- 1College of Mechanical Engineering, Dongguan University of Technology, Dongguan, China
- 2Institute of Science and Technology Innovation, Dongguan University of Technology, Dongguan, China
- 3Research Center for Corrosion and Erosion Process Control of Equipment and Material in Marine Harsh Environment, Guangzhou Maritime University, Guangzhou, China
Pyrite (FeS2) is one of the potential candidates for advanced rechargeable Li-ion batteries (LIBs) owing to its inherent capacity (849 mAh g−1), environmental friendliness, and abundant natural resources. However, the volume expansion of FeS2 and the dissolution of polysulfide in the electrochemical reaction severely limit its application in the field of energy conversion and storage. Herein, FeS2 nanoparticles are encapsulated in S/N co-doped three-dimensional multi-channel structural carbon nanofibers (FeS2@CNFs) through the electrospinning method. As a cathode material for LIBs, FeS2@CNFs demonstrated excellent rate property and cyclic stability. The 3FeS2@CNFs (weight ratio of FeS2 is 30%) present the initial capacity of 1,336.7 mAh g−1 and the remaining 856.5 mAh g−1 at 0.02A g−1 after 100 circles. The favorable electrochemical properties have confirmed that carbon nanofibers can enhance the electroconductivity of electrodes, reduce the volume collapse of FeS2, and remit the dissolution of polysulfide during the Li+ ions insertion/de-insertion process. In addition, co-doped S/N can supply abundant active sites for electrochemical reactions, providing enough space for Li+ ion storage. The results indicate that 3FeS2@CNFs is a cathode with a developmental prospect for LIBs.
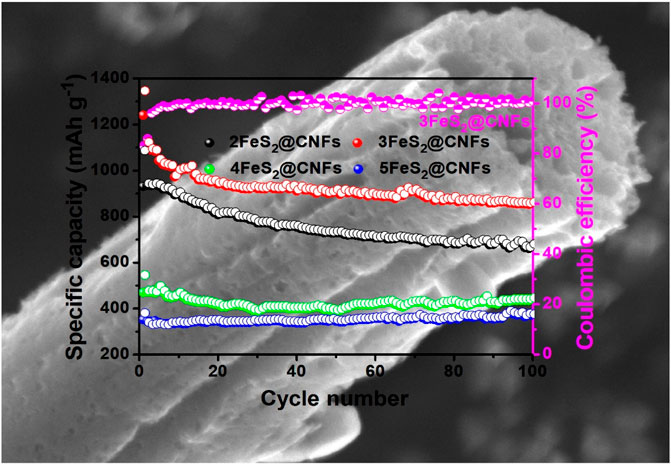
GRAPHICAL ABSTRACTGraphical Abstract Novel FeS2@CNFs nanocomposites with the multi-channel structure were successfully prepared using the electrospinning method. The three-dimensional interlinked multi-channel carbon nanofibers can facilitate the diffusion of Li+ ions and electrons. Meanwhile, the FeS2 nanoparticles are distributed on the inner wall of the carbon nanofibers, improving the phenomenon of volume expansion for FeS2 and preventing the dissolution of polysulfide during the cycling process. In addition, co-doped S/N can supply abundant active sites for electrochemical reactions, providing enough space for Li+ ion storage. The FeS2@CNFs and the preparation method have exceptional applications in the field of energy storage.
1 Introduction
As the population continues to increase, the energy demand is also growing rapidly (Zhao et al., 2015; Chi et al., 2018; Teng et al., 2019; Kesavan et al., 2020). The overexploitation of non-renewable fossil fuels has seriously polluted the environment (Zhang et al., 2019; Fang et al., 2021; Yang et al., 2021). Compared to traditional fossil fuels, electricity is a green, low-carbon, environment-friendly, and efficient energy system. To date, researchers have conducted numerous studies on electric energy storage. The common commercial electronic storage devices currently used contain nickel–cadmium batteries, lead–acid batteries, nickel–metal hydride batteries, Li-ion batteries (LIBs), fuel cells, etc. Among them, LIBs have been universally used in various fields such as manned crafts and small equipment because of their advantages of high energy density, excellent cyclic stability, and low self-discharge. Therefore, LIBs have attracted great attention. For instance, Gou et al. (2021) prepared Li3VO4/C through a facile agitation–drying method combined with subsequent calcination. The as-prepared composites were used as anode materials for LIBs and exhibited outstanding electrochemical properties. Zhong et al. (2018) synthesized a sandwich-type sulfur@Co/N-doped carbon ternary composite for Li–S batteries. The assembled Li–S batteries display excellent energy storage performance and provide the possibility of realizing industrially practical energy. Jiang et al. (2020) encapsulated NaTi2(PO4)3 nanoparticles in N/S dual-doped carbon (NTP@CNS) as the anode for LIBs via the sol–gel method followed by calcination treatment. The NTP@CNS shows excellent electrochemical property. According to daily needs and the rate of production, various types of LIBs are prepared. Recently, Li–FeS2 batteries are considered to be one of the power batteries having the most potential. However, the volume expansion of FeS2 during the intercalation and de-intercalation of Li+ ions lead to a structural collapse, reducing the cycle life of LIBs (Zhang et al., 2016; Wang et al., 2021). Meanwhile, the conversion process is accompanied by accessory substances such as lithium polysulfides (Li2Sx, 2 < x < 8). These accessory substances can make the conductivity between the anode and current collector worse. In addition, the lithium polysulfides also dissolve in the electrolyte and can gradually migrate to the cathode, leading to an increase in the impedance of the cathode (Wang et al., 2019; Li et al., 2021).
To overcome these existing issues, researchers have also attempted to nanosize FeS2. Nanocrystallization can effectively alleviate the damage caused by Li+ ions insertion/de-insertion of active materials during the charge and discharge processes, improving the cyclic stability (Lei et al., 2016). Meanwhile, the nanoscale of active substances also effectively shortens the ion transmission path, accelerates the Li+ ions diffusion rate, and improves the rate property (Polishchuk et al., 2019). Li et al. (2014) reported the synthesis of phase-pure FeS2 nanowires through thermal vulcanization of the precursor α-FeF3 3H2O nanowires. The nano-FeS2 cathode retained 350 mAh g−1 after 50 circles at 0.1°C. Liao et al. (2013) fabricated macroporous FeS2 nanotubes through a solvothermal method. The macroporous FeS2 nanotubes exhibited 925.2 mAh g−1 and retained 439 mAh g−1 at 0.2°C after 60 cycles. Nevertheless, the preparation of nanostructured single-phase FeS2 has long-term challenges due to the presence of many substoichiometric Fe–S phases and orthorhombic FeS2 (Ennaoui et al., 1993). Therefore, researchers began to attempt to hybrid nanostructured FeS2 with carbon materials. Carbon materials can not only improve the electrical conductivity and relieve the volume expansion of electrodes but also delay the damage of polysulfides during charge and discharge processes (Xu et al., 2016; He et al., 2017). For instance, Xu et al. (2016) synthesized a FeS2@HPC composite through the formation of FeS2 nanocrystals in hierarchical porous carbon. The as-fabricated FeS2@HPC presented 907 mAh g−1 and maintained 720 mAh g−1 after 100 circles at 1°C. Xu Q.-T. et al. (2018) prepared the biomass-carbon@FeS2 composites from auricularia auricula after the carbonization and sulfidation procedure. The as-synthesized composite demonstrated 850 mAh g−1 after 80 circles at 0.5°C. Wang et al. (2021) reported a raspberry-like hierarchical-structured FeS2 cathode modified by the dual-carbon framework. The as-prepared cathode delivered 566 mAh g−1 and maintained a capacity reduction rate of 0.014% for each circle at 1°C. These studies demonstrate that the development of nanocomposites combining FeS2 with carbon can improve the electrochemical properties of electrodes.
Herein, a type of FeS2@carbon nanofiber (FeS2@CNF) nanocomposites with a multi-channel structure was successfully prepared using the electrospinning method. The three-dimensional interlinked multi-channel and S/N co-doped carbon nanofibers can improve the electroconductivity of cathodes. Meanwhile, the lotus-like structure can ameliorate the phenomenon of volume expansion for FeS2 and prevent the dissolution of polysulfide during the cycling process. The effect of the FeS2 content on properties was studied through examining the performances of FeS2@CNFs nanocomposites with different contents of FeS2. The application feasibility of FeS2@CNFs as cathodes for LIBs was also explored in detail.
2 Experiment
2.1 Material Preparation
A total of 340 mg iron acetate, 400 mg polystyrene, and 500 mg polyacrylonitrile (PAN) were poured into 5 mlN, N-dimethylformamide and mixed at 65°C for 12 h. The aforementioned mixture was then electrospun with a single nozzle (21 gauge needle). The distance between the syringe and the receiver was 15 cm, the voltage was 17 kV, and the injection rate was 1 mlh−1. The as-prepared precursor film was stabilized at 200°C for 2 h and then calcined at 800°C with 5°C min−1 for 4 h in an Ar/H2 atmosphere. After reducing to 30°C, the film was sealed with sulfur powder in a quartz tube (Vproduct: Vsulfur = 1:2). Subsequently, the quartz tube was heated to 600°C and kept for 6 h. After that, the product was dissolved in CS2 to eliminate redundant sulfur. Finally, it was dried in vacuum at 100°C to obtain a lotus root–like FeS2@CNFs with many channels. The preparation process of FeS2@CNF nanocomposites based on the electrospinning approach is illustrated in Figure 1. The content of FeS2 in FeS2@CNFs nanocomposites prepared by this process was 20 wt%, which was named 2FeS2@CNFs. Samples with contents of 30, 40, and 50 wt% were also synthesized in the same way and named 2FeS2@CNFs, 3FeS2@CNFs, 4FeS2@CNFs, and 5FeS2@CNFs, respectively.
2.2 Characterization
The crystal structure information was obtained on a Rigaku diffractometer with Cu Kα radiation (λ = 1.5418) within 10–90°. Raman measurements were performed on an HR800 spectrophotometer from 400 cm−1 to 2400 cm−1. The information of the valence states was acquired using a Thermo ESCALAB 250 X-ray photoelectron spectrometer (XPS) with monochromatic Al Kα (1486.6 eV). The surface morphologies were observed using scanning and transmission electron microscopes (SEM, Ultra Plus, ZEISS and TEM, Talos F200X). The SEM was obtained at 10 kV. TEM was acquired at 200 kV accelerating voltage.
2.3 Electrochemical Measurements
The synthesized FeS2@CNFs nanocomposites were directly used as the cathodes of LIBs without any conductive agent, binder, and metal collector. The film of FeS2@CNFs was cut into a circle with a diameter of 1 cm. The mass of each cathode was about 1 mg cm−2. A total of 1 M LiPF6 in a mixture of vinyl carbonate/dimethyl carbonate (1:1 in volume) was used directly as the electrolyte. Lithium disks were used as the anode, and the Celgard 2400 microporous polypropylene membrane was employed as the separator. The aforementioned materials were assembled into CR2032 coin-type cells in an argon-filled glovebox and tested for electrochemical properties. The electrochemical properties were tested by using a CHI760E workstation and a Land CT 2001A battery testing system. The cyclic voltammetry (CV) and the galvanostatic charge and discharge (GCD) performances were determined between 1.0 and 3.0 V. Electrochemical impedance spectroscopy (EIS) was conducted at the frequency of 105–10–2 Hz.
3 Results and Discussion
The structures and phase purities of FeS2@CNFs were characterized by XRD patterns, as presented in Figure 2A. The diffraction peaks of FeS2@CNFs were consistent with the pure phase of pyrite FeS2 (JCPDS Card No. 65-3321). No diffraction peaks of the marcasite FeS2 and other impurities were observed. There is no diffraction peak of CNFs, indicating the formation of disordered layered graphite structures during the carbonization of PAN. This structure is composed of tiny crystals of layered graphite. The chemical composition of different FeS2@CNFs nanocomposites was determined using the Raman spectrum (Figure 2B). Two notable peaks at 1,352 cm−1 and 1,594 cm−1 in each spectrum match well with the D band and G band, respectively (Lu et al., 2020). The D band illustrates the defects of the carbon atom lattice, and the G band indicates the first-order scattered E2g vibration mode (Xu X. et al., 2018). The ratio (ID/IG) is higher suggesting that there are more defects on the surface of CNFs (Huang et al., 2018). The values of ID/IG for 2FeS2@CNFs, 3FeS2@CNFs, 4FeS2@CNFs, and 5FeS2@CNFs were calculated to be 1.36, 1.35, 1.33, and 1.27, respectively. As the content of FeS2 increases, the value of ID/IG gradually decreases, indicating that the FeS2@CNFs composites transform from a disordered structure to an ordered structure. The chemical composition of 3FeS2@CNFs was analyzed using the XPS spectrum (Figure 3). The survey spectrum (Figure 3A) displays four typical peaks of Fe2p, O1s, C1s, and S2p, respectively. The high-resolution spectrum of Fe2p is demonstrated in Figure 3B, the two feature peaks at 707.2 and 720.3 eV belong to Fe2p3/2 and Fe2p1/2 of pyrite FeS2, while the two peaks at 712.3 and 725.4 eV belong to slight Fe3+-S or Fe3+-O on the surface of FeS2@CNFs (Chen et al., 2019). The XPS spectra of S displayed in Figure 3C are fit into six peaks. The peaks at 163.8 and 165.1 eV match well with the S2p3/2 and S2p1/2 of FeS2, the binding energy at 164.1 and 165.3 eV are assigned to S2p3/2 and S2p1/2 of S2-, and the higher binding energy at 168.7 and 169.9 eV match well with S2p3/2 and S2p1/2 of SO42- (Zhao et al., 2017; Lin et al., 2019). In the high-resolution spectrum of C 1s (Figure 3D), C-N, C=C/C-C, and C = N peaks are displayed (Ma et al., 2018). The production of C=N and C-N bonds is due to the addition of PAN in the electrospinning process (Huang et al., 2020). The S/N co-doped FeS2@CNFs can provide abundant active sites for redox reactions, improving the electronic conductivity of FeS2@CNFs (Lu et al., 2018).
The morphology characterizations of different FeS2@CNFs were carried out by SEM and TEM. Figure 4A displays the SEM image of 2FeS2@CNFs. There are many pore channels in the CNFs (the inset of Figure 4A) and Figure 5A. As the content of FeS2 increases to 30%, there are many pore channels with different diameters inside the nanofibers parallel to the radial direction of the nanofibers. Meanwhile, many holes appear on the surface of the CNFs, as demonstrated in Figure 4B and Figure 5B. This structure can reduce diffusion resistance and facilitate the diffusion of Li+ ions. At the same time, FeS2 nanoparticles can be firmly loaded on the inner wall of the CNFs to prevent the structure from collapsing caused by volume expansion during cycling. This multi-channel structure can also effectively prevent the dissolution of intermediate products generated during electrochemical reactions (Li et al., 2015). In the SEM and TEM images of 4FeS2@CNFs (Figure 4C and Figure 5C), it can be observed that the shape of CNFs becomes irregular and the phenomenon of bending and entanglement bonding appears. Furthermore, the pores inside the nanofibers are also significantly reduced. When the FeS2 content is 50%, the shape of CNFs is more irregular and the agglomeration phenomenon is more serious. There are no obvious pores inside the CNFs (Figures 4D, 5D). In summary, as the proportion of FeS2 increases, the structure of CNFs changes. This phenomenon can be attributed to the growth and aggregation of FeS2 particles during the reaction of iron and sulfur to form FeS2, occupying the space of the pores in the nanofibers. EDS measurements of the samples were investigated, as shown in Figure 6. EDS mappings present that Fe, S, C, and N are evenly distributed on their inherent positions. The Fe element originates from the addition of iron acetate during the process of experiment. The C and N elements come from PAN. The presence of S element is due to the addition of sulfur powder.
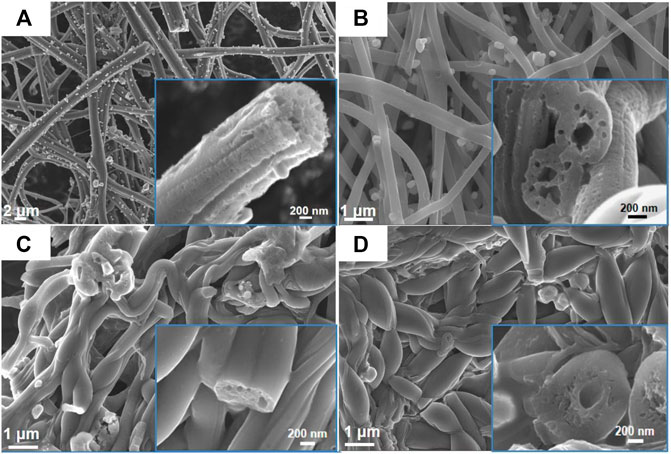
FIGURE 4. FESEM images of (A) 2FeS2@CNFs, (B) 3FeS2@CNFs, (C) 4FeS2@CNFs, and (D) 5FeS2@CNFs (The figure is a high-magnification image).
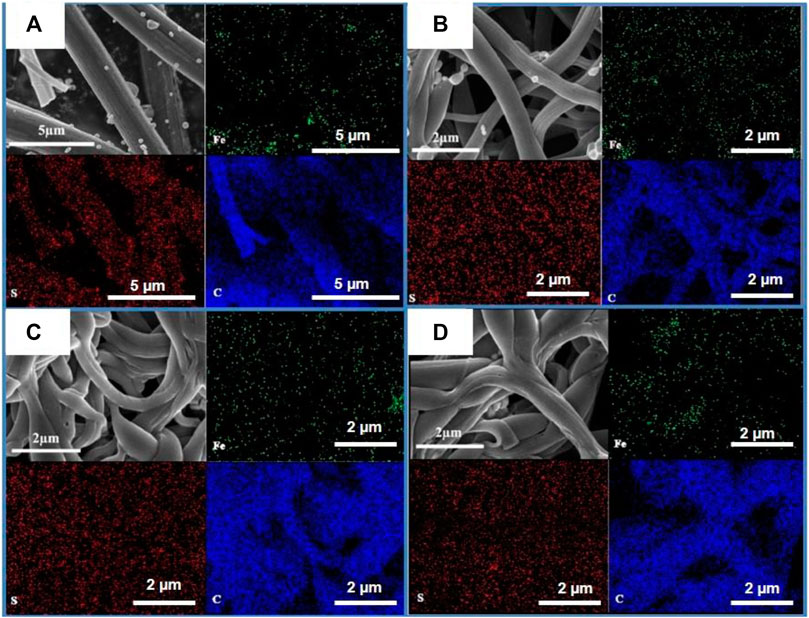
FIGURE 5. Elemental mapping and distribution of (A) 2FeS2@CNFs, (B) 3FeS2@CNFs, (C) 4FeS2@CNFs, and (D) 5FeS2@CNFs.
CV is an important method to study the lithium storage behavior of FeS2@CNF cathodes. As shown in Figures 7A–D, CV tests were carried out for different FeS2@CNF cathodes at 0.5 mV s−1 within 1–3 V. The CV curves of 2FeS2@CNFs show two oxidation peaks at 2.0 and 2.6 V, and two reduction peaks at 2.1 and 1.8 V. There are two oxidation peaks at 2.1 and 2.7 V and a reduction peak at 1.8 V in the CV curves of 3FeS2@CNFs and 4FeS2@CNFs. However, no notable redox peaks can be observed in the CV curves of 5FeS2@CNFs. Figure 7E shows the CV curves of the first cycle for different FeS2@CNFs cathodes. Taking the CV curve of the 3FeS2@CNFs cathode as an example, the electrochemical is analyzed. The reduction peak at about 1.8 V can correspond to the below formula:
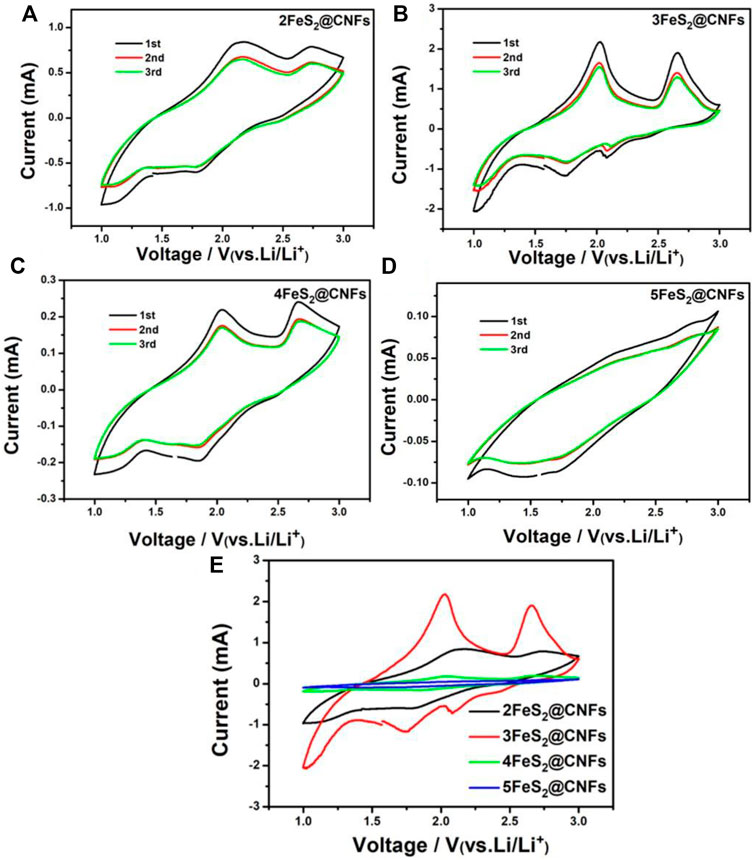
FIGURE 7. CV curves of (A) 2FeS2@CNFs, (B) 3FeS2@CNFs, (C) 4FeS2@CNFs, and (D) 5FeS2@CNFs in the initial 3 cycles at 0.5 mV s−1; (E) CV curves of the first cycle for different electrodes at 0.5 mV s−1.
The aforementioned reactions are conducted simultaneously with reaction (3). But reaction (3) can be attributed to the fact that Li+ ions show relatively slow diffusion in pyrite FeS2 at room temperature.
The oxidation peak at around 2.0 V is related to the generation of Li2-xFeS2 according to reactions (4) and (5).
The peak at around 2.6 V can be put down to the generation of FeSy and S according to formula (6).
Figures 8A–D are the GCD curves of the first three circles for different FeS2@CNFs cathodes at 20 mA g−1. It can be observed that the charge and discharge platforms of each cathode are matched well with the CV curves. Figure 8E shows the GCD profiles of the initial cycle for different cathodes at 0.02 A g−1. The initial discharge capacity of 2FeS2@CNFs, 3FeS2@CNFs, 4FeS2@CNFs, and 5FeS2@CNFs is 905.8, 1,336.7, 520.3, and 400.9 mAh g−1, respectively. It is obvious that 3FeS2@CNFs composites show a relatively high specific capacity. This is mainly because CNFs can not only improve the conductivity of the electrodes, but its internal pores can also facilitate the reversible embed/de-embed of Li+ ions. In addition, FeS2 nanoparticles can be uniformly distributed in the pores, increasing the contact area between the FeS2 and Li+ ions, and effectively prevent the dissolution of polysulfides generated during the discharge process (Li et al., 2020). 2FeS2@CNFs also have many pores, but the content of FeS2 is relatively low, so the specific capacity is less than that of 3FeS2@CNFs. As the content of FeS2 increases, the resistance of 4FeS2@CNFs and 5FeS2@CNFs increases, so their specific capacitances decrease.
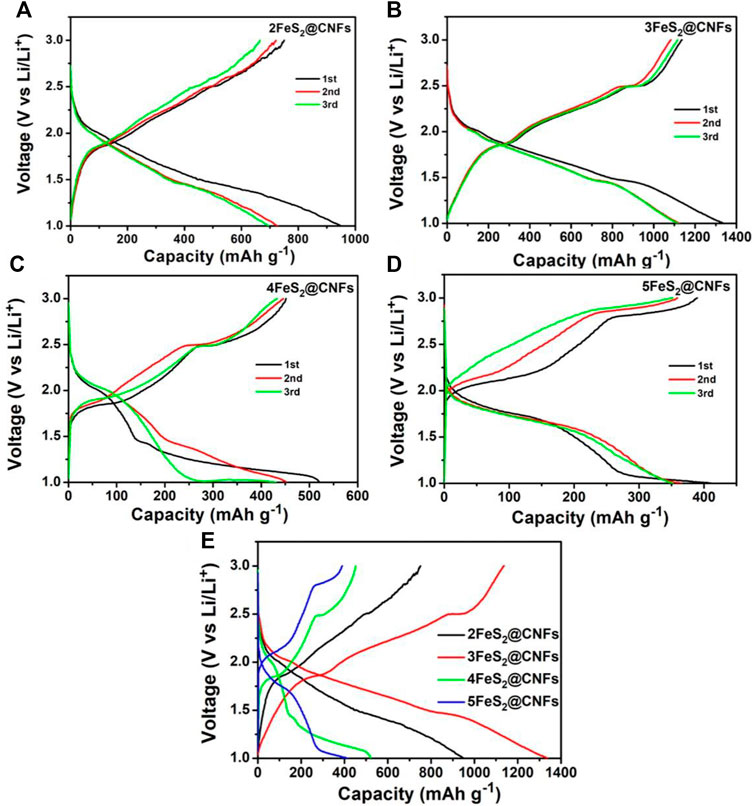
FIGURE 8. Discharge–charge profiles of (A) 2FeS2@CNFs, (B) 3FeS2@CNFs, (C) 4FeS2@CNFs, and (D) 5FeS2@CNFs 20 mA g−1 between 1 and 3 V; (E) the initial discharge-charge profiles of different electrodes at 20 mA g−1.
The cyclic performance of the samples was also determined, as presented in Figure 9A. The specific capacity of 2FeS2@CNFs, 3FeS2@CNFs, 4FeS2@CNFs, and 5FeS2@CNFs is 674.6, 856.5, 440, and 370 mAh g−1 at 20 mA g−1 after 100 cycles. The specific capacities of 2FeS2@CNFs and 3FeS2@CNFs decay during the cycling, which can be attributed to the dissolution of polysulfides during the electrochemical reaction and leading to the loss of active materials. As the proportion of FeS2 increases, the FeS2 in the pores of carbon fibers can build up and agglomerate. Therefore, the space of the pores becomes less and less, resulting in a reduction in the contact area between Li+ ions and active materials so that the phenomena of 4FeS2@CNFs and 5FeS2@CNFs are not obvious. Figure 9B shows the rate performance curves of different samples at 20, 40, 80, 100, and 200 mA g−1, respectively. It is evident that the 3FeS2@CNFs cathode exhibits the highest rate performance at various current densities among the four cathodes. The excellent electrochemical performances of the 3FeS2@CNF cathode can be attributed to the multi-channel structure of CNFs, which can supply abundant paths for ion and charge transfers. The EIS of 2FeS2@CNFs, 3FeS2@CNFs, 4FeS2@CNFs, and 5FeS2@CNFs was confirmed, as displayed in Figure 9C. The values of the equivalent series resistance and the charge transfer resistance for 3FeS2@CNFs are the smallest. The results indicate that the ratio of FeS2 and CNFs is appropriate, which allows the cathode materials possess more three-dimensional hollow channels. Therefore, numerous paths are provided to promote the transport of Li+ ions and electrons, improving the electroconductivity of the cathodes.
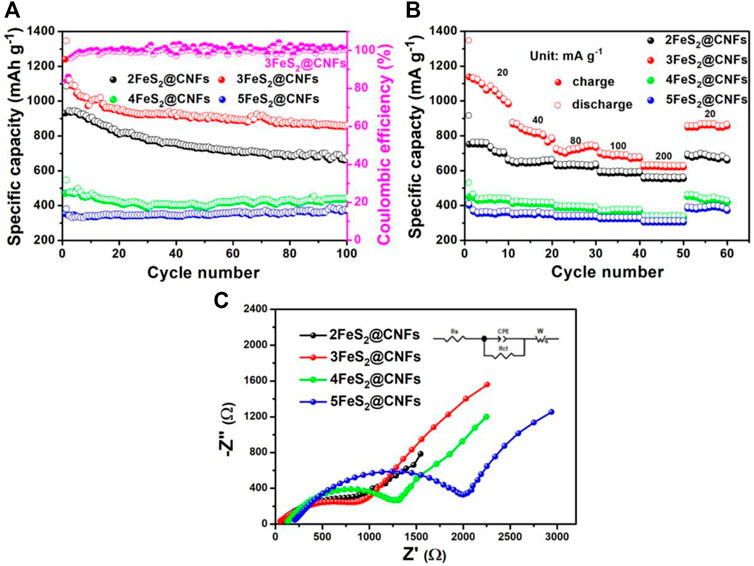
FIGURE 9. (A) Cycling performance at 20 mA g−1, (B) rate performance, and (C) EIS plots of the different electrodes.
4 Conclusion
In summary, the novel FeS2@CNFs nanocomposites with the multi-channel structure are successfully prepared by the electrospinning method. The 3FeS2@CNFs cathode exhibits an admirable capacity of 856.5 mAh g−1 at 20 mA g−1 after 100 cycles. The excellent electrochemical properties can be attributed to the right ratio of FeS2 and carbon nanofibers that can produce lots of hollow channels. The three-dimensional interlinked multi-channel carbon nanofibers can facilitate the diffusion of Li+ ions and electrons, improving the electroconductivity of cathodes. Meanwhile, the FeS2 nanoparticles are distributed on the inner wall of the carbon nanofibers, improving the phenomenon of the volume expansion for FeS2 and preventing the dissolution of polysulfides during the cycling process. In addition, S/N co-doped FeS2@CNFs can supply abundant active sites for electrochemical reactions, providing enough space for Li+ ion storage. Thus, the as-prepared 3FeS2@CNFs are a splendid cathode material for lithium-ion batteries, and it can be one of the promising candidates for next-generation secondary batteries.
Data Availability Statement
The original contributions presented in the study are included in the article/Supplementary Material; further inquiries can be directed to the corresponding author.
Author Contributions
XC: investigation and writing—original draft. YZ: conceptualization and formal analysis. CL: supervision and formal analysis. YY: supervision. YH: methodology, supervision, and writing—review and editing.
Funding
This work was supported by Program of DGUT Innovation Center of Robotics and Intelligent Equipment of China (KCYCXPT2017006), KEY Laboratory of Robotics and Intelligent Equipment of Guangdong Regular Institutions of Higher Education (2017KSYS009), and Dongguan Sci-tech Commissioner Program (20201800500252).
Conflict of Interest
The authors declare that the research was conducted in the absence of any commercial or financial relationships that could be construed as a potential conflict of interest.
Publisher’s Note
All claims expressed in this article are solely those of the authors and do not necessarily represent those of their affiliated organizations, or those of the publisher, the editors, and the reviewers. Any product that may be evaluated in this article, or claim that may be made by its manufacturer, is not guaranteed or endorsed by the publisher.
References
Chen, C., Yang, Y., Tang, X., Qiu, R., Wang, S., Cao, G., et al. (2019). Graphene-Encapsulated FeS2 in Carbon Fibers as High Reversible Anodes for Na+/K+ Batteries in a Wide Temperature Range. Small 15, 1804740. doi:10.1002/smll.201804740
Chi, J.-Q., Lin, J.-H., Qin, J.-F., Dong, B., Yan, K.-L., Liu, Z.-Z., et al. (2018). A Triple Synergistic Effect from Pitaya-like MoNix-MoCx Hybrids Encapsulated in N-Doped C Nanospheres for Efficient Hydrogen Evolution. Sustain. Energy Fuels 2, 1610–1620. doi:10.1039/C8SE00135A
Ennaoui, A., Fiechter, S., Pettenkofer, C., Alonso-Vante, N., Büker, K., Bronold, M., et al. (1993). Iron Disulfide for Solar Energy Conversion. Sol. Energy Mater. Sol. Cells 29, 289–370. doi:10.1016/0927-0248(93)90095-K
Fang, K., Ma, L., Cheng, Y.-J., Xia, S., Yang, Z., Zuo, X., et al. (2021). Si/Cu/C Nanohybrid Lithium-Ion Battery Anode with In Situ Incorporation of Nonagglomerated Super-small Copper Nanoparticles Based on Epoxy Resin. Energy fuels. 35, 6250–6264. doi:10.1021/acs.energyfuels.0c04272
Gou, W.-W., Zhou, S., Cao, X.-X., Luo, Y.-L., Kong, X.-Z., Chen, J., et al. (2021). Agitation Drying Synthesis of Porous Carbon Supported Li3VO4 as Advanced Anode Material for Lithium-Ion Batteries. Rare Mater. 40, 3466–3476. doi:10.1007/s12598-021-01712-5
He, J., Li, Q., Chen, Y., Xu, C., Zhou, K., Wang, X., et al. (2017). Self-assembled Cauliflower-like FeS2 Anchored into Graphene Foam as Free-Standing Anode for High-Performance Lithium-Ion Batteries. Carbon 114, 111–116. doi:10.1016/j.carbon.2016.12.001
Huang, K., Xing, Z., Wang, L., Wu, X., Zhao, W., Qi, X., et al. (2018). Direct Synthesis of 3D Hierarchically Porous carbon/Sn Composites via In Situ Generated NaCl Crystals as Templates for Potassium-Ion Batteries Anode. J. Mat. Chem. A 6, 434–442. doi:10.1039/C7TA08171E
Huang, Y., Bao, S., and Lu, J. (2020). Flower-like MnO2/polyaniline/hollow Mesoporous Silica as Electrode for High-Performance All-Solid-State Supercapacitors. J. Alloys Compd. 845, 156192. doi:10.1016/j.jallcom.2020.156192
Jiang, Z., Li, Y., Han, C., Huang, Z., Wu, X., He, Z., et al. (2020). Raising Lithium Storage Performances of NaTi2(PO4)3 by Nitrogen and Sulfur Dual-Doped Carbon Layer. J. Electrochem. Soc. 167, 020550. doi:10.1149/1945-7111/ab6c5c
Kesavan, T., Partheeban, T., Vivekanantha, M., Prabu, N., Kundu, M., Selvarajan, P., et al. (2020). Design of P-Doped Mesoporous Carbon Nitrides as High-Performance Anode Materials for Li-Ion Battery. ACS Appl. Mat. Interfaces 12, 24007–24018. doi:10.1021/acsami.0c05123
Li, G., Cao, S., Fu, L., Wan, S., and Liu, Q. (2021). A Two-step Hydrothermal Synthesis of TiO2/C/FeS2 Composite as High Performance Anode for Lithium Ion Batteries. Electrochimica Acta 386, 138470. doi:10.1016/j.electacta.2021.138470
Li, L., Cabán-Acevedo, M., Girard, S. N., and Jin, S. (2014). High-purity Iron Pyrite (FeS2) Nanowires as High-Capacity Nanostructured Cathodes for Lithium-Ion Batteries. Nanoscale 6, 2112–2118. doi:10.1039/C3NR05851D
Li, Z., Hu, X., Shi, Z., Lu, J., and Wang, Z. (2020). MOFs-derived Metal Oxides Inlayed in Carbon Nanofibers as Anode Materials for High-Performance Lithium-Ion Batteries. Appl. Surf. Sci. 531, 147290. doi:10.1016/j.apsusc.2020.147290
Li, Z., Zhang, J. T., Chen, Y. M., Li, J., and Lou, X. W. (2015). Pie-like Electrode Design for High-Energy Density Lithium-Sulfur Batteries. Nat. Commun. 6, 8850. doi:10.1038/ncomms9850
Liao, H. T., Wang, Y. R., Wang, J., Qian, X. F., and Cheng, S. Q. (2013). Synthesis of Macroporous FeS2 Nanotubes and Their Electrochemical Properties. Amr 774-776, 677–681. doi:10.4028/www.scientific.net/AMR.774-776.677
Lin, Z., Xiong, X., Fan, M., Xie, D., Wang, G., Yang, C., et al. (2019). Scalable Synthesis of FeS2 Nanoparticles Encapsulated into N-Doped Carbon Nanosheets as a High-Performance Sodium-Ion Battery Anode. Nanoscale 11, 3773–3779. doi:10.1039/C8NR10444A
Lu, Z., Wang, N., Zhang, Y., Xue, P., Guo, M., Tang, B., et al. (2018). Metal-Organic Framework-Derived Sea-cucumber-like FeS2@C Nanorods with Outstanding Pseudocapacitive Na-Ion Storage Properties. ACS Appl. Energy Mat. 1, 6234–6241. doi:10.1021/acsaem.8b01239
Lu, Z., Zhai, Y., Wang, N., Zhang, Y., Xue, P., Guo, M., et al. (2020). FeS2 Nanoparticles Embedded in N/S Co-doped Porous Carbon Fibers as Anode for Sodium-Ion Batteries. Chem. Eng. J. 380, 122455. doi:10.1016/j.cej.2019.122455
Ma, W., Liu, X., Lei, X., Yuan, Z., and Ding, Y. (2018). Micro/nano-structured FeS2 for High Energy Efficiency Rechargeable Li-FeS2 Battery. Chem. Eng. J. 334, 725–731. doi:10.1016/j.cej.2017.10.122
Polishchuk, Y. V., Shembel, E. M., Volfkovich, Y. M., Reisner, D., and Yu. Volfkovich, A. (2019). Synthesized Nanostructured FeS2 for Li-Batteries Application. Influence of Microstructure. Mater. Today Proc. 6, 48–55. doi:10.1016/j.matpr.2018.10.074
Teng, Y., Liu, H., Liu, D., He, H., and Chen, Y. (2019). Pitaya-like Carbon-Coated ZnS/carbon Nanospheres with Inner Three-Dimensional Nanostructure as High-Performance Anode for Lithium-Ion Battery. J. Colloid Interface Sci. 554, 220–228. doi:10.1016/j.jcis.2019.07.012
Wang, J., Fang, J., Zhao, H., Zhang, Z., and Li, Z. (2021). Raspberry-like Hierarchical Structure FeS2 Decorated by Dual-Carbon Framework as High-Performance Cathode for Rechargeable Lithium Batteries. Carbon 171, 171–178. doi:10.1016/j.carbon.2020.09.006
Wang, S., Ning, P., Huang, S., Wang, W., Fei, S., He, Q., et al. (2019). Multi-functional NiS2/FeS2/N-Doped Carbon Nanorods Derived from Metal-Organic Frameworks with Fast Reaction Kinetics for High Performance Overall Water Splitting and Lithium-Ion Batteries. J. Power Sources 436, 226857–226857.11. doi:10.1016/j.jpowsour.2019.226857
Xu, L., Hu, Y., Zhang, H., Jiang, H., and Li, C. (2016). Confined Synthesis of FeS2 Nanoparticles Encapsulated in Carbon Nanotube Hybrids for Ultrastable Lithium-Ion Batteries. ACS Sustain. Chem. Eng. 4, 4251–4255. doi:10.1021/acssuschemeng.6b00741
Xu, Q.-T., Li, J.-C., Xue, H.-G., and Guo, S.-P. (2018). Effective Combination of FeS2 Microspheres and Fe3S4 Microcubes with rGO as Anode Material for High-Capacity and Long-Cycle Lithium-Ion Batteries. J. Power Sources 396, 675–682. doi:10.1016/j.jpowsour.2018.06.088
Xu, X., Cai, T., Meng, Z., Ying, H., Xie, Y., Zhu, X., et al. (2016). FeS2 Nanocrystals Prepared in Hierarchical Porous Carbon for Lithium-Ion Battery. J. Power Sources 331, 366–372. doi:10.1016/j.jpowsour.2016.09.015
Xu, X., Meng, Z., Zhu, X., Zhang, S., and Han, W.-Q. (2018). Biomass Carbon Composited FeS 2 as Cathode Materials for High-Rate Rechargeable Lithium-Ion Battery. J. Power Sources 380, 12–17. doi:10.1016/j.jpowsour.2018.01.057
Yang, X., Chen, X., Qiu, J., Li, M., Ming, H., Zhang, S., et al. (2021). Controllable Synthesis of Silicon/carbon Hollow Microspheres Using Renewable Sources for High Energy Lithium-Ion Battery. J. Solid State Chem. 296, 121968. doi:10.1016/j.jssc.2021.121968
Zhang, F., Wang, C., Huang, G., Yin, D., and Wang, L. (2016). FeS2@C Nanowires Derived from Organic-Inorganic Hybrid Nanowires for High-Rate and Long-Life Lithium-Ion Batteries. J. Power Sources 328, 56–64. doi:10.1016/j.jpowsour.2016.07.117
Zhang, X.-Y., Guo, B.-Y., Chen, Q.-W., Dong, B., Zhang, J.-Q., Qin, J.-F., et al. (2019). Ultrafine and Highly-Dispersed Bimetal Ni2P/Co2P Encapsulated by Hollow N-Doped Carbon Nanospheres for Efficient Hydrogen Evolution. Int. J. Hydrogen Energy 44, 14908–14917. doi:10.1016/j.ijhydene.2019.04.108
Zhao, B., Wang, Q., Zhang, S., and Deng, C. (2015). Self-assembled Wafer-like Porous NaTi2(PO4)3 Decorated with Hierarchical Carbon as a High-Rate Anode for Aqueous Rechargeable Sodium Batteries. J. Mat. Chem. A 3, 12089–12096. doi:10.1039/C5TA02568K
Zhao, W., Guo, C., and Li, C. M. (2017). Lychee-like FeS2@FeSe2 Core-Shell Microspheres Anode in Sodium Ion Batteries for Large Capacity and Ultralong Cycle Life. J. Mat. Chem. A 5, 19195–19202. doi:10.1039/C7TA05931K
Keywords: FeS2, carbon nanofibers, three-dimensional multi-channel structure, cathode material, lithium-ion batteries
Citation: Cao X, Zhang Y, Luo C, Yin Y and Huang Y (2022) FeS2 Nanoparticles Encapsulated in S/N Co-Doped Carbon Nanofibers With a Three-Dimensional Multi-Channel Structure for Lithium-Ion Batteries. Front. Chem. 10:957462. doi: 10.3389/fchem.2022.957462
Received: 31 May 2022; Accepted: 15 June 2022;
Published: 15 July 2022.
Edited by:
Feng Gu, Jiangxi University of Science and Technology, ChinaCopyright © 2022 Cao, Zhang, Luo, Yin and Huang. This is an open-access article distributed under the terms of the Creative Commons Attribution License (CC BY). The use, distribution or reproduction in other forums is permitted, provided the original author(s) and the copyright owner(s) are credited and that the original publication in this journal is cited, in accordance with accepted academic practice. No use, distribution or reproduction is permitted which does not comply with these terms.
*Correspondence: Yingying Huang, WWluZ3lpbmdfSHVhbmcxOTkxQDEyNi5jb20=