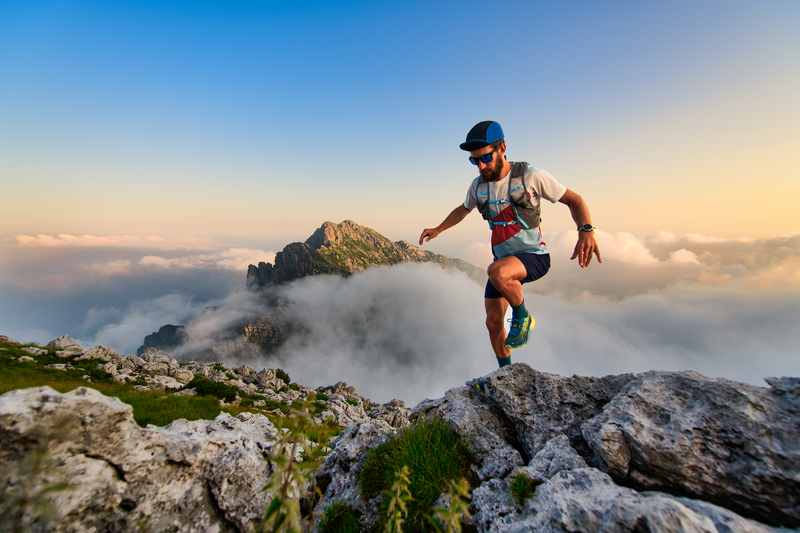
94% of researchers rate our articles as excellent or good
Learn more about the work of our research integrity team to safeguard the quality of each article we publish.
Find out more
ORIGINAL RESEARCH article
Front. Chem. , 05 August 2022
Sec. Electrochemistry
Volume 10 - 2022 | https://doi.org/10.3389/fchem.2022.954592
Aqueous rechargeable zinc-ion batteries (ZIBs) have been given more and more attention because of their high specific capacity, high safety, and low cost. The reasonable design of Mn-based cathode materials is an effective way to improve the performance of ZIBs. Herein, a square block MnCO3 electrode material is synthesized on the surface of carbon cloth by a one-step hydrothermal method. The phase transition of MnCO3 was accompanied by the continuous increase of specific capacity, and finally maintained good cycle stability in the charge–discharge process. The maximum specific capacity of MnCO3 electrode material can reach 83.62 mAh g−1 at 1 A g−1. The retention rate of the capacity can reach 85.24% after 1,500 cycles compared with the stable capacity (the capacity is 61.44 mAh g−1 under the 270th cycle). Ex situ characterization indicates that the initial MnCO3 gradually transformed into MnO2 accompanied by the embedding and stripping of H+ and Zn2+ in charge and discharge. When MnCO3 is no longer transformed into MnO2, the cycle tends to be stable. The phase transformation of MnCO3 could provide a new research idea for improving the performance of electrode materials for energy devices.
With the rapid development of information and intelligence in human society and the growth of new energy demand, secondary batteries play an important role in the application of new energy (Deng et al., 2021; Zhu et al., 2021). Lithium-ion batteries were developed for market application because of their excellent energy density, power density, and cycle life (Wang C et al., 2020; Hou et al., 2021; Hua et al., 2021). However, the further development of lithium-ion batteries is seriously restricted and challenged by their lack of resources, high cost, and potential safety hazards of organic electrolytes (Gan et al., 2020; Cai et al., 2021; Shan et al., 2021; Wang et al., 2021; Cui et al., 2022). The supplementary scheme of lithium-ion batteries has gradually become an urgent problem.
Aqueous zinc-ion batteries stand out among many electrochemical energy-storage devices because of their outstanding advantages such as safety, low cost, high-energy density, and environmental friendliness (Wang T et al., 2020; Du et al., 2020; Zhou and Guo, 2021). At present, the common cathode materials are V-based materials (Li J et al., 2020; Zhang L et al., 2020; Deng et al., 2020; Zhang et al., 2021), Mn-based materials (Wang J et al., 2020; Tan et al., 2020; Mao et al., 2021), and Prussian blue (Li Z et al., 2020). Among them, Mn-based oxides are widely used as cathode materials for aqueous zinc-ion batteries due to their low cost and abundant crystal structures (MnO, MnO2, Mn2O3, Mn3O4, etc.) (Zhao et al., 2019a; Gao et al., 2020). In 2012, Xu proposed a safe and environmentally friendly battery, which was made with α-MnO2 as cathode, zinc plate as anode, and ZnSO4 aqueous as electrolyte. It is first indicated that the Zn2+ intercalation and desorption mechanism based on Zn2+ was inserted into α-MnO2 (Xu et al., 2012). Ji et al. reported a multi-valence cobalt-doped Mn3O4 with high capacity (Ji et al., 2020). Zhu et al. reported the activation of MnO by inducing Mn defects, wherein the Mn defects are formed through a charging process that converts the MnO with poor electrochemical activities toward Zn2+ into high electrochemically active cathode for aqueous ZIBs (Zhu C et al., 2020). However, the diversity of valence states of Mn-based oxides will lead to more side reactions, resulting in irreversible phase transition in the reaction process. It will damage the cycle stability and capacity. Thus, the new material system needs to be further excavated.
In recent years, the crystal structure of MnCO3 is similar to that of MnO2 composed of [MnO6] and CO32−; generally, MnO2 is synthesized through the decomposition of Mn-based oxyacid salt and hydroxide. However, this process is more complicated and energy consuming than that of MnCO3. Consequently, MnCO3 has been gradually used in electrochemical energy-storage devices due to its rich reserves, environmental friendliness, and simple synthesis (Zhong et al., 2015; Li et al., 2018). It is a potential high-performance anode material for electrochemical energy-storage devices (Yao et al., 2021). Yao et al. reported an electrodeposited MnCO3 as a high-performance electrode material for supercapacitors (Yao et al., 2021). Liu et al. reported in situ N-doped MnCO3 anode material via one-step solvothermal for lithium-ion batteries (LIBs) (Liu et al., 2020). Zhao et al. reported that when MnCO3–RGO composite anode materials are used as anode material, they deliver a large capacity of 873 mAh g−1 even after 400 cycles at 1°C (Zhao et al., 2019b). However, their chemical properties are more active, and charge transfer occurs in the process of phase transition, which provides a better cycle stability. At present, the application of MnCO3 for ZIBs is still very rare, and its zinc storage mechanism has not been deeply explored.
Herein, we synthesized a square block MnCO3 electrode material on the surface of carbon cloth by the one-step hydrothermal method. The phase transition of MnCO3 was accompanied by the continuous increase of specific capacity and finally maintained good cycle stability in the charge–discharge process. The maximum specific capacity of MnCO3 electrode material can reach 83.62 mAh g−1 at 1 A g−1. The retention rate of the capacity can reach 85.24% after 1,500 cycles compared with the stable capacity (the capacity is 61.44 mAh g−1 under the 270th cycle). Ex situ characterization indicates that the initial MnCO3 gradually transformed into MnO2 accompanied by the embedding and stripping of H+ and Zn2+ in charge and discharge. When MnCO3 is no longer transformed into MnO2, the cycle tends to be stable. The phase transformation of MnCO3 could provide a new research idea for improving the performance of electrode materials for energy devices.
The prepared empty carbon cloth (CC) was immersed in a beaker containing concentrated nitric acid and heated in a water bath at 80°C for 2–3 h, then repeatedly washed with deionized water and absolute ethanol, and finally dried for future use. After pretreatment of empty CC, 10 mmol Mn(CH3COO)2, 50 mmol urea, and 40 ml of deionized water were added to 100 ml of Teflon lining. After stirring for 5 min, putting a 3 cm*2 cm carbon cloth, the mixed solution was placed in an autoclave and kept at 100°C for 16 h. The carbon cloth after the reaction was washed three times with deionized water and ethanol, and the sample (MnCO3/CC) was obtained after drying at 80°C for one night.
The crystallographic features of the as-prepared samples were characterized by X-ray diffraction (XRD, Philips X'Pert PRO, Cu Kα, λ = 0.1542 nm). The morphology and detailed microstructure were conducted on a scanning electron microscope (SEM, FEI Quanta 200) and transmission electron microscopy (TEM, Philips, Tecnai G220), coupled with energy-dispersive X-ray spectroscopy (EDS). The element composition and surface chemical state were recorded on an X-ray photoelectron spectroscope (XPS, Kratos AXIS Ultra DLD-600W).
In this experiment, the cathode material was MnCO3 grown in situ with carbon cloth, and the anode material was selected from commercial zinc foils with high purity. The electrolyte was 2 M zinc sulfate, and 0.2 M manganese sulfate was added as the electrolyte compensation. The two electrodes were separated by a glass fiber separator. Cyclic voltammetry (CV) and galvanostatic charge–discharge (GCD) were performed on the ChenHua electrochemical workstation (CHI760E) to test the electrochemical performance and used the eight-channel battery test equipment (NEWARE) to test rate performance and cycle life.
The micro-morphology of MnCO3@CC was characterized by a scanning electron microscope (SEM), as shown in Figure 1. Figures 1A–D show that the as-synthesized MnCO3 cubes are grown evenly on the carbon cloth, and the surface of MnCO3 is relatively smooth. Figures 1F–H show the EDS element distribution diagram of C, Mn, and O, respectively. The results are basically consistent with the MnCO3. The crystal structure of the sample was analyzed by XRD, as shown in Figure 2A. The diffraction peaks can be well indexed to the representative peaks of the MnCO3 phase (JCPDS 44-1,472) (Zhu L et al., 2020; Zhu J et al., 2020). In detail, the peaks at 24.25°, 31.36°, 37.52°, 41.42°, 45.18°, 49.67°, 51.68°, 60.13°, 63.88°, and 67.70° correspond to (012), (104), (110), (113), (202), (024), (116), (122), (214), and (300) crystal planes of MnCO3, respectively. In addition, there are no other heteropeaks in the XRD spectrum, which prove that the material we synthesized is pure MnCO3 cathode material. Previously, EDS and XRD confirmed that there were three elements, Mn, C, and O, in the cathode material synthesized by the hydrothermal method in the experimental process, and the phase accorded with the diffraction results of MnCO3.
In order to further confirm the structure of the samples, the valence states of the synthetic materials were analyzed by XPS. Figure 2B demonstrates two typical Mn2p1/2 and Mn2p3/2 orbitals of the MnCO3 phase. The binding energies of the two main peaks are 641.02 and 653.04 eV, with a difference of 12.02 eV, indicating that the valence of Mn in the compound is +2 (Zhang B et al., 2020). In the C1s spectrum in Figure 2C, the binding energies of the two main peaks are 284.3 and 288.9 eV, corresponding to C–C and C = O. In Figure 2D, the binding energy corresponding to the O1s is 530.3 eV, which further illustrates that O and C are combined and are expressed in the form of CO32− (Wang N et al., 2020). Supplementary Figure S1 shows the representative XPS survey spectrum of the sample, which verifies the presence of Mn, O, and C elements, indicating that the sample synthesized in the experiment is MnCO3 without other impurities. This conclusion is consistent with the XRD results in Figure 2A.
The electrochemical performance of MnCO3@CC cathode material is carried out in the coin cell, which utilized zinc foil as the anode and 2 M ZnSO4 + 0.2 M MnSO4 as the electrolyte. Cyclic voltammetry (CV) curves of unactivated MnCO3@CC are obtained at the scan rate of 0.5 mV/s under 0.4–2.0 V, as shown in Figure 3A. In the first cycle, the oxidation potential is 1.63 V, corresponding to two reduction potentials of 1.20 and 1.33 V, respectively. In the second cycle, the original oxidation potential shifted to the left to 1.64 V, while the second oxidation potential appeared at 1.69 V and the reduction potential remained at 1.20 and 1.33 V, respectively. There are also two oxidation potentials in the third circle, which are 1.65 and 1.69 V, respectively. The reduction potential is consistent with the first two cycles, and their response current and peak intensity further increase. The change of oxidation potential should be caused by the phase transition process of Mn ions, corresponding to Mn3+ to Mn4+, indicating that there is a gradual activation process in the initial stage. The two reduction peaks are mainly attributed to the embedding and stripping behavior of H+ and Zn2+. Galvanostatic charge–discharge (GCD) curves under different cycles at 0.1 A g−1 are shown in Figure 3B. There is only one discharge platform in 1.33 V under 1, 5, 10, 20, and 50 cycles, which is due to the embedding behavior of Zn2+ in the reaction process after the cathode material reaches a stable phase. Figure 3C shows the CV curves of MnCO3@CC at different scanning rates from 0.5 mV/s to 5 mV/s after activation. It is indicated that the response current gradually increases with the increase of the scanning rate. Figure 3D shows GCD curves under different current densities. The specific capacity is the highest at 0.1 A g−1, but the Coulomb efficiency is the lowest because the phase change is the most obvious at this stage, which is basically consistent with the previous conclusions.
FIGURE 3. (A) CV curves of the first three cycles of MnCO3 at 0.5 mV/s. (B) Galvanostatic charge–discharge (GCD) curves under different cycles. (C) CV curves of MnCO3 at different scan rates (0.5–5 mV/s). (D) GCD curves under different current densities. (E) The cycle performance of MnCO3 at 0.2 A g−1. (F) Rate performance of MnCO3. (G) The cycle performance of MnCO3 at 2.0 A g−1.
After the first cycle, Figure 3E shows the cyclic test of MnCO3 at 0.2 A g−1. Because the cathode material reaches a stable state after the activation process, its capacity remains stable during the following cycling. The first cycle capacity of the cathode material is 114.82 mAh g−1, the cycle capacity of the 50th cycle is 99.26 mAh g−1, and the retention rate is 87%. Figure 3F shows the rate performances of MnCO3 at 0.1, 0.2, 0.3, 0.5, 1.0, 2.0, 3.0, and 5.0 A g−1 in 0.4–2.0 V. The specific capacities are 164.17, 108.30, 91.52, 72.64, 56.23, 43.24, 36.59, and 28.89 mAh g−1, respectively. At low current density, the capacity of MnCO3@CC begins to remain stable after reaching a stable phase. In the subsequent current density, its capacity remains basically stable, indicating that the MnCO3@CC has good stability and rate performance under different current densities. Figure 3G shows the cycle performance of MnCO3/CC at 2.0 A g−1. At the higher current density, the cycle curve also rises slowly at first and then remains stable. The initial capacity of the MnCO3@CC is also low. After 400 cycles, the capacity reaches 48.46 mAh g−1 and then remains stable. After 1,700 cycles, when the capacity is relatively stable, the retention rate can reach 112.5% compared to the 400th cycle, which proves the good stability of the material.
To further explore the phase change and ion intercalation mechanisms of MnCO3@CC cathode material, the electrodes were tested by EX-XRD, SEM, and XPS in different stages of charge and discharge. Figure 4 shows the EX-XRD patterns of nanocube MnCO3@CC at 0.1 A g−1. In the initial (position 1), only the original peak of MnCO3 exists on the electrode (Figure 4A). When charging to 1.68 and 2.0 V, the peak of MnO2 appears near 36.8°, but its peak intensity is weak, indicating that little amount of MnCO3 changes into MnO2 during charging. In the discharge, no strong peak of MnCO3 is observed at positions 6, 7, and 8 in Figure 4A, but the MnO2 diffraction peak is obvious near 36.8°, which indicated that MnO2 is generated by the phase transformation of some MnCO3. When discharging to 0.3 V, the results show that the ZnMn2O4 phase appears near 32.9° and 38.9°, indicating that Zn2+ embedding behavior occurs in this process. In the charging process (Figure 4B), the diffraction peak deviates to the left between 30° and 34°, 36° and 38°, 44° and 46°, and 51° and 53°, and the crystal plane spacing becomes smaller, which is affected by the stripping behavior of Zn2+. Supplementary Figure S2 shows the SEM images of the electrode charging to 2.0 V and discharging to 0.3 V at 0.1 A g−1. Supplementary Figures S2A–S2C are the SEM images of charging to 2.0 V. After the original reaction, the smooth surface of MnCO3 is gradually rough, and the cube is stacked by sheets. Supplementary Figures S2D–S2E are the SEM images after discharging to 0.3 V. The results show that there are lines on the surface of cube edges and corners, which increases its specific surface area. It is conducive to the ion transmission between the host and Zn2+ and improves the electrochemistry performance of the cathode material.
FIGURE 4. (A) EX-XRD patterns of MnCO3@CC at 0.1 A g−1. (B) Angular shifts of different diffraction peaks. (C–E) The XPS patterns of MnCO3@CC for Mn, C, and Zn elements under charge to 2.0 V at 0.1 A g−1. (F–H) The XPS patterns of MnCO3@CC for Mn, C, and Zn elements under discharge to 0.3 V at 0.1 A g−1.
Figures 4C–E show the Mn, C, and Zn XPS spectrums of MnCO3@CC cathode material under charging to 2.0 V at 0.1 A g−1. Figure 4C shows the high-resolution Mn2p spectrum. These two groups of spin-orbit resolution peaks can be decomposed into Mn2+ (2p3/2, 641.4 eV; 2p1/2, 653.2 eV) and Mn4+ (2p3/2, 643.2 eV; 2p1/2, 655.6 eV). The content of Mn2+ is much greater than that of Mn4+ because only a small amount of MnCO3 is transformed into MnO2 in the charging stage, and a large number of cathode materials have not been activated, resulting in two valence states of +2 and +4 at the same time, and the proportion difference is large. The high-resolution spectrum of element C shown in Figure 4D is consistent with the reaction process. Figure 4E shows the high-resolution spectrum of Zn. The existence of Zn2+ (2p3/2, 1,044.5 eV; 2p1/2, 1,021.4 eV) is caused by the residue of ZnSO4 in the electrolyte.
The sample was discharged to 0.3 V for XPS analysis at 1.0 A g−1. In the high-resolution spectrum of Mn2p shown in Figure 4F, the two groups of spin orbits can be decomposed into Mn3+(2p3/2, 642.4 eV; 2p1/2, 654.2 eV) and Mn4+ (2p3/2, 644.1 eV; 2p1/2, 655.6 eV). The existence of Mn3+ and Mn4+ further proved the formation of MnO2 during charging and the embedding of Zn2+ in MnO2 during discharge, and ZnMn2O4 with spinel structure was formed. Due to the low content of MnO2 generated in the charging stage and since only part of MnO2 reacts with Zn2+ in the discharge process, the content of Mn3+ should be much less than Mn4+. Mn2+ was not detected because the generated MnO2 covered the original material, so its valence state could not be detected. Figure 4H shows the high-resolution spectrum of Zn2p. Zn is +2 valence in the test sample, which is consistent with the embedding behavior of Zn2+.
In order to further explore the activation mechanism and ion intercalation/desorption behavior of the MnCO3/CC, the batteries were analyzed after 10 cycles at a low current density of 0.1 A g−1. Figure 5A shows the EX-XRD patterns of charging to 2.0 V and discharging to 0.3 V after 10 cycles. After charging, the original MnCO3 diffraction peak gradually disappears, while the MnO2 characteristic peak becomes more and the peak intensity becomes stronger. In the discharge stage, a large amount of Zn2+ is embedded in MnO2 rather than MnCO3 and transformed into the spinel ZnMn2O4. The diffraction peak of ZnMn2O4 is positively correlated with MnO2 content, which is also the reason for the continuous increase in the electrochemical capacity of cathode materials. When MnCO3 is not in a phase transition to MnO2, the cycle curve reaches stability. Figure 5B shows the SEM image of the MnCO3@CC charged to 2.0 V after 10 cycles. The initial MnCO3@CC smooth cube block edges and corners basically disappear, and cracks appear on the surface. The change of morphology is conducive to expand the contact area between Zn2+ and the host, reduce the ion and charge transmission path and resistance, and then improve performance. Figure 5C shows the SEM image of the MnCO3@CC discharged to 0.3 V after 10 cycles. The result shows that the carbon cloth is covered by nano-spheres, the original nanocube basically disappears, and the specific surface area continues to increase.
FIGURE 5. (A) The XRD pattern of MnCO3@CC in charge to 2.0 V and discharge to 0.3 V at 0.1 A g−1 after 10 cycles. (B) The SEM image of MnCO3@CC under charge to 2.0 V at 0.1 A g−1 after 10 cycles. (C)The SEM image of MnCO3@CC under discharge to 0.3 V at 0.1 A g−1 after 10 cycles. (D–F) The XPS patterns of MnCO3@CC for Mn, C, and Zn elements under charge to 2.0 V at 0.1 A g−1 after 10 cycles. (G–I) The XPS patterns of MnCO3@CC for Mn, C, and Zn elements under discharge to 0.3 V at 0.1 A g−1 after 10 cycles.
The gradual change of morphology is also the reason for the continuous increase of specific capacity. The XPS patterns of Mn are shown in Figure 5D under charging to 2.0 V after 10 cycles. The two groups of spin orbits can be decomposed into Mn2+ (2p3/2, 641.5 eV; 2p1/2, 653.8 eV) and Mn4+ (2p3/2, 641.5 eV; 2p1/2, 653.8 eV), and the contents of Mn2+ and Mn4+ are opposite to the results after the first cycle. At this time, the proportion of Mn4+ is much larger than Mn2+, which further proves that MnCO3 gradually changes into MnO2. The high-resolution spectrum of Mn2p is shown in Figure 5H, the two groups of spin orbits can be decomposed into Mn3+ (2p3/2, 642.3 eV; 2p1/2, 654.2 eV) and Mn4+ (2p3/2, 644.2 eV; 2p1/2, 655.8 eV). After long-time activation, a large number of MnCO3 change into MnO2, and the increase of MnO2 gradually increases the content of discharge product ZnMn2O4. Therefore, the proportion of Mn3+ and Mn4+ is basically the same. The changes in valence and content further prove the mechanism that the MnCO3 first changes into MnO2, and then the generated MnO2 reacts with Zn2+ in the electrolyte.
The MnCO3 nanocubes are synthesized on carbon cloth by the one-step hydrothermal method, which has good electrochemical performance as cathode material for ZIBs. The specific capacity of the MnCO3/CC shows 82.73 mAh g−1 at 1.0 A g−1 after long-time activation. After 1,500 cycles, the capacity retention rate is 110.6% compared with that at 200 cycles, which indicates that the MnCO3@CC has excellent stability in the charging and discharging process. The Zn2+ storage mechanism of the MnCO3@CC was explored by in situ SEM, XRD, and XPS. In the initial stage, MnCO3 transformed into MnO2, and the generated MnO2 reacted with Zn2+ in the electrolyte. In the discharge stage, the spinel ZnMn2O4 is gradually formed in the cathode with the embedding of Zn2+. In the charging stage, the ZnMn2O4 is gradually transformed into MnO2 with the removal of Zn2+. In this study, the MnCO3 cathode material can achieve high specific capacity and cycle stability, which provides a new idea for high-performance aqueous zinc-ion batteries.
The original contributions presented in the study are included in the article/Supplementary Material; further inquiries can be directed to the corresponding authors.
JZ and PL: Data curation, experimental operation, and writing—original draft. JY, YG, JL, and CW: Conceptualization, visualization, and formal analysis. XL, GM, LL, HW, LT, and JZ: Writing—review and editing. YR and HW: Funding acquisition, project administration, and supervision.
This study was supported by the National Natural Science Foundation of China (No. 52002122), the Project funded by the China Postdoctoral Science Foundation (No. 2021M690947), and the application Fundamental Research Project of Wuhan Science and Technology Bureau (No. 2019010701011396).
The authors declare that the research was conducted in the absence of any commercial or financial relationships that could be construed as a potential conflict of interest.
The handling editor YR declared a past co-authorship with the author LL.
All claims expressed in this article are solely those of the authors and do not necessarily represent those of their affiliated organizations, or those of the publisher, the editors, and the reviewers. Any product that may be evaluated in this article, or claim that may be made by its manufacturer, is not guaranteed or endorsed by the publisher.
The Supplementary Material for this article can be found online at: https://www.frontiersin.org/articles/10.3389/fchem.2022.954592/full#supplementary-material
Cai, P., Zou, K., Deng, X., Wang, B., Zheng, M., Li, L., et al. (2021). Comprehensive understanding of sodium-ion capacitors: Definition, mechanisms, configurations, materials, key technologies and future developments. Adv. Energy Mater 11, 2003804. doi:10.1002/aenm.202003804
Cui, H., Ma, L., Huang, Z., Chen, Z., and Zhi, C. (2022). Organic materials-based cathode for zinc ion battery. SmartMat 3, 1–17. doi:10.1002/smm2.1110
Deng, S., Yuan, Z., Tie, Z., Wang, C., Song, L., and Niu, Z. (2020). Electrochemically induced metal-organic-framework-derived amorphous V2O5 for superior rate aqueous zinc-ion batteries. Angew. Chem. Int. Ed. 59, 22002–22006. doi:10.1002/anie.202010287
Deng, X., Zou, K., Momen, R., Cai, P., Chen, J., Hou, H., et al. (2021). High content anion (S/Se/P) doping assisted by defect engineering with fast charge transfer kinetics for high-performance sodium ion capacitors. Sci. Bull. 66, 1858–1868. doi:10.1016/j.scib.2021.04.042
Du, W., Ang, E. H., Yang, Y., Zhang, Y., Ye, M., and Li, C. C. (2020). Challenges in the material and structural design of zinc anode towards high-performance aqueous zinc-ion batteries. Energy Environ. Sci. 13, 3330–3360. doi:10.1039/d0ee02079f
Gan, Y., Wang, C., Chen, X., Liang, P., Wan, H., Liu, X., et al. (2020). High conductivity Ni12P5 nanowires as high-rate electrode material for battery-supercapacitor hybrid devices. Chem. Eng. J. 392, 123661. doi:10.1016/j.cej.2019.123661
Gao, X., Zhang, H., Liu, X., and Lu, X. (2020). Flexible Zn‐ion batteries based on manganese oxides: Progress and prospect. Carbon Energy 2, 387–407. doi:10.1002/cey2.63
Hou, S., Ji, X., Gaskell, K., Wang, P.-f., Wang, L., Xu, J., et al. (2021). Solvation sheath reorganization enables divalent metal batteries with fast interfacial charge transfer kinetics. Science 374, 172–178. doi:10.1126/science.abg3954
Hua, X., Eggeman, A. S., Castillo-Martínez, E., Robert, R., Geddes, H. S., Lu, Z., et al. (2021). Revisiting metal fluorides as lithium-ion battery cathodes. Nat. Mat. 20, 841–850. doi:10.1038/s41563-020-00893-1
Ji, J., Wan, H., Zhang, B., Wang, C., Gan, Y., Tan, Q., et al. (2020). Co2+/3+/4+‐Regulated electron state of Mn‐O for superb aqueous zinc‐manganese oxide batteries. Adv. Energy Mat. 11, 2003203. doi:10.1002/aenm.202003203
Li, J., McColl, K., Lu, X., Sathasivam, S., Dong, H., Kang, L., et al. (2020). Multi‐scale investigations of δ‐Ni0.25V2O5·nH2O cathode materials in aqueous zinc‐ion batteries. Adv. Energy Mat. 10, 2000058. doi:10.1002/aenm.202000058
Li, Q., Liu, Z., Wang, C., Zhao, Y., and Che, R. (2018). Doping of Ni and Zn elements in MnCO3: High-power anode material for lithium-ion batteries. Small 14, 1702574. doi:10.1002/smll.201702574
Li, Z., Liu, T., Meng, R., Gao, L., Zou, Y., Peng, P., et al. (2020). Insights into the structure stability of prussian blue for aqueous zinc ion batteries. Energy Environ. Mat. 4, 111–116. doi:10.1002/eem2.12108
Liu, M., Wang, Q., Liu, Z., Zhao, Y., Lai, X., Bi, J., et al. (2020). In-situ N-doped MnCO3 anode material via one-step solvothermal synthesis: Doping mechanisms and enhanced electrochemical performances. Chem. Eng. J. 383, 123161. doi:10.1016/j.cej.2019.123161
Mao, M., Wu, X., Hu, Y., Yuan, Q., He, Y.-B., and Kang, F. (2021). Charge storage mechanism of MOF-derived Mn2O3 as high performance cathode of aqueous zinc-ion batteries. J. Energy Chem. 52, 277–283. doi:10.1016/j.jechem.2020.04.061
Shan, L., Wang, Y., Liang, S., Tang, B., Yang, Y., Wang, Z., et al. (2021). Interfacial adsorption-insertion mechanism induced by phase boundary toward better aqueous Zn‐ion battery. InfoMat 3, 1028–1036. doi:10.1002/inf2.12223
Tan, Q., Li, X., Zhang, B., Chen, X., Tian, Y., Wan, H., et al. (2020). Valence engineering via in situ carbon reduction on octahedron sites Mn3O4 for ultra‐long cycle life aqueous Zn‐ion battery. Adv. Energy Mat. 10, 2001050. doi:10.1002/aenm.202001050
Wang, C., Song, Z., Wan, H., Chen, X., Tan, Q., Gan, Y., et al. (2020). Ni-Co selenide nanowires supported on conductive wearable textile as cathode for flexible battery-supercapacitor hybrid devices. Chem. Eng. J. 400, 125955. doi:10.1016/j.cej.2020.125955
Wang, J., Wang, J. G., Liu, H., You, Z., Li, Z., Kang, F., et al. (2020). A highly flexible and lightweight MnO2/graphene membrane for superior zinc‐ion batteries. Adv. Funct. Mat. 31, 2007397. doi:10.1002/adfm.202007397
Wang, N., Ma, S., Duan, J., Zhai, X., Guan, F., Wang, X., et al. (2020). Electrocatalytic oxygen reduction to hydrogen peroxide by oxidized graphene aerogel supported cubic MnCO3 for antibacteria in neutral media. Electrochimica Acta 340, 135880. doi:10.1016/j.electacta.2020.135880
Wang, N., Wan, H., Duan, J., Wang, X., Tao, L., Zhang, J., et al. (2021). A review of zinc-based battery from alkaline to acid. Mater. Today Adv. 11, 100149. doi:10.1016/j.mtadv.2021.100149
Wang, T., Li, C., Xie, X., Lu, B., He, Z., Liang, S., et al. (2020). Anode materials for aqueous zinc ion batteries: Mechanisms, properties, and perspectives. ACS Nano 14, 16321–16347. doi:10.1021/acsnano.0c07041
Xu, C., Li, B., Du, H., and Kang, F. (2012). Energetic zinc ion chemistry: The rechargeable zinc ion battery. Angew. Chem. Int. Ed. 51, 933–935. doi:10.1002/anie.201106307
Yao, J., Wan, H., Chen, C., Ji, J., Wang, N., Zheng, Z., et al. (2021). Oxygen-defect enhanced anion adsorption energy toward super-rate and durable cathode for Ni-Zn batteries. Nano-Micro Lett. 13, 167. doi:10.1007/s40820-021-00699-z
Zhang, B., Li, X., Zou, J., and Kim, F. (2020). MnCO3 on graphene porous framework via diffusion-driven layer-by-layer assembly for high-performance pseudocapacitor. ACS Appl. Mat. Interfaces 12, 47695–47703. doi:10.1021/acsami.0c15511
Zhang, L., Hu, J., Zhang, B., Liu, J., Wan, H., Miao, L., et al. (2021). Suppressing cathode dissolution via guest engineering for durable aqueous zinc-ion batteries. J. Mat. Chem. A 9, 7631–7639. doi:10.1039/d1ta00263e
Zhang, L., Miao, L., Zhang, B., Wang, J., Liu, J., Tan, Q., et al. (2020). A durable VO2(M)/Zn battery with ultrahigh rate capability enabled by pseudocapacitive proton insertion. J. Mat. Chem. A 8, 1731–1740. doi:10.1039/c9ta11031c
Zhao, Y., Mu, Y., Wang, L., Liu, M., Lai, X., Bi, J., et al. (2019a). MnCO3-RGO composite anode materials: In-situ solvothermal synthesis and electrochemical performances. Electrochimica Acta 317, 786–794. doi:10.1016/j.electacta.2019.06.001
Zhao, Y., Zhu, Y., and Zhang, X. (2019b). Challenges and perspectives for manganese‐based oxides for advanced aqueous zinc‐ion batteries. InfoMat 2, 237–260. doi:10.1002/inf2.12042
Zhong, Y., Yang, M., Zhou, X., Luo, Y., Wei, J., and Zhou, Z. (2015). Orderly packed anodes for high-power lithium-ion batteries with super-long cycle life: Rational design of MnCO3/large-area graphene composites. Adv. Mat. 27, 806–812. doi:10.1002/adma.201404611
Zhou, J., and Guo, S. (2021). Carbon‐based anode materials for potassium‐ion batteries: From material, mechanism to performance. SmartMat 2, 176–201. doi:10.1002/smm2.1042
Zhu, C., Fang, G., Liang, S., Chen, Z., Wang, Z., Ma, J., et al. (2020). Electrochemically induced cationic defect in MnO intercalation cathode for aqueous zinc-ion battery. Energy Storage Mater. 24, 394–401. doi:10.1016/j.ensm.2019.07.030
Zhu, G., Tian, X., Tai, H.-C., Li, Y.-Y., Li, J., Sun, H., et al. (2021). Rechargeable Na/Cl2 and Li/Cl2 batteries. Nature 596, 525–530. doi:10.1038/s41586-021-03757-z
Zhu, J., Wei, P., Zeng, Q., Wang, G., Wu, K., Ma, S., et al. (2020). MnS@N,S Co-doped carbon core/shell nanocubes: Sulfur-bridged bonds enhanced Na-storage properties revealed by in situ Raman spectroscopy and transmission electron microscopy. Small 16, e2003001. doi:10.1002/smll.202003001
Keywords: MnCO3, doping, zinc-ion battery, phase transformation, cyclic stability
Citation: Zheng J, Liu P, Yao J, Gan Y, Li J, Wang C, Liu X, Rao Y, Ma G, Lv L, Wang H, Tao L, Zhang J and Wang H (2022) Phase transformation mechanism of MnCO3 as cathode materials for aqueous zinc-ion batteries. Front. Chem. 10:954592. doi: 10.3389/fchem.2022.954592
Received: 27 May 2022; Accepted: 27 June 2022;
Published: 05 August 2022.
Edited by:
Yunjun Ruan, Guizhou University, ChinaReviewed by:
Pei Liang, China Jiliang University, ChinaCopyright © 2022 Zheng, Liu, Yao, Gan, Li, Wang, Liu, Rao, Ma, Lv, Wang, Tao, Zhang and Wang. This is an open-access article distributed under the terms of the Creative Commons Attribution License (CC BY). The use, distribution or reproduction in other forums is permitted, provided the original author(s) and the copyright owner(s) are credited and that the original publication in this journal is cited, in accordance with accepted academic practice. No use, distribution or reproduction is permitted which does not comply with these terms.
*Correspondence: Yiheng Rao, eWloZW5ncmFvQDE2My5jb20=; Jun Zhang, Z3dlbl96aGFuZ0AxMjYuY29t
†These authors have contributed equally to this work
Disclaimer: All claims expressed in this article are solely those of the authors and do not necessarily represent those of their affiliated organizations, or those of the publisher, the editors and the reviewers. Any product that may be evaluated in this article or claim that may be made by its manufacturer is not guaranteed or endorsed by the publisher.
Research integrity at Frontiers
Learn more about the work of our research integrity team to safeguard the quality of each article we publish.