- 1Key Laboratory of Biocatalysis and Chiral Drug Synthesis of Guizhou Province, Green Pharmaceuticals Engineering Research Center of Guizhou Province, Zunyi Medical University, Zunyi, China
- 2Key Laboratory of Basic Pharmacology of Ministry of Education, Joint International Research Laboratory of Ethnomedicine of Ministry of Education, Zunyi Medical University, Zunyi, China
Chiral allylic oxidized products play an increasingly important role in the pharmaceutical, agrochemical, and pharmaceutical industries. Biocatalytic C–H oxyfunctionalization to synthesize allylic oxidized products has attracted great attention in recent years, with the ability to simplify synthetic approaches toward complex compounds. As a result, scientists have found some new enzymes and mutants through techniques of gene mining and enzyme-directed evolution in recent years. This review summarizes the recent developments in biocatalytic selective oxidation of olefins by different kinds of biocatalysts.
Introduction
As the demand for oxygenated allylic intermediates in food, flavor and fragrance, cosmetics, and pharmaceuticals is increasing, the significance of this type of compound is gradually revealed (Casarano et al., 2005; Fanani et al., 2021; Raffo et al., 2012; Venancio et al., 2021; Ke et al., 2021). The reaction that converts alkenes to allyl alcohols has recently attracted particular interest. Due to containing two functional groups, including the hydroxyl group and olefins, this type of compound can participate in a variety of reactions such as oxidation, reduction, esterification, and addition (Sharpless and Michaelson., 1973; Church and Andersson., 2008; Pellissier, 2008) and can also be used for subsequent functional group transformation (Adam et al., 2000; Müller and Fruit, 2003; Hernández-Toribio et al., 2011) (Figure 1A). In addition, there is an increasing number of examples of allylic oxidation catalyzed by transition metals and co-oxidants to produce useful natural products; among them, the selenium and chromium reagents have been widely applied in natural product syntheses (Nakamura and Nakada, 2013). For example, (±)-Ingenol (1c) was synthesized with the use of tert-butyl hydroperoxide (TBHP) as a catalyst for allylic oxidation to prepare key alcohol intermediate (Figure 1B).
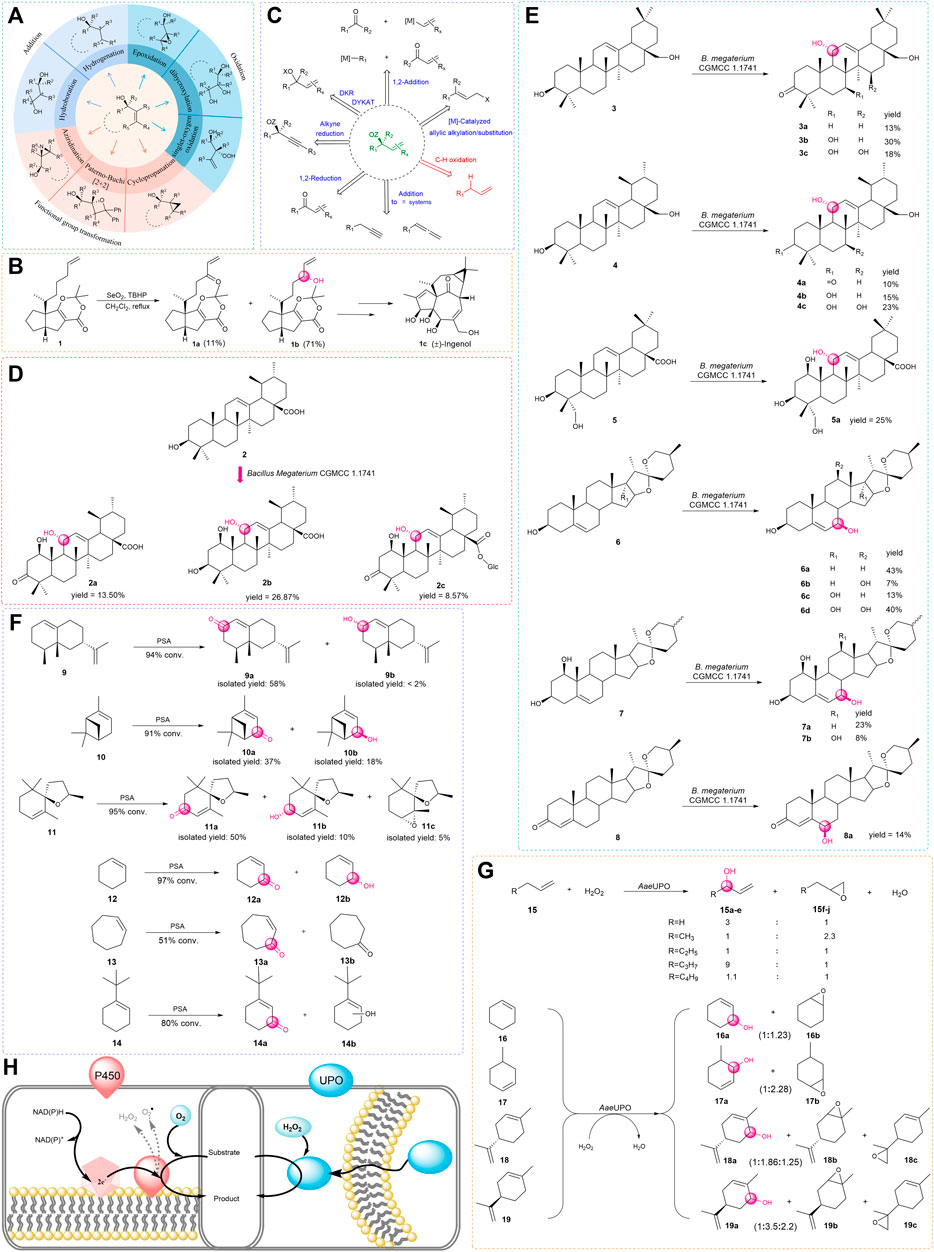
FIGURE 1. (A) Diastereoselective transformations of allylic alcohols. (B) Total synthesis of (±)-Ingenol. (C) Catalytic asymmetric access to enantio-enriched allylic alcohols. (D,E) Biotransformation of triterpenes and steroids by Bacillus megaterium. (F) Biocatalytic allylic oxidation by PSA. (G) Epoxidation competes with allylic hydroxylation in most cases. (H) P450s and UPOs are compared. (The red on the left is P450s which rely on auxiliary proteins and cofactors, the blue on the right is UPOs which require only hydrogen peroxide).
In the past few decades, there are several strategies available for the oxidation of allyl to allyl alcohols or allyl esters; both enzymatic and traditional organic chemical syntheses have made great contributions to the field (Lumbroso et al., 2013; Dong et al., 2018). With the development of the technology, efforts have been put together to set a goal to achieve attractive green, step- and atom-economical, and sustainable methods (Kim and Li. 2020). For this aim, enzyme-catalyzed oxidation with molecular oxygen and incorporation of the oxygen atom(s) into the allyl molecule has been emerging to be one of the most attractive research areas (Hamilton, 1974; Sono et al., 1996) (Figure 1C). First of all, this method generally avoids the use of toxic metals or expensive complex ligands and usually offers remarkable chemo-, regio-, and stereoselectivity (Jiang, 2020). Furthermore, enzymatic oxygenation is usually performed under mild reaction conditions, therefore avoiding the use of harsh conditions such as high temperature and pressure (Blank et al., 2010).
Combining the importance of allyl alcohol products with the advantages of enzyme-mediated catalysis, this review will discuss the recent advances in allylic selective oxidation by different enzymes to reveal the great achievements and potentials in this field.
Whole cell as a catalyst
Bacillus megaterium CGMCC 1.1741
Bacillus megaterium is a rod-shaped, gram-positive prokaryotic bacterium, which has offered a green method to modify natural products (König et al., 2019). Zhang and his coworkers found that Bacillus megaterium CGMCC 1.1741 could transform the ursolic acid (2) into three oxidation products. For ursolic acid (2), introducing a hydroxyl group is a key step because it provides a functional group for subsequent modifications. In this study (Zhang et al., 2017), a very unique pathway for the biosynthesis of pentacyclic triterpenes was presented. However, the bioconversion of ursolic acid (2) gave lots of byproducts, and the yield of the desired product is not satisfactory (Figure 1D).
Zhang found that Bacillus megaterium CGMCC 1.1741 had the ability to catalyze pentacyclic triterpenes and steroids, which was the first report about the one biotransformation culture that could catalyze allylic oxidation on both Δ12-triterpenes and Δ5-steroids (Wang et al., 2020). In further studies, the aforementioned products were shown to be inhibitors of sterol biosynthesis (Schroepfer Jr, 1981; Berthier et al., 2004; Okabe et al., 2014; Gargiulo et al., 2017), which offered promising candidates for the prevention and treatment of cancers (Zhao et al., 2015). These findings not only broaden the substrate scope but also provide direct access to prepare these vital products with high stereoselectivity in one step (Figure 1E). Since P450 enzymes may be responsible for allylic hydroxylation, studies of gene clone and protein characterization of the potential P450s from Bacillus megaterium CGMCC 1.1741 is in process.
Pleurotus sapidus
Berger’s group found that the white-rot fungus Pleurotus sapidus (PSA) could transform limonene into cis/trans-carveol and carvone (Onken and Berger, 1999). With further study on PSA, Holger Zorn and Wolfgang Maison proposed an effective way to transform valencene (9) into nootkatone (9a), which had a pleasant grapefruit-like aroma. Due to nootkatone (9a) being commonly used in the food and pharmaceutical industries, it is important that this type of compound is prepared in a biocatalytic manner (Fraatz et al., 2009; Krugener et al., 2010).
For example, the biocatalytic oxidation with PSA has a relatively wide range of substrates including several functionalized terpenoids such as α-pinene (10) and theaspirane (11) (Fraatz et al., 2009; Krugener et al., 2010; Rickert et al., 2012; Weidmann et al., 2015). In addition, PSA can also oxidize cyclohexene derivatives, but many reactions have limited regioselectivity, except that tert-butyl cyclohexene (14) was converted with good regioselectivity (Figure 1F).
Another point worthy of attention is that biocatalytic oxidations with PSA may be performed with the lyophilisate of the fungus. This provides a lot of convenience and is straightforward for the catalytic reaction. However, because the lyophilisate was used as a mixture of enzymes, oxidation of the substrate by pathways other than allylic hydroxylation might exist as the corresponding by-product was detected.
Unspecific peroxygenases
Eighteen years ago, Pamela Manzi and coworkers found an enzyme that could oxidize halides and aryl alcohols, but the enzyme was not named (Ullrich et al., 2004). A few years later, additional aromatic, heterocyclic, and aliphatic substrates were found to be oxidized by this enzyme (Hofrichter and Ullrich, 2006; Ullrich and Hofrichter, 2007; Hofrichter and Ullrich, 2011), therefore, the name of this enzyme is called unspecific peroxygenase (UPO, EC1.11.2.1), which is also known as aromatic peroxygenase (APO). APO was discovered in the basidiomycetous fungus, Agrocybe aegerita (AaeUPO) (Ullrich and Hofrichter, 2005). UPOs were approached as a functional monoperoxygenase which can catalyze many reactions that involve the transfer of an oxygen atom by reacting with hydrogen peroxide. The reactions catalyzed by this enzyme range from epoxidation, hydroxylation, dealkylation, aromatization, sulfoxidation to dechlorination, and halide oxidation (Hofrichter and Ullrich., 2014; Hofrichter et al., 2015; Wang et al., 2017). For hydroxylation, in 2013, Peter and coworkers employed the whole cells to convert a series of alkenes into the corresponding epoxides and hydroxyl products in one step (Figure 1G). In this work (Peter et al., 2013), UPOs could insert oxygen into aliphatic and aromatic compounds that contain non-activated carbon atoms (stereoselectivity data is not given), although the reaction tends to form the epoxidation products except for chain alkenes, they found a broad spectrum of substrates catalyzed by the AaeUPO is another gift.
Moreover, many results showed that the hydroxylation reaction preferentially occurred in the free methylene groups (Gutiérrez et al., 2011; Peter et al., 2011; Kluge et al., 2012). On the other hand, UPOs have similar advantages to cytochrome P450 monooxygenases (CYP or P450) that can mediate allylic oxidation reactions. First of all, UPOs are usually extracellular and soluble proteins, while the P450s are usually intermembrane proteins that are relatively less stable. In addition, during the catalytic process, UPOs need H2O2 as a cosubstrate for the reaction, while the P450s usually require auxiliary electron transport systems and cofactors (Figure 1H).
Although the catalytic mechanisms of P450s and UPOs are similar, there are some fundamental structural differences at the enzyme active sites which are important to form compound I (Cpd I). After the crystal structure of AaeAPO (Piontek et al., 2010) had been reported (Figure 2A), Hofrichter and coworkers studied the catalytic mechanism of the enzyme (Hofrichter and Ullrich., 2014). The structure has a heme-thiolate protein with acid-base residues of the distal glutamate 196 and arginine 189 at the active site. During the catalytic process, the iron-heme center activates the hydrogen peroxide first, then H2O2 reacts with the resting state UPO to form the compound 0 (Cpd 0). The complex is deprotonated by glutamate 196 by electron re-arrangement to give the key active Cpd I. Finally, this reactive species abstracts an H atom from the substrate to form a low degree of stereoselectivity for allylic hydroxylation reaction (Hrycay and Bandiera., 2012). In addition, UPOs are eukaryotic enzymes, and their expressions have been limited to yeasts in most circumstances (Wang, 2013; Molina-Espeja et al., 2014). In light of this, techniques of molecular biology have been used to resolve these issues, in particular the directed evolution.
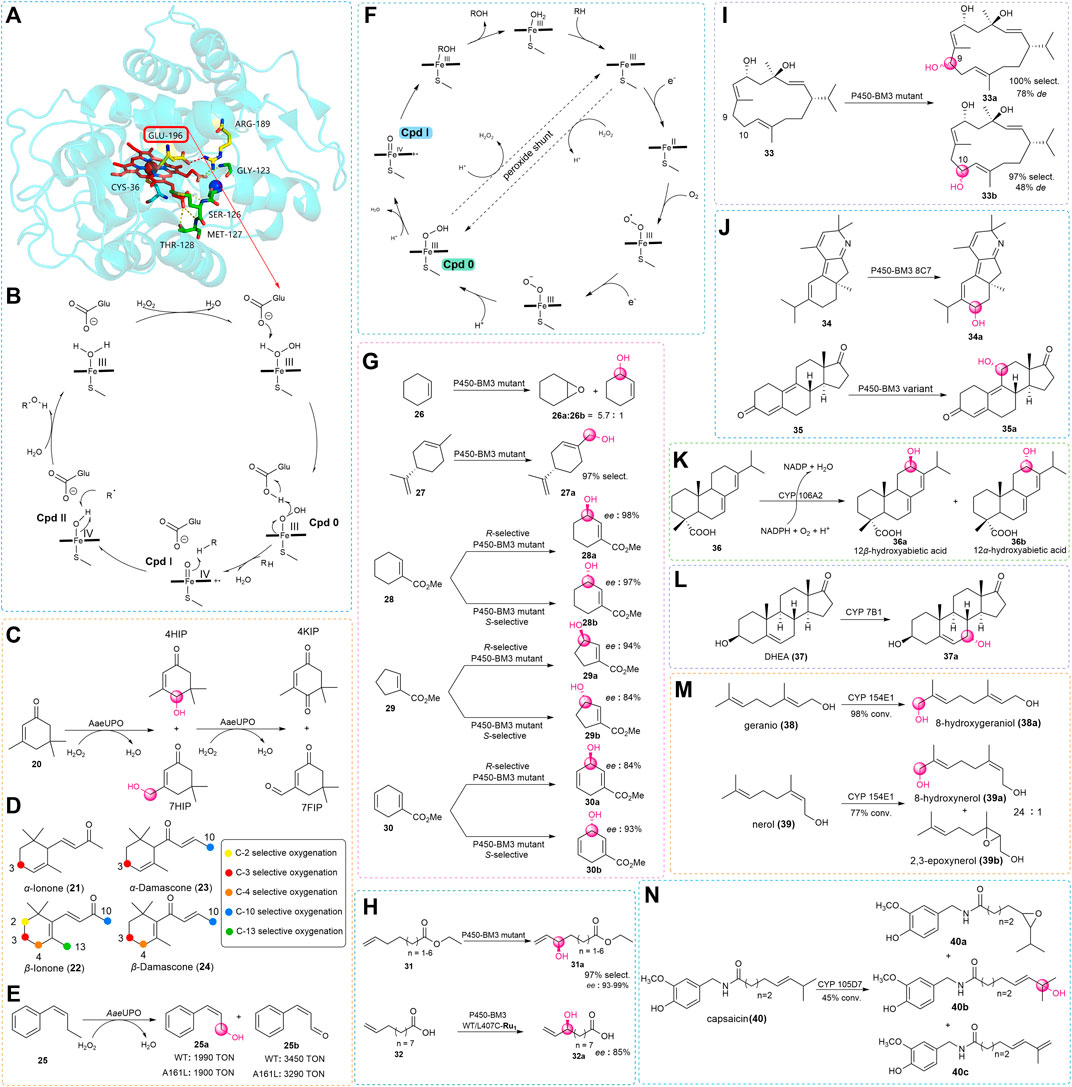
FIGURE 2. (A) Overall crystal structure of AaeAPO (Wang et al., 2017). (B) Catalytic mechanism of AaeAPO. (C) Isophorone hydroxylation and hyperoxidation catalyzed by AaeUPO. (D) Different colors indicate the positions where the substrate may be hydroxylated during UPO catalysis, obtaining the different oxygenated derivatives. (E) Bioconversion of the MthUPO mutant A161L gains the allylic oxidation products. (F) Oxidation reaction mechanism of P450s. (G) Biocatalytic allylic oxidation of cyclic olefins by P450-BM3 mutants. (H) Biocatalytic allylic oxidation of chain terminal alkenes by P450-BM3 mutants. (I) Potential oxidation sites of β-cembrenediol (32) by the P450-BM3 mutant. (J) Hydroxylation reaction of terpenoids (33) and steroids (34) by P450-BM3 mutants. (K) Biocatalytic allylic oxidation by CYP106A2. (L) Biocatalytic allylic oxidation of DHEA (36) by CYP 7B1. (M) Biocatalytic allylic oxidation of gernio (37) and nerol (38) by CYP 154E1. (N) Biocatalytic allylic oxidation of capsaicin (39) by CYP 105D7.
Directed evolution is constituted of random or semi-rational mutagenesis which is extremely successful for improved enzyme abilities and has led to many enzyme libraries for different substrates (Bornscheuer et al., 2012; Hammer et al., 2017; Qu et al., 2020; Li B. B et al., 2021; Li D et al., 2021).
For one thing, due to the UPOs being eukaryotic enzymes and usually being glycosylated, most examples of heterologous expression appear to require expression in yeasts such as Saccharomyces cerevisiae and Pichia pastoris which are closely related to the microorganism that the enzyme was isolated from, for full activity. However, for wild-type UPOs, the yield of functional expression in yeast is low. Alcalde group used five rounds of directed evolution of the AaeUPO to resolve this issue, which resulted in the functional expression in Saccharomyces cerevisiae (Molina-Espeja et al., 2019). Since Escherichia coli (E. coli) has the characteristics of fast amplification, easy cultivation and low cost of cultivation, expression in the E. coli has become a good choice for most heterologous expressions in the laboratory (Baeshen et al., 2015). Wang (2016) successfully purified the wild-type AaeUPO by its expression in E. coli, however, the recombinant protein showed no activity, because of the formation of inclusion bodies (Wang, 2016). With the development of the directed evolution, Martínez and co-workers obtained Collariella virescens and Daldinia caldariorum from the ascomycetes by exploring the heterologous expression system of UPOs. They found the mutants of them to be active when expressed in E. coli, but the enzyme activity was low, with 38.18 U/mg and 7.68 U/mg, respectively (Linde et al., 2020).
Moreover, earlier results showed that wild-type UPOs catalyzed linear alkenes with a preference for allylic hydroxylation reaction but showed low chemoselectivity toward branched and cyclic alkenes for the formation of epoxide products. With the increasing number of newly discovered enzymes and screened substrates, some novel and interesting results were obtained. Gutiérrez and co-workers discovered that the UPOs were capable of selectively hydroxylating isophorone (20) to 4-hydroxyisophorone (4HIP) and 4-ketoisophorone (4KIP), which are now used in the flavor-and-fragrance and pharmaceutical industries (Aranda et al., 2019). They used three UPO enzymes, biocatalytic isophorone (20) with different selectivities. The first enzyme is AaeUPO, which is the earliest wild-type UPO described in previous reports. The enzyme isolated from Marasmius rotula aromatic peroxygenase (MroAPO) is another wild-type enzyme (Grobe et al., 2011). Last, the UPO of Chaetomium globosum (CglUPO) is a third wild-type peroxygenase (Kiebist et al., 2017). In this work, they found the AaeUPO had poor regioselectivity, and in addition to 4HIP, the reaction also formed 7-hydroxyisophorone (and 7-formylisophorone), yet this enzyme has the most stereoselectivity which provided the S-4HIP with an enantiomeric excess (ee) of 88% (Figure 2C). Then, this group tried some new substrates and found UPOs could accomplish the oxyfunctionalization of ionones and damascones. The products of these reactions are essential compounds for the flavor and important building blocks for pharmaceutical industries. Similar to the previous method, they used a series of UPOs to react with α-ionone (21), β-ionone (22), α-damascone (23), and β-damascone (24). In test, most types of UPOs showed high conversion, and oxidation of various positions was observed (Figure 2D). With AaeUPO, the products with the highest oxidation yield occurred at the C-3 position for α-Ionone (21), α-Damascone (23), and β-Damascone (24), and at the C-4 position for β-Ionone (22). With MroUPO, the products with the highest oxidation yield occurred at the C-3 position for α-Ionone (21) and α-Damascone (23), and at the C-4 position for β-Ionone (22) and β-Damascone (24). With rCciUPO, the products with the highest oxidation yield occurred at the C-3 position for α-Ionone (21), at the C-4 position for β-Ionone (22), and at the C-10 position for α-Damascone (23). For this enzyme with (24), oxidation seemed to occur with no preference, with a yield greater than 99% obtained for positions C-3, C-4, and C-10, respectively. With CglUPO, the products with the highest oxidation yield occurred at the C-3 position for α-Ionone (21) and α-Damascone (23), at the C-4 position for β-Ionone (22) and β-Damascone (24). With rDcaUPO, the products with the highest oxidation yield occurred at the C-3 position for α-Ionone (21) and α-Damascone (23), at the C-4 position for β-Ionone (22) and β-Damascone (24).
Although the only variation between the two types of ionone is the position of the double bond, the α-Ionone (21) demonstrated better regioselectivity (up to 99%) than the β-Ionone (22), possibly because of the preference of the UPOs toward the allylic substrate. This preference exists on α- and β-Damascones as well, however, the same enzyme’s regioselectivity was not consistent with ionones. Among the tested reaction, the hydroxylation of AaeUPO happened primarily at the terminal position of the butenoyl side chain, whereas CglUPO did not yield this product at all (Babot et al., 2020). The results of this study demonstrated the unique regioselectivity for diverse UPOs. Moreover, a little change in the structure of the substrate might lead to different regioselective products. Except for wild-type enzymes, in recent years a growing body of research has revealed the charm of directed evolution with high throughput screening. Weissenborn and co-workers used the variants of MthUPO from Myceliophthora thermophile for enhancing the low selectivity with epoxidation of the double bond substrates such as cyclohexene and styrene. However, when methylstyrene (25) was used as the substrate (Knorrscheidt et al., 2021), it was surprising to find that the wild-type MthUPO and one of its variants, A161L, had excellent selectivity for allylic oxidation. This unexpected result provided an idea for discovering enzymes with new catalytic performances for allylic oxidation in the future (Figure 2E).
P450s
In recent decades, in order to comply with the principles of green chemistry, P450s are one of the most extensively studied biocatalysts. P450s are widely distributed in animals, plants, and microorganisms, which is a recognized multi-functional biological oxidation catalyst, and it has a wide source. The number of P450s from the bacteria has exceeded 62,000, and the number of P450s derived from the fungi has exceeded 85,000 species (Nelson, 2018). Furthermore, the enzymes catalyze the incorporation of molecular oxygen in the air into the substrate, which meets a green development. In addition, metalloenzymes have a heme-iron prosthetic group at the active site, which can overcome the barriers associated with the electronic structure of molecular oxygen (Jensen and Ryde., 2004). Although P450s have been shown to have great potential in biocatalysis, they require additional cofactors. In addition, they usually have the issues of poor stability and low solubility. These limit the industrial applications of enzymes. On one hand, with the development of catalytic self-sufficient P450, heme and reductase domains are fused in a polypeptide, which requires no additional redox partners that can avoid complex reaction conditions. On the other hand, substrates used for this field of study are generally hydrophobic, which usually causes substrate solubility issues, leading to relatively low transformation efficiencies. For this issue, several permeation methods are currently available, such as freeze and thaw, electropermeabilization, and using EDTA as a permeabilizer (Chen, 2007).
The activation of C–H bonds in allylic compounds has raised great interest in the chemical industry (Balcells et al., 2010). Due to the bonds in hydrocarbons being thermodynamically strong and usually kinetically inert, controlling the chemo-, regio-, and stereo-selectivities under mild conditions is rather difficult (Xue et al., 2017).
Considering these issues, one of the currently adopted methods for allylic oxidation is to use green and efficient enzyme catalysts, such as P450s (Urlacher and Girhard, 2019). It has been mentioned that the hydroxylation mechanism of UPOs and P450s is similar but is essentially different in terms of the formation of Cpd I (Figure 2F). In P450s, after the substrate binding to the enzyme active site, the heme iron accepts one electron of the cofactor to be reduced to Fe2+. Then the reduced heme iron reacts with molecular oxygen to generate a complex, which is reduced by another single electron and subsequently protonated to give Cpd 0. Then, the cleavage of the O–O bond brings in a water molecule to give the highly reactive Cpd I. Finally, Cpd I oxidizes the substrate which leads to the formation of the hydroxylation product and restores the resting state of the heme. In addition, some P450s can utilize the H2O2 as an oxygen source and promote the formation of Cpd I via the peroxide shunt pathway (Munro et al., 2013). Similar to the UPOs, this enzyme is also relatively sensitive to H2O2, which should be considered in reaction.
Miura and Fulco (1974) identified CYP102A1 from Bacillus megaterium, wildly known as fatty acid hydroxylase Cytochrome P450-BM3, as the first enzyme of this family in 1970 and has been an extensively studied self-sufficient P450 in the past few decades (Miura and Fulco., 1974). More and more studies on allylic hydroxylation have been described based on the crystal structure of P450-BM3 (Whitehouse et al., 2012). In the early stage, when Arnold’s group looked into alkene epoxidation (Farinas et al., 2004), they discovered that the mutant of the P450-BM3 not only obtained the epoxide product but could also catalyze allylic hydroxylation of cyclohexene (Figure 2G). As early as 1993, Montellano had discovered that when the wild-type P450-BM3 was used, oxidation of ω-unsaturated fatty acids preferentially occurred at its allylic position.
Therefore, in addition to cyclic alkenes, Arnold also tried to react linear alkenes with this mutant and found that only allyl hydroxylated products were produced (Shirane et al., 1993). In view of this, there are more instances of P450-BM3 and variants catalyzed oxidation of the allylic site of natural product to acquire beneficial hydroxylated building blocks of pharmaceuticals and intermediates. Limonene (27) being the cheap and promptly accessible terpene, Pleiss and co-workers used variants of P450-BM3 to convert (4R)-limonene to perillyl alcohol (27a) which might slow down the process of tumor development in the pancreatic, breast, colon, and liver (Seifert et al., 2011). The anti-tumor substance can be obtained by the selective C-7 hydroxylation of limonene (27). However, owing to the substrate that could react with many active positions, perillyl alcohol (27a) was not detected when using the wild-type P450-BM3 (Seifert et al., 2009). After iterative rounds of molecular modeling (Seifert et al., 2011), mutagenesis, and screening, a triple mutant (A264V/A328V/L437F) with high regioselectivity of 97% for the purpose of conversion of limonene (27) to perillyl alcohol (27a) was identified (Figure 2G). A few years later, Reetz and his colleague started mutating from the site near the active center of P450-BM3 and combined with the method of iterative saturation mutation to provide a high degree of regio- and enantio-selectivity. In this work, they reported the first example of P450-BM3 and variants acting as catalysts for the selective complementary synthesis hydroxylation of templates and other similar substrates. For cyclohexene-L-carboxylic acid methyl ester (28), they accomplished the goal of obtaining the mutant (F87L/A328V) with R selectivity of 98% and the mutant (A328S) with S selectivity of 97%, moreover, both mutants can achieve >95% substrate conversion under specific reaction condition. Next, they obtained mutants by screening from the established combinatorial library (Agudo et al., 2012), some of these P450 mutants could also be used to stereoselectively hydrolyze a structurally similar cyclopentene (29) and cyclohexadiene (30) molecule with 84%–94% ee (Figure 2G). Except for cyclic olefins, the Pietruszka group identified the mutant (A74G/L188Q) of P450-BM3 as a highly selective biocatalyst for the C–H oxidation of linear terminal olefins (31) with regio- (90%) and stereo-selectivity (93%–99% ee) by utilizing a comparative method based on the directed evolution (Neufeld et al., 2014). Around the same time, with the introduction of some new technologies, Cheruzel and co-workers reported an efficient combination of light-driven and P450-BM3 enzymes, this method could cause selective oxidation of 10-undecenoic acid (32) with R enantiomer in 85% ee. In addition (Figure 2H), there were no products formed if there was no light source or reductive quencher involved (Kato et al., 2014).
With the continuing exploration of P450-BM3, Urlacher and co-workers demonstrated the sufficient selective oxidation of the tobacco cembranoid, β-cembrenediol which can inhibit tumor promotion by the use of tetradecanoylphorbol acetate by inhibiting the early antigen of the Epstein–Barr virus. The compound was a 14-membered macrocycle (33), which contains seven potential sites for allylic hydroxylation along with three epoxidation sites. However, the Urlacher group still obtained a few P450-BM3 mutants (Figure 2I) that allowed for the selective hydroxylation of the adjoining position C-9 with 100% regioselectivity and 78% diastereoselectivity for the mutant (F87A/I263L) and C-10 with 97% regioselectivity and 48% diastereoselectivity for the mutant (L75A/V78A/F87G) (Le-Huu et al., 2015).
In recent years, examples of biocatalysis for the total synthesis of drugs have gradually increased. Stoltz and coworkers made use of the engineered P450-BM3 enzyme for the total synthesis of nigelladine A, which is the natural product that belongs to the diterpenoid alkaloids. The construction of the ketone carbonyl (34) at the C-7 position is a challenging step because the hydroxylation of the 2o carbon at the C-7 position is accompanied by the competitive process of the 3o carbon at the C-10 position. The optimal mutant 8C7 was obtained by directed evolution of P450-BM3, with residues 75 and 181 being to alanine (Figure 2J). Finally, an ideal product in a 2.8:1 ratio at the desired position was obtained (Loskot et al., 2017). Aitao Li and coworkers designed a two-step chemoenzymatic strategy to generate the steroid drug Trenbolone (35a) (Peng et al., 2022). In this study, the P450-BM3 mutant LG23/T438S was used as a key enzyme to catalyze estra-4,9-diene-3,17-dione (35) C-11 hydroxylation with 94% selectivity and >95% conversion. It should be noted that this was the only bacterial C-11 selective P450 for the model substrate (Figure 2J), others were mainly reported using filamentous fungi with unsatisfactory selectivity and activity (Carballeira et al., 2009).
Those cases show the strong specificity of the P450-BM3. Other types of P450s have also contributed to allylic hydroxylation of various substrates in recent decades. In 2011, Bernhardt reported the prokaryotic cytochrome P450 CYP106A2 from Bacillus megaterium ATCC 13368 which was discovered to be the first bacterial P450 diterpene hydroxylase capable of regioselectively allylic hydroxylation of abietic acid (36) (Figure 2K) in a single step (Bleif et al., 2011). Afterward, to address the insufficient supply of cofactors, P450 CYP7B1 was used by Song in a bioelectrocatalytic 7α-hydroxylation of dehydroepiandosterone (DHEA, 37). To regenerate cofactors, Zhang et al. (2019) used an electricity-driven NADPH regeneration with a concomitant electron shuttle. By using this system, 289 ± 8 mg·L−1 of 7α-hydroxy-DHEA (37a) was produced (Figure 2L). In addition to the steroid compound, Urlacher and coworkers exploited the use of acyclic terpenoids as a substrate and found there was a sort of CYP154E1 from Thermobifida fusca YX that enables this kind of hydroxylation. They discovered that (Bogazkaya et al., 2014) the wild-type CYP154E1 could catalyze the selective oxidation of natural products geraniol (38) and nerol (39). When the wild-type strain reacted with geraniol (38), it was more prone to allylic hydroxylation to generate a single product of 8-hydroxygeraniol, and the reaction conversion was as high as 98%. However, when the wild-type CYP154E1 was used for nerol (39), the yield of conversion was slightly lower. In addition to 8-hydroxygeraniol (39a), 2,3-epoxynerol (39b) was also produced (Figure 2M). The importance of the hydroxylated norisoprenoids has been mentioned in the previous description of the oxyfunctionalization of UPOs. Bernhardt used these types of compounds as substrates for the exploration of the oxidative activity of myxobacterial CYP260B1 and CYP267B1 from Sorangium cellulosum Soce56. The study found the regioselective hydroxylation at the allylic position by CYP260B1 toward a single product is lower compared to that by CYP267B1, as CYP267B1 showed only hydroxylation activity for all tested substrates, while CYP260B1 could also catalyze epoxidation reactions to form byproducts (Litzenburger and Bernhardt., 2016).
Based on the metabolism studies of capsaicin (40) by Tian et al. (2019) found the P450 enzyme CYP105D7 from Streptomyces avermitilis could be a catalyst for the oxidation of the capsaicin (40), which is a pain reliever and also has activities of anti-tumor, anti-obesity, and cardio-protection. In this reaction (Figure 2N), three products were generated, with the majority being obtained by ω-1 allylic hydroxylation at a yield of 64% (40b), the alkyl dehydrogenation product at a yield of 23% (40c), and the epoxidation product at a yield of 13% (40a) (Ma et al., 2021).
Conclusion and outlook
As the demand for oxygenated allylic groups in food, fragrance, cosmetics, pharmaceutical, and materials has grown, so has their significance. Several strategies for the oxidation of allyl to allyl alcohols or allyl esters have been published in recent years. However, compounds often have multiple allylic positions, and the requirement of specificity and chirality is challenging to traditional organic chemistry. Since biocatalysis is emerging to be a viable alternative to traditional chemical catalysis, the use of biocatalytic oxyfunctionalization reactions has increased over the past decade, including whole-cell as the catalyst, UPO enzyme, and P450 enzyme presented in this review. Among the majority of examples presented the whole-cell as the catalyst was often difficult to have high selectivity and yield. The UPO-mediated catalysis was usually accompanied by hydroxylation and epoxidation products, but for this problem, the mutants of UPO have improved chemical selectivity. Being sensitive to hydrogen peroxide and difficult to express in E. coli has limited its wide application in industries. Compared with them, the P450 enzyme is relatively easy to express in E. coli and the mutants generally have better selectivity and activity. Particularly the engineered self-sufficient P450s that have been discussed in this review could catalyze a series of useful reactions, could present promising potential, and broad application prospects. However, the poor stability and the limited scope of substrate usually are the main hinders to their industrial application. With the discovery of new enzymes and the rapid development of directed evolution, substrate engineering, synthetic biology, and immobilization, researchers can continue to address these bottlenecks in the future. In summary, given the advances in enzyme development, biocatalysts can still be considered to have considerable potential for chemo-, regio- and stereo-selectivity oxygenation reactions.
Author contributions
MW wrote the first draft of the manuscript. YC, XZ, and ZW wrote sections of the manuscript. All authors contributed to manuscript revision, read, and approved the submitted version.
Funding
We are grateful for financial support from the National Natural Science Foundation of China (No. 21961048, and 22061049), the Guizhou Science and Technology Department (QKHZC-2019-2759, QKHRC-2016-4029, and QKHJC-ZK2021-036), the Science and Technology Department of Zunyi (ZSKRPT-2020-5, ZSKH-2018-3).
Conflict of interest
The authors declare that the research was conducted in the absence of any commercial or financial relationships that could be construed as a potential conflict of interest.
Publisher’s note
All claims expressed in this article are solely those of the authors and do not necessarily represent those of their affiliated organizations, or those of the publisher, the editors, and the reviewers. Any product that may be evaluated in this article, or claim that may be made by its manufacturer, is not guaranteed or endorsed by the publisher.
References
Adam, W., Peters, K., and PetersStegmann, E. M. V. R. (2000). Hydroxy-directed regio-and diastereoselective [2+2] photocycloaddition (Paternò−Büchi reaction) of benzophenone to chiral allylic alcohols. J. Am. Chem. Soc. 122, 2958–2959. doi:10.1021/ja994279z
Agudo, R., Roiban, G. D., and Reetz, M. T. (2012). Achieving regio- and enantioselectivity of P450-catalyzed oxidative C−H activation of small functionalized molecules by structure-guided directed evolution. Chembiochem 13, 1465–1473. doi:10.1002/cbic.201200244
Aranda, C., Municoy, M., Guallar, V., Kiebist, J., Scheibner, K., Ullrich, R., et al. (2019). Selective synthesis of 4-hydroxyisophorone and 4-ketoisophorone by fungal peroxygenases. Catal. Sci. Technol. 9, 1398–1405. doi:10.1039/c8cy02114g
Babot, E. D., Aranda, C., Del Ri, O. J., Ullrich, R., Kiebist, J., Scheibner, K., et al. (2020). Selective oxygenation of ionones and damascones by fungal peroxygenases. J. Agric. Food Chem. 68, 5375–5383. doi:10.1021/acs.jafc.0c01019
Baeshen, M. N., Al-Hejin, A. M., Bora, R. S., Ahmed, M. M., Ramadan, H. A., Saini, K. S., et al. (2015). Production of biopharmaceuticals in E. coli: Current scenario and future perspectives. J. Microbiol. Biotechnol. 25, 953–962. doi:10.4014/jmb.1412.12079
Balcells, D., Clot, E., and Eisenstein, O. (2010). C−H bond activation in transition metal species from a computational perspective. Chem. Rev. 110, 749–823. doi:10.1021/cr900315k
Berthier, A., Lemaire-Ewing, S., Prunet, C., Monier, S., Athias, A., Bessede, G., et al. (2004). Involvement of a calcium-dependent dephosphorylation of BAD associated with the localization of Trpc-1 within lipid rafts in 7-ketocholesterol-induced THP-1 cell apoptosis. Cell Death Differ. 11, 897–905. doi:10.1038/sj.cdd.4401434
Blank, L. M., Ebert, B. E., BuehlerBühler, K. B., and Buhler, B. (2010). Redox biocatalysis and metabolism: molecular mechanisms and metabolic network analysis. Antioxidants redox Signal. 13, 349–394. doi:10.1089/ars.2009.2931
Bleif, S., Hannemann, F., Lisurek, M., von Kries, J. P., Zapp, J., Dietzen, M., et al. (2011). Identification of CYP106A2 as a regioselective allylic bacterial diterpene hydroxylase. Chembiochem 12, 576–582. doi:10.1002/cbic.201000404
Bogazkaya, A. M., von Buhler, C. J., Kriening, S., Busch, A., Seifert, A., Pleiss, J., et al. (2014). Selective allylic hydroxylation of acyclic terpenoids by CYP154E1 from thermobifida fusca YX. Beilstein J. Org. Chem. 10, 1347–1353. doi:10.3762/bjoc.10.137
Bornscheuer, U. T., Huisman, G., Kazlauskas, R., Lutz, S., and MooreRobins, J. K. (2012). Engineering the third wave of biocatalysis. Nature 485, 185–194. doi:10.1038/nature11117
Carballeira, J., Quezada, M., Hoyos, P., Simeó, Y., Hernaiz, M., Alcantara, A., et al. (2009). Microbial cells as catalysts for stereoselective red–ox reactions. Biotechnol. Adv. 27, 686–714. doi:10.1016/j.biotechadv.2009.05.001
Casarano, R., Matos, J. R., and FantiniPetri, M. C. A. D. F. S. (2005). Composites of allyl glycidyl ether modified polyethylene and cellulose. Polymer 46, 3289–3299. doi:10.1016/j.polymer.2005.02.077
Chen, R. R. (2007). Permeability issues in whole-cell bioprocesses and cellular membrane engineering. Appl. Microbiol. Biotechnol. 74, 730–738. doi:10.1007/s00253-006-0811-x
Church, T. L., and Andersson, P. G. (2008). Iridium catalysts for the asymmetric hydrogenation of olefins with nontraditional functional substituents. Coord. Chem. Rev. 252, 513–531. doi:10.1016/j.ccr.2007.09.015
Dong, J., Fernández‐Fueyo, E., Hollmann, F., Paul, C. E., Pesic, M., Schmidt, S., et al. (2018). Biocatalytic oxidation reactions: a chemist's perspective. Angew. Chem. Int. Ed. 57, 9238–9261. doi:10.1002/anie.201800343
Fanani, M. Z., Sawai, S., Seki, H., Ishimori, M., Ohyama, K., Fukushima, E. O., et al. (2021). Allylic hydroxylation activity is a source of saponin chemodiversity in the genus glycyrrhiza. Plant Cell Physiol. 62, 262–271. doi:10.1093/pcp/pcaa173
Farinas, E. T., Alcalde, M., and Arnold, F. (2004). Alkene epoxidation catalyzed by cytochrome P450 BM-3 139-3. Tetrahedron 60, 525–528. doi:10.1016/j.tet.2003.10.099
Fraatz, M. A., Berger, R. G., and Zorn, H. (2009). Nootkatone--a biotechnological challenge. Appl. Microbiol. Biotechnol. 83, 35–41. doi:10.1007/s00253-009-1968-x
Gargiulo, S., Testa, G., Gamba, P., Staurenghi, E., and PoliLeonarduzzi, G. G. (2017). Oxysterols and 4-hydroxy-2-nonenal contribute to atherosclerotic plaque destabilization. Free Radic. Biol. Med. 111, 140–150. doi:10.1016/j.freeradbiomed.2016.12.037
Grobe, G., Ullrich, R., Pecyna, M. J., Kapturska, D., Friedrich, S., Hofrichter, M., et al. (2011). High-yield production of aromatic peroxygenase by the agaric fungus Marasmius rotula. Amb. Express 1, 31. doi:10.1186/2191-0855-1-31
Gutiérrez, A., Babot, E. D., Ullrich, R., Hofrichter, M., MartínezJosé, A. T. C., and del Rio, J. C. (2011). Regioselective oxygenation of fatty acids, fatty alcohols and other aliphatic compounds by a basidiomycete heme-thiolate peroxidase. Archives Biochem. biophysics 514, 33–43. doi:10.1016/j.abb.2011.08.001
Hammer, S. C., Knight, A. M., and Arnold, F. H. (2017). Design and evolution of enzymes for non-natural chemistry. Curr. Opin. Green Sustain. Chem. 7, 23–30. doi:10.1016/j.cogsc.2017.06.002
Hernández-Toribio, J., Hussain, M. M., Cheng, K., Carroll, P. J., and Walsh, P. J. (2011). Diastereoselective aziridination of 2-B (pin)-substituted allylic alcohols: an efficient approach to novel organoboron compounds. Org. Lett. 13, 6094–6097. doi:10.1021/ol202588g
Hofrichter, M., Kellner, H., and PecynaUllrich, M. J. R. (2015). Fungal unspecific peroxygenases: heme-thiolate proteins that combine peroxidase and cytochrome P450 properties.” in Monooxygenase, peroxidase and peroxygenase properties and mechanisms of cytochrome P450, 341–368. doi:10.1007/978-3-319-16009-2_13
Hofrichter, M., and Ullrich, R. (2006). Heme-thiolate haloperoxidases: Versatile biocatalysts with biotechnological and environmental significance. Appl. Microbiol. Biotechnol. 71, 276–288. doi:10.1007/s00253-006-0417-3
Hofrichter, M., and Ullrich, R. (2014). Oxidations catalyzed by fungal peroxygenases. Curr. Opin. Chem. Biol. 19, 116–125. doi:10.1016/j.cbpa.2014.01.015
Hrycay, E. G., and Bandiera, S. M. (2012). The monooxygenase, peroxidase, and peroxygenase properties of cytochrome P450. Archives Biochem. biophysics 522, 71–89. doi:10.1016/j.abb.2012.01.003
Jensen, K. P., and Ryde, U. (2004). How O2 binds to heme: Reasons for rapid binding and spin inversion. J. Biol. Chem. 279, 14561–14569. doi:10.1074/jbc.M314007200
Jiang, W. Fang B., and Fang, B. (2020). Synthesizing chiral drug intermediates by biocatalysis. Appl. Biochem. Biotechnol. 192, 146–179. doi:10.1007/s12010-020-03272-3
Kato, M., Nguyen, D., Gonzalez, M., Cortez, A., and MullenCheruzel, S. E. L. E. (2014). Regio- and stereoselective hydroxylation of 10-undecenoic acid with a light-driven P450 BM3 biocatalyst yielding a valuable synthon for natural product synthesis. Bioorg. Med. Chem. 22, 5687–5691. doi:10.1016/j.bmc.2014.05.046
Ke, M., Liu, Z., Zhang, K., Zuo, S., and Chen, F. (2021). Synergistic Pd/Cu catalysis for stereoselective allylation of vinylethylene carbonates with glycine iminoesters: Enantioselective access to diverse trisubstituted allylic amino acid derivatives. Green Synthesis Catal. 2, 228–232. doi:10.1016/j.gresc.2021.04.004
Kiebist, J., Schmidtke, K. U., Zimmermann, J., Kellner, H., Jehmlich, N., Ullrich, R., et al. (2017). A peroxygenase from chaetomium globosum catalyzes the selective oxygenation of testosterone. Chembiochem 18, 563–569. doi:10.1002/cbic.201600677
Kim, Y., and Li, C. J. (2020). Perspectives on green synthesis and catalysis. Green Synthesis Catal. 1, 1–11. doi:10.1016/j.gresc.2020.06.002
Kluge, M., Ullrich, R., and ScheibnerHofrichter, K. M. (2012). Stereoselective benzylic hydroxylation of alkylbenzenes and epoxidation of styrene derivatives catalyzed by the peroxygenase of Agrocybe aegerita. Green Chem. 14, 440–446. doi:10.1039/c1gc16173c
Knorrscheidt, A., Soler, J., Hünecke, N., Püllmann, P., and Garcia-BorràsWeissenborn, M. M. J. (2021). Simultaneous screening of multiple substrates with an unspecific peroxygenase enabled modified alkane and alkene oxyfunctionalisations. Catal. Sci. Technol. 11, 6058–6064. doi:10.1039/d0cy02457k
König, L., Hartz, P., and BernhardtHannemann, R. F. (2019). High-yield C11-oxidation of hydrocortisone by establishment of an efficient whole-cell system in Bacillus megaterium. Metab. Eng. 55, 59–67. doi:10.1016/j.ymben.2019.06.005
Krugener, S., Krings, U., and ZornBerger, H. R. G. (2010). A dioxygenase of Pleurotus sapidus transforms (+)-valencene regio-specifically to (+)-nootkatone via a stereo-specific allylic hydroperoxidation. Bioresour. Technol. 101, 457–462. doi:10.1016/j.biortech.2009.08.087
Le-Huu, P., Heidt, T., Claasen, B., and LaschatUrlacher, S. V. B. (2015). Chemo-regio-and stereoselective oxidation of the monocyclic diterpenoid β-cembrenediol by P450 BM3. ACS Catal. 5, 1772–1780. doi:10.1021/cs5020404
Li, B. B, B. B., Zhang, J., Chen, F. F., Chen, Q., Xu, J. H., Zheng, G. W., et al. (2021). Direct reductive amination of ketones with amines by reductive aminases. Green Synthesis Catal. 2, 345–349. doi:10.1016/j.gresc.2021.08.005
Li, D, D., Chen, X., Chen, Z., Lin, X., Xu, J., Wu, Q., et al. (2021). Directed evolution of lipase a from Bacillus subtilis for the preparation of enantiocomplementary sec-alcohols. Green Synthesis Catal. 2, 290–294. doi:10.1016/j.gresc.2021.07.003
Linde, D., Olmedo, A., Gonzalez-Benjumea, A., Estevez, M., Renau-Minguez, C., Carro, J., et al. (2020). Two new unspecific peroxygenases from heterologous expression of fungal genes in Escherichia coli. Appl. Environ. Microbiol. 86, e02899-19. doi:10.1128/AEM.02899-19
Litzenburger, M., and Bernhardt, R. (2016). Selective oxidation of carotenoid-derived aroma compounds by CYP260B1 and CYP267B1 from Sorangium cellulosum So ce56. Appl. Microbiol. Biotechnol. 100, 4447–4457. doi:10.1007/s00253-015-7269-7
Loskot, S. A., Romney, D. K., and ArnoldStoltz, F. H. B. M. (2017). Enantioselective total synthesis of nigelladine a via late-stage C–H oxidation enabled by an engineered P450 enzyme. J. Am. Chem. Soc. 139, 10196–10199. doi:10.1021/jacs.7b05196
Lumbroso, A., Cooke, M. L., and Breit, B. (2013). Catalytic asymmetric synthesis of allylic alcohols and derivatives and their applications in organic synthesis. Angew. Chem. Int. Ed. 52, 1890–1932. doi:10.1002/anie.201204579
Ma, B., Wang, Q., Han, B. N., Ikeda, H., and ZhangXu, C. L. H. (2021). Hydroxylation, epoxidation, and dehydrogenation of capsaicin by a microbial promiscuous cytochrome P450 105D7. Chem. Biodivers. 18, e2000910. doi:10.1002/cbdv.202000910
Miura, Y., and Fulco, A. J. (1974). (ω–2) Hydroxylation of fatty acids by a soluble system from Bacillus megaterium. J. Biol. Chem. 249, 1880–1888. doi:10.1016/s0021-9258(19)42868-8
Molina-Espeja, P., Garcia-Ruiz, E., Gonzalez-Perez, D., Ullrich, R., and HofrichterAlcalde, M. M. (2014). Directed evolution of unspecific peroxygenase from Agrocybe aegerita. Appl. Environ. Microbiol. 80, 3496–3507. doi:10.1128/AEM.00490-14
Molina-Espeja, P., Santos-Moriano, P., Garcia-Ruiz, E., Ballesteros, A., and PlouAlcalde, F. J. M. (2019). Structure-guided immobilization of an evolved unspecific peroxygenase. Int. J. Mol. Sci. 20, 1627. doi:10.3390/ijms20071627
Müller, P., and Fruit, C. (2003). Enantioselective catalytic aziridinations and asymmetric nitrene insertions into CH bonds. Chem. Rev. 103, 2905–2920. doi:10.1021/cr020043t
Munro, A. W., Girvan, H. M., Mason, A. E., and DunfordMcLean, A. J. K. J. (2013). What makes a P450 tick? Trends Biochem. Sci. 38, 140–150. doi:10.1016/j.tibs.2012.11.006
Nakamura, A., and Nakada, M. (2013). Allylic oxidations in natural product synthesis. Synthesis 45, 1421–1451. doi:10.1055/s-0033-1338426
Nelson, D. R. (2018). Cytochrome P450 diversity in the tree of life. Biochimica Biophysica Acta - Proteins Proteomics 1866, 141–154. doi:10.1016/j.bbapap.2017.05.003
Neufeld, K., Henssen, B., and Pietruszka, J. (2014). Enantioselective allylic hydroxylation of omega-alkenoic acids and esters by P450 BM3 monooxygenase. Angew. Chem. Int. Ed. 53, 13253–13257. doi:10.1002/anie.201403537
Okabe, A., Urano, Y., Itoh, S., Suda, N., Kotani, R., Nishimura, Y., et al. (2014). Adaptive responses induced by 24S-hydroxycholesterol through liver X receptor pathway reduce 7-ketocholesterol-caused neuronal cell death. Redox Biol. 2, 28–35. doi:10.1016/j.redox.2013.11.007
Onken, J., and Berger, R. (1999). Effects of R-(+)-limonene on submerged cultures of the terpene transforming basidiomycete Pleurotus sapidus. J. Biotechnol. 69, 163–168. doi:10.1016/S0168-1656(99)00040-1
Pellissier, H. (2008). Recent developments in asymmetric cyclopropanation. Tetrahedron 64, 7041–7095. doi:10.1016/j.tet.2008.04.079
Peng, Y., Gao, C., Zhang, Z., Wu, S., and ZhaoLi, J. A. (2022). A chemoenzymatic strategy for the synthesis of steroid drugs enabled by P450 monooxygenase-mediated steroidal core Modification. ACS Catal. 12, 2907–2914. doi:10.1021/acscatal.1c05776
Peter, S., Kinne, M., Ullrich, R., and KayserHofrichter, G. M. (2013). Epoxidation of linear, branched and cyclic alkenes catalyzed by unspecific peroxygenase. Enzyme Microb. Technol. 52, 370–376. doi:10.1016/j.enzmictec.2013.02.013
Peter, S., Kinne, M., Wang, X., Ullrich, R., Kayser, G., Groves, J. T., et al. (2011). Selective hydroxylation of alkanes by an extracellular fungal peroxygenase. FEBS J. 278, 3667–3675. doi:10.1111/j.1742-4658.2011.08285.x
Piontek, K., Ullrich, R., Liers, C., Diederichs, K., and PlattnerHofrichter, D. A. M. (2010). Crystallization of a 45 kDa peroxygenase/peroxidase from the mushroom Agrocybe aegerita and structure determination by SAD utilizing only the haem iron. Acta Crystallogr. Sect. F. Struct. Biol. Cryst. Commun. 66, 693–698. doi:10.1107/s1744309110013515
Qu, G., Li, A., Acevedo‐Rocha, C. G., and SunReetz, Z. M. T. (2020). The crucial role of methodology development in directed evolution of selective enzymes. Angew. Chem. Int. Ed. 59, 13204–13231. doi:10.1002/anie.201901491
Raffo, A., D'Aloise, A., Magri, A. D. Leclercq C., and Leclercq, C. (2012). Quantification of allyl hexanoate in pineapple beverages and yogurts as a case study to characterise a source of uncertainty in dietary exposure assessment to flavouring substances. Food Addit. Contam. Part A 29, 43–53. doi:10.1080/19440049.2011.623285
Rickert, A., Krombach, V., Hamers, O., and ZornMaison, H. W. (2012). Enzymatic allylic oxidations with a lyophilisate of the edible fungus Pleurotus sapidus. Green Chem. 14, 639. doi:10.1039/c2gc16317a
Schroepfer, G. J. (1981). Sterol biosynthesis. Annu. Rev. Biochem. 50, 585–621. doi:10.1146/annurev.bi.50.070181.003101
Seifert, A., Antonovici, M., and HauerPleiss, B. J. (2011). An efficient route to selective bio-oxidation catalysts: An iterative approach comprising modeling, diversification, and screening, based on CYP102A1. Chembiochem 12, 1346–1351. doi:10.1002/cbic.201100067
Seifert, A., Vomund, S., Grohmann, K., Kriening, S., Urlacher, V. B., Laschat, S., et al. (2009). Rational design of a minimal and highly enriched CYP102A1 mutant library with improved regio-stereo- and chemoselectivity. Chembiochem 10, 853–861. doi:10.1002/cbic.200800799
Sharpless, K. B., and Michaelson, R. (1973). High stereo- and regioselectivities in the transition metal catalyzed epoxidations of olefinic alcohols by tert-butyl hydroperoxide. J. Am. Chem. Soc. 95, 6136–6137. doi:10.1021/ja00799a061
Shirane, N., Sui, Z., PetersonOrtiz de Montellano, J. A. P. R., and Ortiz de Montellano, P. R. (1993). Cytochrome P450bm-3 (CYP102): regiospecificity of oxidation of ω-unsaturated fatty acids and mechanism-based inactivation. Biochemistry 32, 13732–13741. doi:10.1021/bi00212a044
Sono, M., Roach, M. P., and CoulterDawson, E. D. J. H. (1996). Heme-containing oxygenases. Chem. Rev. 96, 2841–2888. doi:10.1021/cr9500500
Tian, K., Zhu, J., and LiQiu, M. X. (2019). Capsaicin is efficiently transformed by multiple cytochrome P450s from Capsicum fruit-feeding Helicoverpa armigera. Pesticide Biochem. Physiology 156, 145–151. doi:10.1016/j.pestbp.2019.02.015
Ullrich, R., and Hofrichter, M. (2007). Enzymatic hydroxylation of aromatic compounds. Cell. Mol. Life Sci. 64, 271–293. doi:10.1007/s00018-007-6362-1
Ullrich, R., and Hofrichter, M. (2005). The haloperoxidase of the agaric fungus Agrocybe aegerita hydroxylates toluene and naphthalene. FEBS Lett. 579, 6247–6250. doi:10.1016/j.febslet.2005.10.014
Ullrich, R., Nüske, J., Scheibner, K., Spantzel, J., and Hofrichter, M. (2004). Novel haloperoxidase from the agaric basidiomycete Agrocybe aegerita oxidizes aryl alcohols and aldehydes. Appl. Environ. Microbiol. 70, 4575–4581. doi:10.1128/aem.70.8.4575-4581.2004
Urlacher, V. B., and Girhard, M. (2019). Cytochrome P450 monooxygenases in biotechnology and synthetic biology. Trends Biotechnol. 37, 882–897. doi:10.1016/j.tibtech.2019.01.001
Venancio, A. N., Menini, L., Maronde, D. N., and GusevskayaParreira, E. V. L. A. (2021). Palladium catalyzed oxidation of biorenewable β-citronellol and geraniol for the synthesis of polyfunctionalized fragrances. Mol. Catal. 504, 111449. doi:10.1016/j.mcat.2021.111449
Wang, W., Wang, W., Ge, H., Li, G., Shen, P., Xu, S., et al. (2020). Biocatalytic allylic hydroxylation of unsaturated triterpenes and steroids by Bacillus megaterium CGMCC 1.1741. Bioorg. Chem. 99, 103826. doi:10.1016/j.bioorg.2020.103826
Wang, X. (2013). A novel heme-thiolate peroxygenase AaeAPO and its implications for carbon-hydrogen bond activation chemistry. New Jersey: Princeton University Press.
Wang, X. (2016). Cloning and expression of AaeAPO from Agrocybe aegerita to E. coli, for studies of structure-function relationships by site-specific mutagenesis. Switzerland: Springer International Publishing, 113–130.
Wang, Y., Lan, D., and DurraniHollmann, R. F. (2017). Peroxygenases en route to becoming dream catalysts. what are the opportunities and challenges? Curr. Opin. Chem. Biol. 37, 1–9. doi:10.1016/j.cbpa.2016.10.007
Weidmann, V., Kliewer, S., Sick, M., Bycinskij, S., Kleczka, M., Rehbein, J., et al. (2015). Studies towards the synthetic applicability of biocatalytic allylic oxidations with the lyophilisate of Pleurotus sapidus. J. Mol. Catal. B Enzym. 121, 15–21. doi:10.1016/j.molcatb.2015.07.008
Whitehouse, C. J., Bell, S. G., and Wong, L. L. (2012). P450 BM3 (CYP102A1): Connecting the dots. Chem. Soc. Rev. 41, 1218–1260. doi:10.1039/c1cs15192d
Xue, X. S., Ji, P., and ZhouCheng, B. J. P. (2017). The essential role of bond energetics in C−H activation/functionalization. Chem. Rev. 117, 8622–8648. doi:10.1021/acs.chemrev.6b00664
Zhang, C., Xu, S. H., Ma, B. L., Wang, W. W., and YuZhang, B. Y. J. (2017). New derivatives of ursolic acid through the biotransformation by Bacillus megaterium CGMCC 1.1741 as inhibitors on nitric oxide production. Bioorg. Med. Chem. Lett. 27, 2575–2578. doi:10.1016/j.bmcl.2017.03.076
Zhang, Z., Li, F., Cao, Y., Tian, Y., Li, J., Zong, Y., et al. (2019). Electricity-driven 7α-hydroxylation of a steroid catalyzed by a cytochrome P450 monooxygenase in engineered yeast. Catal. Sci. Technol. 9, 4877–4887. doi:10.1039/c9cy01288e
Keywords: biocatalysis, allylic oxidation, C–H activation, oxyfunctionalization, UPO, P450
Citation: Wang M, Zhou X, Wang Z and Chen Y (2022) Enzyme-catalyzed allylic oxidation reactions: A mini-review. Front. Chem. 10:950149. doi: 10.3389/fchem.2022.950149
Received: 22 May 2022; Accepted: 04 July 2022;
Published: 15 August 2022.
Edited by:
Francisco Solano, University of Murcia, SpainReviewed by:
Sebastian Cosgrove, Keele University, United KingdomCopyright © 2022 Wang, Zhou, Wang and Chen. This is an open-access article distributed under the terms of the Creative Commons Attribution License (CC BY). The use, distribution or reproduction in other forums is permitted, provided the original author(s) and the copyright owner(s) are credited and that the original publication in this journal is cited, in accordance with accepted academic practice. No use, distribution or reproduction is permitted which does not comply with these terms.
*Correspondence: Yongzheng Chen, eXpjaGVuQHptdS5lZHUuY24=