018). Catalytic conversion of glycerol to lactic acid over graphite-supported nickel nanoparticles and reaction kinetics. J. Industrial Eng. Chem. 57, 226–235. doi:10.1016/j.jiec.2017.08.028
Yin, H., Yin, H., Wang, A., Shen, L., Liu, Y., and Zheng, Y. (2017). Catalytic conversion of glycerol to lactic acid over metallic copper nanoparticles and reaction kinetics. J. Nanosci. Nanotechnol. 17 (2), 1255–1266. doi:10.1166/jnn.2017.12573
Yin, H., Zhang, C., Yin, H., Gao, D., Shen, L., and Wang, A. (2016). Hydrothermal conversion of glycerol to lactic acid catalyzed by Cu/hydroxyapatite, Cu/MgO, and Cu/ZrO2 and reaction kinetics. Chem. Eng. J. 288, 332–343. doi:10.1016/j.cej.2015.12.010
Zavrazhnov, S. A., Esipovich, A. L., Danov, S. M., Zlobin, S. Y., and Belousov, A. S. (2018). Catalytic conversion of glycerol to lactic acid: State of the art and prospects. Kinet. Catal. 59 (4), 459–471. doi:10.1134/s0023158418040171
Zhang, C., Wang, T., and Ding, Y. (2017). Influence of Pt particle size on the activity of Pt/AC catalyst in selective oxidation of glycerol to lactic acid. Catal. Lett. 147 (5), 1197–1203. doi:10.1007/s10562-017-2016-6
Zhang, C., Wang, T., Liu, X., and Ding, Y. (2016). Cu-promoted Pt/activated carbon catalyst for glycerol oxidation to lactic acid. J. Mol. Catal. A Chem. 424, 91–97. doi:10.1016/j.molcata.2016.08.018
Zhang, C., Wang, T., Liu, X., and Ding, Y. (2016). Selective oxidation of glycerol to lactic acid over activated carbon supported Pt catalyst in alkaline solution. Chin. J. Catal. 37 (4), 502–509. doi:10.1016/s1872-2067(15)61055-5
Zhang, G., Jin, X., Guan, Y., Yin, B., Chen, X., Liu, Y., et al. (2019). Toward selective dehydrogenation of glycerol to lactic acid over bimetallic Pt-Co/CeOx catalysts. Ind. Eng. Chem. Res. 58, 14548–14558. doi:10.1021/acs.iecr.9b01918
Keywords: glycerol, lactic acid, H2 acceptor, CO2, dehydrogenation, catalytic transfer hydrogenation
Citation: Zhang G, Zhao J, Jin X, Qian Y, Zhou M, Jia X, Sun F, Jiang J, Xu W and Sun B (2022) Combined dehydrogenation of glycerol with catalytic transfer hydrogenation of H2 acceptors to chemicals: Opportunities and challenges. Front. Chem. 10:962579. doi: 10.3389/fchem.2022.962579
Received: 06 June 2022; Accepted: 13 July 2022;
Published: 22 August 2022.
Edited by:
Patrick Cognet, National Polytechnic Institute of Toulouse, FranceReviewed by:
Emmanuel Nicolas, Commissariat à l’Energie Atomique et aux Energies Alternatives (CEA), FranceCopyright © 2022 Zhang, Zhao, Jin, Qian, Zhou, Jia, Sun, Jiang, Xu and Sun. This is an open-access article distributed under the terms of the Creative Commons Attribution License (CC BY). The use, distribution or reproduction in other forums is permitted, provided the original author(s) and the copyright owner(s) are credited and that the original publication in this journal is cited, in accordance with accepted academic practice. No use, distribution or reproduction is permitted which does not comply with these terms.
*Correspondence: Guangyu Zhang, emhhbmdneS5xZGF5QHNpbm9wZWMuY29t
Disclaimer: All claims expressed in this article are solely those of the authors and do not necessarily represent those of their affiliated organizations, or those of the publisher, the editors and the reviewers. Any product that may be evaluated in this article or claim that may be made by its manufacturer is not guaranteed or endorsed by the publisher.
Research integrity at Frontiers
Learn more about the work of our research integrity team to safeguard the quality of each article we publish.