- 1State Key Laboratory of Coal Conversion, Institute of Coal Chemistry, Chinese Academy of Sciences, Taiyuan, China
- 2University of the Chinese Academy of Sciences, Beijing, China
Conversion of carbon dioxide (CO2) into value-added fuels and chemicals can not only reduce the emission amount of CO2 in the atmosphere and alleviate the greenhouse effect but also realize carbon recycling. Through hydrogenation with renewable hydrogen (H2), CO2 can be transformed into various hydrocarbons and oxygenates, including methanol, ethanol, methane and light olefins, etc. Recently, metal-organic frameworks (MOFs) have attracted extensive attention in the fields of adsorption, gas separation, and catalysis due to their high surface area, abundant metal sites, and tunable metal-support interface interaction. In CO2 hydrogenation, MOFs are regarded as important supports or sacrificed precursors for the preparation of high-efficient catalysts, which can uniformly disperse metal nanoparticles (NPs) and enhance the interaction between metal and support to prevent sintering and aggregation of active metal species. This work summarizes the recent process on hydrogenation of CO2 to methanol, methane and other C2+ products over various MOFs-based catalysts, and it will provide some dues for the design of MOFs materials in energy-efficient conversion and utilization.
Introduction
Due to the rapid consumption of fossil resources, e.g., coal, petroleum, and natural gas, a large number of CO2 have been released into the atmosphere (Song, 2006). From 2006 to 2021, the global CO2 concentration in the atmosphere has been elevated from 381 to 415 ppm (NOAA, 2022). The massive emission of CO2 has brought serious environmental problems, such as global climate change and ocean acidification (Valles-Regino et al., 2015). Hence, reduction of CO2 amount and mitigation of greenhouse effect are the major challenges faced by the whole human society.
Regardless of this, CO2 is an important C1 platform molecule. Conversion of CO2 through sustainable catalytic processes into valuable chemicals and clean fuels is a promising way for CO2 utilization which could promote a circular carbon economy (Srinivas et al., 2014; Didas et al., 2015; Porosoff et al., 2016; Rafiee et al., 2018). CO2 conversion can be achieved by electro-catalysis, photo-catalysis, and thermal-catalysis processes. Electro-catalysis or photo-catalysis from clean and renewable electrical or solar energy is regarded as an important route for CO2 reduction reaction (CO2 RR). Through the rational design of high efficient catalysts, these reactions can be performed under relatively mild conditions that considerably decrease the energy consumption (Liu et al., 2012; Handoko et al., 2013; Jhong et al., 2013; Wang et al., 2015; Perathoner and Centi, 2019; Wang J.-J. et al., 2021; Zhang et al., 2021; Zhang et al., 2022a). Nevertheless, the electro-catalysis or photo-catalysis for CO2 conversion is time or geographically dependent, which, thus, decreases their economic viability. Compared to the former two manners, the thermal catalytic conversion of CO2 shows higher efficiency and it is more potential for industrial application. Since the CO2 molecule is thermodynamically stable and kinetically inert, the activation of the C=O bond in CO2 needs to overcome a high energy barrier. Renewable hydrogen (H2) generated from photolysis or electrolysis of water has high energy density and it can effectively reduce CO2. Thus, hydrogenation of CO2 into high-value hydrocarbons and oxygenates, including methane (CH4), methanol (CH3OH), ethanol (C2H5OH), and light olefins (C2=-C4=), has received increasing research interest (Sha et al., 2020).
The catalytic system for CO2 hydrogenation mainly consists of metal sites and support, including metal oxide/carbide, zeolite, graphene, and so on. Coperet and co-workers prepared zirconia-supported copper nanoparticles (NPs), which showed methanol selectivity of 75% (Larmier et al., 2017). Encapsulation of Cu NPs in Beta zeolite elevates ethanol selectivity and space-time yield (STY) to ∼ 100% and 398 mg gcat−1 h−1 (Ding et al., 2020). Highly selective conversion of CO2 into light olefins, aromatics, gasolines, and diesel was also achieved over metal oxides/zeolites bifunctional catalysts (Gao et al., 2017; Wang Y. et al., 2018; Zhou et al., 2019; Wang S. et al., 2020; Wei et al., 2021; Zhang et al., 2022b; Wang et al., 2022). In general, improvement of metal site dispersion and modulation of metal-support interaction can increase CO2 conversion and product selectivity.
In recent years, metal-organic frameworks (MOFs) have been considered important host materials in adsorption, gas separation and catalysis processes, due to their large surface area, abundant metal sites, and three-dimensional (3D) porous structure (Kumar et al., 2017; Guntern et al., 2021). MOFs are composed of metal-oxygen clusters serving as secondary building units (SBUs) that are connected by the organic ligands (Ranocchiari and Bokhoven, 2011; Chavan et al., 2012). The cages and the missing linker defects in MOFs are ideal places for confining ultra-small metal NPs, thus, inhibiting the sintering and aggregation of active sites (An et al., 2017; Zhao et al., 2018; Abdel-Mageed et al., 2019; Hu et al., 2019; Zhu et al., 2020; Yu et al., 2021). The metal-support interaction can be adjusted by controlling the pyrolysis of nodes and linkers in MOFs (Furukawa et al., 2010; Abdel-Mageed et al., 2019; Wang, 2022). This work gives a short review about the recent progress on the application of MOFs-based catalysts in CO2 hydrogenation to methanol, methane, and some C2+ products. Synthesis and structural regulation of MOFs materials have been reviewed in many other literatures (Eddaoudi et al., 2002; Cavka et al., 2008; Wang C. et al., 2018; Usman et al., 2021).
CO2 Hydrogenation to Methanol
Methanol is an important platform compound in the chemical industry, and it can be transformed into commodity olefins, aromatics, formaldehyde, and longer-chain alcohols (Liu et al., 2018; Yarulina et al., 2018). Considering the unique features role of methanol in energy conversion, a concept of “methanol economy” was proposed by Olah and co-workers (Goeppert et al., 2014). Hydrogenation of CO2 to methanol is described as the following Equation 1
Although CO2 hydrogenation to methanol is exothermic, the activation of O=C=O bond requires to overcome a high energy barrier that makes operating temperature generally as high as 240–300°C (Murthy et al., 2021). An increase in reaction temperature not only accelerates competitive reverse water-gas shift (RWGS) reaction (Equation 2) and produces more CO but also induces sintering and aggregation of active metal NPs.
One efficient method to improve the sintering resistance of active metal species is the enhancement of metal-support interface interaction through confining ultrasmall metal NPs within the pores or cages of MOFs. Yaghi and co-workers prepared the catalyst with the single Cu nanocrystal encapsulated in the cage of UiO-66 (Cu@UiO-66); Cu@UiO-66 shows 100% methanol selectivity, and 8 times higher yield than Cu/ZnO/Al2O3 in CO2 hydrogenation (Rungtaweevoranit et al., 2016). It suggests that the strong interaction between Cu nanocrystal and Zr-based SBUs of UiO-66 effectively stabilizes Cu active sites. Anchoring Cu NPs into the missing-linker defects of UiO-66 considerably enhances the interaction of metallic Cu with Zr6O8 nodes of UiO-66. It is found that the isolated Cu can only produce CO via RWGS reaction, whereas Cu NPs anchored on the Zr6O8 nodes generates larger numbers of Cu-O-Zr interface sites that show higher activity for methanol synthesis (Figure 1A) (Zhu et al., 2020). An and co-workers reported that the organic coordinating groups in MOFs play a vital role in stabilizing metal NPs (An et al., 2017). The ultra-small Cu/ZnOx NPs are in situ generated through the reduction of frameworks Cu2+ and Zn2+ ions in Zr6 clusters of UiO-bpy (2,2-bipyridine) MOF (Figure 1B). The strong interaction between Cu/ZnOx NPs and bpy moieties in MOFs prevents the phase separation of Cu/ZnOx. Thus, the prepared Cu/ZnOx@MOFs catalyst shows methanol selectivity of 100% in CO2 hydrogenation, with the STY as high as 2.59 gMeOH kgCu−1 h−1. It should be noticed that although these MOF catalysts have been widely used in CO2 hydrogenation, they usually need to be carried out at a relatively low temperature, because of their low thermal/hydrothermal stability. It is found that the organic ligands in MOF decompose easily at the high reaction temperature, causing the collapse of the pore structure and the decrease of catalytic activity.
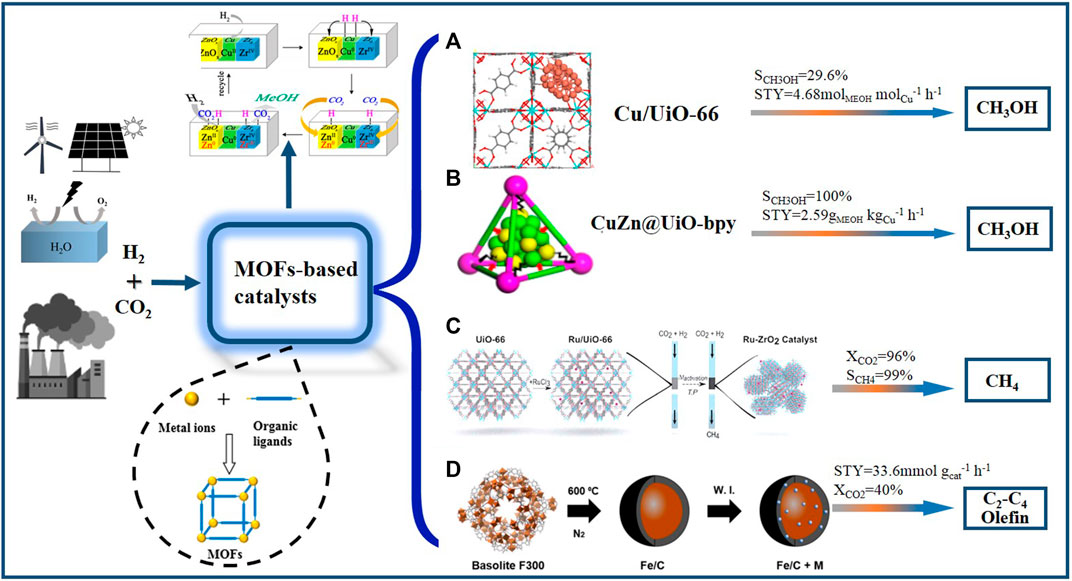
FIGURE 1. CO2 hydrogenation over various MOFs-based catalysts. (A) Interface bonding of sub-nanometer Cu clusters with Zr6O8 nodes over Cu/UiO-66 and its catalytic results in CO2 hydrogenation to methanol. Reproduced with permission from Zhu et al. (2020). Copyright 2020 Springer Nature. (B) The Cu/ZnOx NPs embedded UiO-bpy and its catalytic results in CO2 hydrogenation to methanol. Reproduced with permission from An et al. (2017). Copyright 2017 American Chemical Society. (C) Structural evolution in the decomposition process of Ru/UiO-66 and its catalytic results obtained material for CO2 hydrogenation to CH4. Reproduced with permission from Lippi et al. (2017). Copyright 2017 Royal Society of Chemistry. (D) Fe/C-K catalyst fabricated through decomposition of Basolite F300 MOF and its catalytic results obtained material for CO2 hydrogenation to CH4. Reproduced with permission from Ramirez et al. (2018). Copyright 2018 American Chemical Society.
Another way to enhance the metal-support interaction is to pyrolyze the metal-loaded MOF precursors in an inert atmosphere. Liu and co-workers fabricated a stable Cu@ZrOx catalyst via in situ treatment of Cu/UiO-66 in H2 flow at different temperatures (Liu et al., 2019). Cu@ZrOx possesses abundant Cu-ZrOx interfaces and a stable 3D ZrOx framework that leads to the formation of more Cu+ species on the surface of ZrO2. As a result, CO2 hydrogenation to methanol is significantly improved via forming more formate and methoxy intermediates. An inverse ZnO/Cu catalyst with closer proximity to ZnO-Cu interface was prepared by Hu and co-workers through directly calcining Cu@ZIF-8 (Hu et al., 2019). It is shown that the small ZnO NPs on the surface of Cu promote the formation of methanol in CO2 hydrogenation. HKUST-1 was used as the Cu source to prepare the ZrO2@HKUST-1 core-shell precursor via one-step hydrothermal method. Upon calcination and reduction, the Cu nanoclusters are highly dispersed on ZrO2, forming strong Cu-ZrO2 interface interaction. This nano Cu-ZrO2 catalyst gives a methanol space-time yield (STY) of about 5.2 times higher than that of the sample obtained by the traditional impregnation method (Yu et al., 2021).
Besides Cu-based catalysts, other metals-loaded MOFs have been developed for CO2 hydrogenation to methanol. Yin and co-workers embedded ultrasmall Pd crystals into ZIF-8 and further pyrolyzed them into PdZn alloy after calcination under airflow (Yin et al., 2018). The strong interface interaction between PdZn and ZnO not only prevents the aggregation of metal sites but also leads to the formation of more oxygen defects, thereby enhancing catalytic activity and stability of PdZn catalyst in CO2 hydrogenation to methanol. Co3O4 coated by amorphous In2O3 shell was synthesized through decomposition of In-modified ZIF-67(Co) (Pustovarenko et al., 2020). Co3O4/In2O3 core-shell catalyst exhibits a maximum methanol STY of 0.65 gMeOH gcat−1 h−1 over 100 h time on stream. Olsbye and co-workers (Gutterod et al., 2019) encapsulated Pt NPs into the octahedral cavity of UiO-67. The Pt-embedded UiO-67 produces more methanol but less methane in CO2 hydrogenation than the Pt/C, Pt/SiO2 and Pt/Al2O3 at 170°C and 1–8 bar. It is shown that the interface between Pt NPs and linker-deficient Zr6O8 nodes is the main site for methanol formation. A decrease in missing-linker defects lowered the methanol formation rate (Gutterød et al., 2020). Introduction of H2O is beneficial to increase in methanol selectivity, due to the facilitation of methanol desorption. The catalytic results of some MOFs-based catalysts in CO2 hydrogenation to methanol are summarized in Table 1.
CO2 Hydrogenation to Methane
Methane (CH4) is the main component of natural gas and it is also an important building block in the chemical industry, and can be further transformed into downstream products such as ethyne and ammonia (Bai et al., 2008; Sha et al., 2020). Hydrogenation of CO2 to CH4 provides an alternative solution to alleviated methane market shortage (Qin et al., 2017). Although CO2 methanation is a strongly exothermic reaction (Equation 3), it is always operated at high temperatures because of the kinetic limitation.
Supported ruthenium (Ru), rhodium (Rh), nickel (Ni), and cobalt (Co) catalysts are commonly used, as their high activity in CO2 hydrogenation. Strong metal-support interaction (SMSI) improves the dispersion of metal species and the stability of catalyst. Using SMSI to mediate the catalytic behavior of supported metal species is of significance in CO2 hydrogenation. MOFs are believed as promising supports or sacrificed templates, as they can promote active metal species dispersion and enhance the metal-support interaction.
Mihet and co-workers encapsulated Ni into MIL-101 (Ni@MIL-101) using the impregnation method. The high surface area (2,497 m2 g−1) and pore volume (1.75 cm3 g−1) of Ni@MIL-101 make the Ni particles highly dispersed in MIL-101 which enhances the adsorption and activation of CO2. With such a catalyst, CO2 conversion reaches 56.4%, with CH4 selectivity of 91.6% at 320°C and gas hourly space velocity (GHSV) of 4,650 ml g−1 h−1 (Mihet et al., 2019). Ni@MOF-5 shows higher Ni dispersion due to a larger surface area (2,961 m2 g−1) and thus resulting in higher CO2 conversion and CH4 selectivity of 75.1 and ∼100% respectively at 300°C, 1 atm and GHSV of 2000 ml g−1 h−1 (Zhen et al., 2015). In addition, the metal-support interaction is also enhanced by anchoring Ni on MOFs. Zhao and co-workers prepared a series of UiO-66-supported Ni-based catalysts using impregnation and reduction methods (Zhao et al., 2018). Encapsulation of ultrasmall Ni particles in UiO-66 can increase the interface interaction of Ni with UiO-66 support, which, hence, inhibits the sintering of Ni species. The prepared 20%Ni@UiO-66 exhibits CO2 conversion of 57.6% and CH4 selectivity of 100% in CO2 hydrogenation. Interestingly, no significant deactivation was observed even after a reaction of 100 h.
Lippi and co-workers investigated the structural evolution in the decomposition process of metal-loaded MOFs (Lippi et al., 2017; Lippi et al., 2021). The 3D framework of Ru-impregnated UiO-66 (Ru/UiO-66) gradually collapsed under CO2 methanation conditions to form amorphous C and Zr containing phase structure, which is then transformed into tetragonal ZrO2(t) and finally into more stable monoclinic ZrO2(m) (Figure 1C). The structure and morphology of catalyst can be precisely controlled by altering the treatment conditions during MOFs decomposition. The Ru/ZrO2(m) is a highly active and stable CO2 methanation catalyst and it gives the CO2 conversion of 96% and CH4 selectivity of 99% at 350°C and 5 bar. The catalytic results of reported MOFs-based catalysts for CO2 hydrogenation to CH4 was shown in Table 1.
CO2 Hydrogenation to C2+ Products
Conversion of CO2 to C2+ products, such as alcohol, olefins and aromatics, is highly desirable but remains a greater challenge than to C1 compounds due to the high C-C coupling barrier (Guo et al., 2018; Wei et al., 2021). Although CO2 hydrogenation to ethanol, light olefin, or aromatic is exothermic (Equations 4–6), relatively high temperature and pressure are always necessary for the activation of CO2 molecules.
Recently, some researchers suggested that direct pyrolysis of metal-loaded MOFs in an inert atmosphere can generate various metal-carbides, in which the metal NPs are closely confined in the carbon porous materials to achieve strong metal-support interaction and avoid aggregation of active sites. Tsubaki and co-workers designed the K-CuZnAl and Na-Fe@C composite catalyst. This catalyst enhances the ethanol selectivity as high as 35.0% and CO2 conversion of 39.2%, at 350°C and 5.0 MPa in CO2 hydrogenation (Wang Y. et al., 2021). The K-CuZnAl activates CO2 to form methanol and CO, and Na-Fe@C promotes the C-C coupling, with Na-Fe@C obtained from the pyrolysis of Fe-based MOFs under N2 flow. The carbon matrix effectively prevents Fe sintering and achieves the uniform dispersion of Fe active sites.
The hydrogenation of CO2 to olefins is a potential way of achieving a sustainable carbon cycle. It is generally performed via a two-step process on Fe-based catalysts: CO2 is firstly converted to CO via RWGS reaction, and then CO is hydrogenated to olefins via Fischer-Tropsch synthesis (FTS) reaction (Yang et al., 2017; De et al., 2020). Ramirez and co-workers prepared the Fe/C-K catalyst through the decomposition of Basolite F300 MOF under N2 atmosphere (Ramirez et al., 2018). It shows good catalytic performance for CO2 hydrogenation to olefins; CO2 conversion and C2=-C4= STY reach 40% and 33.6 mmol gcat−1 h−1 at 320°C and 3 MPa (Figure 1D). The uniform distribution of Fe active sites is considered effectively promote RWGS and FTS reactions.
Similarly, Li and co-workers fabricated ZnZrOx@C catalyst with three-dimensional (3D) hierarchical structure through carbonization of Zn-modified UiO-66 (Wang Y. et al., 2020). The introduction of Zn into the synthesis gel of UiO-66 induces the formation of more defects due to the substitution of Zn for Zr. Upon coupling with H-ZSM-5 zeolite, it affords the selectivity of aromatics in hydrocarbons as high as 73.1%, CH4 is decreased to 3.4%. Compared with possesses traditional ZnZrOx oxides, ZnZrOx@C catalyst formed by the carbonization of defective MOFs owns richer surface vacancies, and hence, shows higher CO/CO2 conversion due to their strong adsorption. In addition, the 3D hierarchical carbon framework structure facilitates the diffusion of products, thus, avoiding secondary reactions and elevating the proportion of benzene, toluene, and xylene (BTX) in aromatics.
Discussion
Metal-organic frameworks (MOFs) are burgeoning porous materials and they are widely used in adsorption, separation, and catalysis processes due to their unique pore structure, versatile compositions, and large surface area. The cages and the missing-linker defects in MOFs provide ideal places for encapsulating or anchoring metal nanoparticles, thereby preventing the sintering and aggregation of active sites. Nevertheless, MOFs are also important sacrificed templates for the preparation of high-efficient metal oxides or carbides. Some MOFs derived metal@C were designed, and exhibited high catalytic performance in CO2 hydrogenation to methanol, methane, and other C2+ products, due to high dispersion of active sites and strong metal-support interaction.
Despite that MOFs as supports or catalysts have received extensive attention and they show superior catalytic activity and product selectivity in CO2 hydrogenation, however, the much lower thermal and hydrothermal stability of MOFs than SiO2, Al2O3, and zeolites limits the applications in many industrial processes. This is because the organic ligands are nearly impossible to resist high temperature or their facile pyrolysis character. Nevertheless, there are potential candidates for preparing highly dispersed oxide-supported metal catalysts with strong metal-support interaction.
Author Contributions
QZ: conceptualization, writing—original draft; SW: conceptualization, funding acquisition, writing—review and editing; MD: conceptualization, funding acquisition; WF: supervision, conceptualization, funding acquisition, writing—review and editing.
Conflict of Interest
The authors declare that the research was conducted in the absence of any commercial or financial relationships that could be construed as a potential conflict of interest.
Publisher’s Note
All claims expressed in this article are solely those of the authors and do not necessarily represent those of their affiliated organizations, or those of the publisher, the editors, and the reviewers. Any product that may be evaluated in this article, or claim that may be made by its manufacturer, is not guaranteed or endorsed by the publisher.
Acknowledgments
The authors thank the financial support from the National Natural Science Foundation of China (U1910203, U1862101, 21991090, 21991092, 21802157), National Key R&D Program of China (2018YFB0604802, 2020YFA0210900); Natural Science Foundation of Shanxi Province of China (201901D211581), Youth Innovation Promotion Association CAS (2021172), Young Talent Training Program of State Key Laboratory of Coal Conversion, Institute of Coal Chemistry, CAS (2021BWZ003), and Excellent Doctoral Student Award and Subsidy program of Shanxi Province (BK2018001).
References
Abdel-Mageed, A. M., Rungtaweevoranit, B., Parlinska-Wojtan, M., Pei, X., Yaghi, O. M., and Behm, R. J. (2019). Highly Active and Stable Single-Atom Cu Catalysts Supported by a Metal-Organic Framework. J. Am. Chem. Soc. 141, 5201–5210. doi:10.1021/jacs.8b11386
An, B., Zhang, J., Cheng, K., Ji, P., Wang, C., and Lin, W. (2017). Confinement of Ultrasmall Cu/ZnOx Nanoparticles in Metal-Organic Frameworks for Selective Methanol Synthesis from Catalytic Hydrogenation of CO2. J. Am. Chem. Soc. 139, 3834–3840. doi:10.1021/jacs.7b00058
Bai, M., Zhang, Z., Bai, M., Bai, X., and Gao, H. (2008). Synthesis of Ammonia Using CH4/N2 Plasmas Based on Micro-Gap Discharge under Environmentally Friendly Condition. Plasma Chem. Plasma Process 28, 405–414. doi:10.1007/s11090-008-9132-4
Cavka, J. H., Jakobsen, S., Olsbye, U., Guillou, N., Lamberti, C., Bordiga, S., et al. (2008). A New Zirconium Inorganic Building Brick Forming Metal Organic Frameworks with Exceptional Stability. J. Am. Chem. Soc. 130, 13850–13851. doi:10.1021/ja8057953
Chavan, S., Vitillo, J. G., Gianolio, D., Zavorotynska, O., Civalleri, B., Jakobsen, S., et al. (2012). H2 Storage in Isostructural UiO-67 and UiO-66 MOFs. Phys. Chem. Chem. Phys. 14, 1614–1626. doi:10.1039/c1cp23434j
De, S., Dokania, A., Ramirez, A., and Gascon, J. (2020). Advances in the Design of Heterogeneous Catalysts and Thermocatalytic Processes for CO2 Utilization. ACS Catal. 10, 14147–14185. doi:10.1021/acscatal.0c04273
Didas, S. A., Choi, S., Chaikittisilp, W., and Jones, C. W. (2015). Amine-Oxide Hybrid Materials for CO2 Capture from Ambient Air. Acc. Chem. Res. 48, 2680–2687. doi:10.1021/acs.accounts.5b00284
Ding, L., Shi, T., Gu, J., Cui, Y., Zhang, Z., Yang, C., et al. (2020). CO2 Hydrogenation to Ethanol over Cu@Na-Beta. Chem 6, 2673–2689. doi:10.1016/j.chempr.2020.07.001
Eddaoudi, M., Kim, J., Rosi, N., Vodak, D., Wachter, J., O'Keeffe, M., et al. (2002). Systematic Design of Pore Size and Functionality in Isoreticular MOFs and Their Application in Methane Storage. Science 295, 469–472. doi:10.1126/science.1067208
Furukawa, H., Ko, N., Go, Y. B., Aratani, N., Choi, S. B., Choi, E., et al. (2010). Ultrahigh Porosity in Metal-Organic Frameworks. Science 329, 424–428. doi:10.1126/science.1192160
Gao, P., Dang, S., Li, S., Bu, X., Liu, Z., Qiu, M., et al. (2017). Direct Production of Lower Olefins from CO2 Conversion via Bifunctional Catalysis. ACS Catal. 8, 571–578. doi:10.1021/acscatal.7b02649
Goeppert, A., Czaun, M., Jones, J.-P., Surya Prakash, G. K., and Olah, G. A. (2014). Recycling of Carbon Dioxide to Methanol and Derived Products - Closing the Loop. Chem. Soc. Rev. 43, 7995–8048. doi:10.1039/c4cs00122b
Guntern, Y. T., Vávra, J., Karve, V. V., Varandili, S. B., Segura Lecina, O., Gadiyar, C., et al. (2021). Synthetic Tunability of Colloidal Covalent Organic Framework/nanocrystal Hybrids. Chem. Mat. 33, 2646–2654. doi:10.1021/acs.chemmater.1c00501
Guo, L., Sun, J., Ge, Q., and Tsubaki, N. (2018). Recent Advances in Direct Catalytic Hydrogenation of Carbon Dioxide to Valuable C2+ Hydrocarbons. J. Mat. Chem. A 6, 23244–23262. doi:10.1039/c8ta05377d
Gutterød, E. S., Pulumati, S. H., Kaur, G., Lazzarini, A., Solemsli, B. G., Gunnæs, A. E., et al. (2020). Influence of Defects and H2O on the Hydrogenation of CO2 to Methanol over Pt Nanoparticles in UiO-67 Metal-Organic Framework. J. Am. Chem. Soc. 142, 17105–17118. doi:10.1021/jacs.0c07153
Gutterod, E. S., Lazzarini, A., Fjermestad, T., Kaur, G., Manzoli, M., Bordiga, S., et al. (2019). Hydrogenation of CO2 to Methanol by Pt Nanoparticles Encapsulated in UiO-67: Deciphering the Role of the Metal–Organic Framework. J. Am. Chem. Soc. 142, 999–1009. doi:10.1021/jacs.9b10873
Handoko, A. D., Li, K., and Tang, J. (2013). Recent Progress in Artificial Photosynthesis: CO2 Photoreduction to Valuable Chemicals in a Heterogeneous System. Curr. Opin. Chem. Eng. 2, 200–206. doi:10.1016/j.coche.2012.12.003
Hu, B., Yin, Y., Zhong, Z., Wu, D., Liu, G., and Hong, X. (2019). Cu@ZIF-8 Derived Inverse ZnO/Cu Catalyst with Sub-5 Nm ZnO for Efficient CO2 Hydrogenation to Methanol. Catal. Sci. Technol. 9, 2673–2681. doi:10.1039/c8cy02546k
Jhong, H.-R. M., Ma, S., and Kenis, P. J. (2013). Electrochemical Conversion of CO2 to Useful Chemicals: Current Status, Remaining Challenges, and Future Opportunities. Curr. Opin. Chem. Eng. 2, 191–199. doi:10.1016/j.coche.2013.03.005
Kumar, P., Vellingiri, K., Kim, K.-H., Brown, R. J. C., and Manos, M. J. (2017). Modern Progress in Metal-Organic Frameworks and Their Composites for Diverse Applications. Microporous Mesoporous Mater. 253, 251–265. doi:10.1016/j.micromeso.2017.07.003
Larmier, K., Liao, W.-C., Tada, S., Lam, E., Verel, R., Bansode, A., et al. (2017). CO2-to-Methanol Hydrogenation on Zirconia-Supported Copper Nanoparticles: Reaction Intermediates and the Role of the Metal-Support Interface. Angew. Chem. Int. Ed. 56, 2318–2323. doi:10.1002/anie.201610166
Lippi, R., D’Angelo, A. M., Li, C., Howard, S. C., Madsen, I. C., Wilson, K., et al. (2021). Unveiling the Structural Transitions during Activation of a CO2 Methanation Catalyst Ru0/ZrO2 Synthesised from a MOF Precursor. Catal. Today 368, 66–77. doi:10.1016/j.cattod.2020.04.043
Lippi, R., Howard, S. C., Barron, H., Easton, C. D., Madsen, I. C., Waddington, L. J., et al. (2017). Highly Active Catalyst for CO2 Methanation Derived from a Metal Organic Framework Template. J. Mat. Chem. A 5, 12990–12997. doi:10.1039/c7ta00958e
Liu, L., Zhao, H., Andino, J. M., and Li, Y. (2012). Photocatalytic CO2 Reduction with H2O on TiO2 Nanocrystals: Comparison of Anatase, Rutile, and Brookite Polymorphs and Exploration of Surface Chemistry. ACS Catal. 2, 1817–1828. doi:10.1021/cs300273q
Liu, T., Hong, X., and Liu, G. (2019). In Situ Generation of the Cu@3D-ZrOx Framework Catalyst for Selective Methanol Synthesis from CO2/H2. ACS Catal. 10, 93–102. doi:10.1021/acscatal.9b03738
Liu, W.-C., Baek, J., and Somorjai, G. A. (2018). The Methanol Economy: Methane and Carbon Dioxide Conversion. Top. Catal. 61, 530–541. doi:10.1007/s11244-018-0907-4
Mihet, M., Grad, O., Blanita, G., Radu, T., and Lazar, M. D. (2019). Effective Encapsulation of Ni Nanoparticles in Metal-Organic Frameworks and Their Application for CO2 Methanation. Int. J. Hydrogen Energy 44, 13383–13396. doi:10.1016/j.ijhydene.2019.03.259
Murthy, P. S., Liang, W., Jiang, Y., and Huang, J. (2021). Cu-Based Nanocatalysts for CO2 Hydrogenation to Methanol. Energy fuels. 35, 8558–8584. doi:10.1021/acs.energyfuels.1c00625
NOAA (2022). Trends in Atmospheric Carbon Dioxide: Full Record. ESRL Global Monitoring Division, Global Greenhouse Gas Reference Network. Available at: https://gml.noaa.gov/ccgg/trends/global.html (Accessed Apr 15, 2022).
Perathoner, S., and Centi, G. (2019). Catalysis for Solar-Driven Chemistry: The Role of Electrocatalysis. Catal. Today 330, 157–170. doi:10.1016/j.cattod.2018.03.005
Porosoff, M. D., Yan, B., and Chen, J. G. (2016). Catalytic Reduction of CO2 by H2 for Synthesis of CO, Methanol and Hydrocarbons: Challenges and Opportunities. Energy Environ. Sci. 9, 62–73. doi:10.1039/c5ee02657a
Pustovarenko, A., Dikhtiarenko, A., Bavykina, A., Gevers, L., Ramírez, A., Russkikh, A., et al. (2020). Metal-Organic Framework-Derived Synthesis of Cobalt Indium Catalysts for the Hydrogenation of CO2 to Methanol. ACS Catal. 10, 5064–5076. doi:10.1021/acscatal.0c00449
Qin, Z., Zhou, Y., Jiang, Y., Liu, Z., and Ji, H. (2017). “Recent Advances in Heterogeneous Catalytic Hydrogenation of CO2 to Methane,” in New Advances in Hydrogenation Processes - Fundamentals and Applications (Londan: IntechOpen), 57–82.
Rafiee, A., Rajab Khalilpour, K., Milani, D., and Panahi, M. (2018). Trends in CO2 Conversion and Utilization: A Review from Process Systems Perspective. J. Environ. Chem. Eng. 6, 5771–5794. doi:10.1016/j.jece.2018.08.065
Ramirez, A., Gevers, L., Bavykina, A., Ould-Chikh, S., and Gascon, J. (2018). Metal Organic Framework-Derived Iron Catalysts for the Direct Hydrogenation of CO2 to Short Chain Olefins. ACS Catal. 8, 9174–9182. doi:10.1021/acscatal.8b02892
Ranocchiari, M., and Bokhoven, J. A. v. (2011). Catalysis by Metal–Organic Frameworks: Fundamentals and Opportunities. Phys. Chem. Chem. Phys. 13, 6388. doi:10.1039/c0cp02394a
Rungtaweevoranit, B., Baek, J., Araujo, J. R., Archanjo, B. S., Choi, K. M., Yaghi, O. M., et al. (2016). Copper Nanocrystals Encapsulated in Zr-Based Metal-Organic Frameworks for Highly Selective CO2 Hydrogenation to Methanol. Nano Lett. 16, 7645–7649. doi:10.1021/acs.nanolett.6b03637
Sha, F., Han, Z., Tang, S., Wang, J., and Li, C. (2020). Hydrogenation of Carbon Dioxide to Methanol over Non-cu-based Heterogeneous Catalysts. ChemSusChem 13, 6160–6181. doi:10.1002/cssc.202002054
Song, C. (2006). Global Challenges and Strategies for Control, Conversion and Utilization of CO2 for Sustainable Development Involving Energy, Catalysis, Adsorption and Chemical Processing. Catal. Today 115, 2–32. doi:10.1016/j.cattod.2006.02.029
Srinivas, G., Krungleviciute, V., Guo, Z.-X., and Yildirim, T. (2014). Exceptional CO2capture in a Hierarchically Porous Carbon with Simultaneous High Surface Area and Pore Volume. Energy Environ. Sci. 7, 335–342. doi:10.1039/c3ee42918k
Usman, M., Helal, A., Abdelnaby, M. M., Alloush, A. M., Zeama, M., and Yamani, Z. H. (2021). Trends and Prospects in UiO‐66 Metal‐Organic Framework for CO 2 Capture, Separation, and Conversion. Chem. Rec. 21, 1771–1791. doi:10.1002/tcr.202100030
Valles-Regino, R., Tate, R., Kelaher, B., Savins, D., Dowell, A., and Benkendorff, K. (2015). Ocean Warming and CO2-Induced Acidification Impact the Lipid Content of a Marine Predatory Gastropod. Mar. Drugs 13, 6019–6037. doi:10.3390/md13106019
Wang, C., An, B., and Lin, W. (2018). Metal-Organic Frameworks in Solid-Gas Phase Catalysis. ACS Catal. 9, 130–146. doi:10.1021/acscatal.8b04055
Wang, H. (2022). Nanostructure@metal-organic Frameworks (MOFs) for Catalytic Carbon Dioxide (CO2) Conversion in Photocatalysis, Electrocatalysis, and Thermal Catalysis. Nano Res. 15, 2834–2854. doi:10.1007/s12274-021-3984-9
Wang, J.-J., Li, X.-P., Cui, B.-F., Zhang, Z., Hu, X.-F., Ding, J., et al. (2021). A Review of Non-noble Metal-Based Electrocatalysts for CO2 Electroreduction. Rare Met. 40, 3019–3037. doi:10.1007/s12598-021-01736-x
Wang, S., Zhang, L., Wang, P., Liu, X., Chen, Y., Qin, Z., et al. (2022). Highly Effective Conversion of CO2 into Light Olefins Abundant in Ethene. Chem 8, 1376–1394. doi:10.1016/j.chempr.2022.01.004
Wang, S., Zhang, L., Zhang, W., Wang, P., Qin, Z., Yan, W., et al. (2020). Selective Conversion of CO2 into Propene and Butene. Chem 6, 3344–3363. doi:10.1016/j.chempr.2020.09.025
Wang, W.-H., Himeda, Y., Muckerman, J. T., Manbeck, G. F., and Fujita, E. (2015). CO2 Hydrogenation to Formate and Methanol as an Alternative to Photo- and Electrochemical CO2 Reduction. Chem. Rev. 115, 12936–12973. doi:10.1021/acs.chemrev.5b00197
Wang, Y., Tan, L., Tan, M., Zhang, P., Fang, Y., Yoneyama, Y., et al. (2018). Rationally Designing Bifunctional Catalysts as an Efficient Strategy to Boost CO2 Hydrogenation Producing Value-Added Aromatics. ACS Catal. 9, 895–901. doi:10.1021/acscatal.8b01344
Wang, Y., Wang, K., Zhang, B., Peng, X., Gao, X., Yang, G., et al. (2021). Direct Conversion of CO2 to Ethanol Boosted by Intimacy-Sensitive Multifunctional Catalysts. ACS Catal. 11, 11742–11753. doi:10.1021/acscatal.1c01504
Wang, Y., Zhan, W., Chen, Z., Chen, J., Li, X., and Li, Y. (2020). Advanced 3D Hollow-Out ZnZrO@C Combined with Hierarchical Zeolite for Highly Active and Selective CO Hydrogenation to Aromatics. ACS Catal. 10, 7177–7187. doi:10.1021/acscatal.0c01418
Wei, J., Yao, R., Han, Y., Ge, Q., and Sun, J. (2021). Towards the Development of the Emerging Process of CO2 Heterogenous Hydrogenation into High-Value Unsaturated Heavy Hydrocarbons. Chem. Soc. Rev. 50, 10764–10805. doi:10.1039/d1cs00260k
Yang, H., Zhang, C., Gao, P., Wang, H., Li, X., Zhong, L., et al. (2017). A Review of the Catalytic Hydrogenation of Carbon Dioxide into Value-Added Hydrocarbons. Catal. Sci. Technol. 7, 4580–4598. doi:10.1039/c7cy01403a
Yang, Y., Xu, Y., Ding, H., Yang, D., Cheng, E., Hao, Y., et al. (2021). Cu/ZnOx@UiO-66 Synthesized from a Double Solvent Method as an Efficient Catalyst for CO2 Hydrogenation to Methanol. Catal. Sci. Technol. 11, 4367–4375. doi:10.1039/d0cy02450c
Yarulina, I., Chowdhury, A. D., Meirer, F., Weckhuysen, B. M., and Gascon, J. (2018). Recent Trends and Fundamental Insights in the Methanol-To-Hydrocarbons Process. Nat. Catal. 1, 398–411. doi:10.1038/s41929-018-0078-5
Yin, Y., Hu, B., Li, X., Zhou, X., Hong, X., and Liu, G. (2018). Pd@zeolitic Imidazolate Framework-8 Derived PdZn Alloy Catalysts for Efficient Hydrogenation of CO2 to Methanol. Appl. Catal. B Environ. 234, 143–152. doi:10.1016/j.apcatb.2018.04.024
Yu, J., Liu, S., Mu, X., Yang, G., Luo, X., Lester, E., et al. (2021). Cu-ZrO2 Catalysts with Highly Dispersed Cu Nanoclusters Derived from ZrO2@ HKUST-1 Composites for the Enhanced CO2 Hydrogenation to Methanol. Chem. Eng. J. 419, 129656. doi:10.1016/j.cej.2021.129656
Zhang, W., Jin, Z., and Chen, Z. (2022a). Rational‐Designed Principles for Electrochemical and Photoelectrochemical Upgrading of CO2 to Value‐Added Chemicals. Adv. Sci. 9, 2105204. doi:10.1002/advs.202105204
Zhang, W., Ma, D., Pérez-Ramírez, J., and Chen, Z. (2021). Recent Progress in Materials Exploration for Thermocatalytic, Photocatalytic, and Integrated Photothermocatalytic CO 2 ‐to‐Fuel Conversion. Adv Energy Sustain Res 3, 2100169. doi:10.1002/aesr.202100169
Zhang, W., Wang, S., Guo, S., Qin, Z., Dong, M., Wang, J., et al. (2022b). Effective Conversion of CO2 into Light Olefins over a Bifunctional Catalyst Consisting of La-Modified ZnZrOx Oxide and Acidic Zeolite. Catal. Sci. Technol. 12, 2566–2577. doi:10.1039/d2cy00210h
Zhao, Z.-W., Zhou, X., Liu, Y.-N., Shen, C.-C., Yuan, C.-Z., Jiang, Y.-F., et al. (2018). Ultrasmall Ni Nanoparticles Embedded in Zr-Based MOFs Provide High Selectivity for CO2 Hydrogenation to Methane at Low Temperatures. Catal. Sci. Technol. 8, 3160–3165. doi:10.1039/c8cy00468d
Zhen, W., Li, B., Lu, G., and Ma, J. (2015). Enhancing Catalytic Activity and Stability for CO2 Methanation on Ni@MOF-5 via Control of Active Species Dispersion. Chem. Commun. 51, 1728–1731. doi:10.1039/c4cc08733j
Zhou, C., Shi, J., Zhou, W., Cheng, K., Zhang, Q., Kang, J., et al. (2019). Highly Active ZnO-ZrO2 Aerogels Integrated with H-ZSM-5 for Aromatics Synthesis from Carbon Dioxide. ACS Catal. 10, 302–310. doi:10.1021/acscatal.9b04309
Keywords: MOFs, CO2 hydrogenation, methanol, methane, C2+ Products
Citation: Zhang Q, Wang S, Dong M and Fan W (2022) CO2 Hydrogenation on Metal-Organic Frameworks-Based Catalysts: A Mini Review. Front. Chem. 10:956223. doi: 10.3389/fchem.2022.956223
Received: 30 May 2022; Accepted: 15 June 2022;
Published: 18 July 2022.
Edited by:
Haifeng Xiong, Xiamen University, ChinaCopyright © 2022 Zhang, Wang, Dong and Fan. This is an open-access article distributed under the terms of the Creative Commons Attribution License (CC BY). The use, distribution or reproduction in other forums is permitted, provided the original author(s) and the copyright owner(s) are credited and that the original publication in this journal is cited, in accordance with accepted academic practice. No use, distribution or reproduction is permitted which does not comply with these terms.
*Correspondence: Sen Wang, d2FuZ3NlbkBzeGljYy5hYy5jbg==; Weibin Fan, ZmFud2JAc3hpY2MuYWMuY24=