- 1Institute of Drug Discovery Technology, Ningbo University, Ningbo, China
- 2Qian Xuesen Collaborative Research Center of Astrochemistry and Space Life Sciences, Ningbo University, Ningbo, China
- 3Department of Chemical Biology, College of Chemistry and Chemical Engineering, Xiamen University, Xiamen, China
Life’s origins have always been a scientific puzzle. Understanding the production of biomolecules is crucial for understanding the evolution of life on Earth. Numerous studies on trimetaphosphate have been conducted in the field of prebiotic chemistry. However, its role in prebiotic chemistry has been documented infrequently in the review literature. The goal of this thesis is to review the role of trimetaphosphate in the early Earth’s biomolecule synthesis and phosphorylation. Additionally, various trimetaphosphate-mediated reaction pathways are discussed, as well as the role of trimetaphosphate in prebiotic chemistry. Finally, in our opinion, interactions between biomolecules should be considered in prebiotic synthesis scenarios since this may result in some advances in subsequent research on this subject. The research establishes an essential and opportune foundation for an in-depth examination of the “mystery of life".
Introduction
The question “how did life emerge on the early earth” has perplexed scientists for decades. Scientists have recently made a concerted attempt to accomplish this. Charles Darwin said, “Phosphorus played an irreversible role in the creation of life, and phosphorus is the main source of life on Earth” (Zimmer, 2009). F H Westheimer pointed out that for genetic material like DNA to exist, a connecting link that is at least divalent must be present as well. The connecting unit should have a third, ionizable group to ensure that the final substance retains its membrane charge. Thus, phosphorus is an irreplaceable role (Westheimer, 1987). It can be said that phosphorus plays an absolutely important role in all life forms, and this chemical is widely involved in metabolism and biochemical reactions (Karki et al., 2017).
However, the availability of phosphates in prebiotic chemistry has been questioned. According to early geological research, most of the phosphorus on the primitive earth was insoluble in water and existed in the form of apatite (Gulick, 1957; Keefe and Miller, 1995; Macia et al., 1997; Ulian et al., 2021). As a result, a common perception is that phosphate was strongly limited in prebiotic chemical development, and thus no such indispensable element in prebiotic processes, unless it is derived from meteorites, lightning and sources (de Graaf et al., 1995; Ritson et al., 2020). Burcar, Bradley, et al. proposed that the formation of a urea/ammonium formate/water (UAFW) eutectic solution could promote phosphorylation, which results in increased sources of phosphate with different solubilities (Burcar et al., 2016). Polyphosphates, in contrast to orthophosphates, are long-chain molecules generated when orthophosphates are joined together via stable P-O-P bonds during dehydration (Brown and Kornberg, 2004). Polyphosphates have a higher phosphorylation capability. Thus, polyphosphates are required for bioactivation and phosphorylation, and they are also more active and soluble inherently than orthophosphates (Omelon et al., 2009; Dürr-Mayer et al., 2021). Numerous researchers have attempted to convert orthophosphates to polyphosphates in water using a variety of condensing agents (Weber, 1981, 1982; Hagan et al., 2007). Griffith et al. discovered that iron (III) phosphate may be reduced to iron (II) phosphate and carbon dioxide in the presence of carbon monoxide and that iron (III) phosphate can form pyrophosphate in the presence of hydrogen sulfide. At somewhat increased temperatures, pyrophosphate can then spontaneously polymerize into linear and cyclic polyphosphates (Griffith et al., 1977). Under prebiotic conditions, this cascade of processes was feasible. Yamagata et al. conducted experiments replicating the conditions of volcanic magma and studied volatile condensates in volcanic gases. Additionally, studies revealed that volcanic activity can result in the formation of water-soluble polyphosphates, such as trimetaphosphate (P3m) (Yamagata et al., 1991). Pasek et al. also proposed that the P3m could be generated among other polyphosphates (Pasek et al., 2008), Gibard et al. demonstrated that diamidophosphate could form through the reaction of NH4+ with the meteorite mineral schreibersite. Thus the N-P compounds could generate P3m (Gibard et al., 2018). Then after, Gibard et al. also showed that diamidophosphate could form through the reaction of NH4+ with the meteorite mineral schreibersite. Thus the N-P compounds could generate P3m (Gibard et al., 2019). Additionally, Britvin et al. reported the cyclic tetraphosphate from mineral. (Britvin et al., 2021). The researchers identified pyrophosphate and triphosphate in the volcanic jet condensate from Wusu Mountain (Schwartz, 2006). All of the above evidences infer the existence of polyphosphates before the existence of living matter, and promotes the occurrence of chemical reactions and the evolution of life. Furthermore, numerous investigations into the phosphorylation and polymerization of biomolecules and polyphosphates have been conducted in this area (Ponnamperuma et al., 1963b; Schwartz and Fox, 1964; Ponnamperuma and Mack, 1965; Ferris, 1968; Fox and Waehneldt, 1968; Lohrmann and Orgel, 1968; Rabinowitz et al., 1968; Schwartz and Ponnamperuma, 1968; Rabinowitz et al., 1969; Rabinowitz, 1970; Lohrmann and Orgel, 1971; Handschuh et al., 1973; Lohrmann, 1975; Hulshof and Ponnamperuma, 1976; Schoffstall, 1976; Yamagata et al., 1979; Yamanaka et al., 1988; Schwartz, 2006; Holm and Baltscheffsky, 2011; Kee and Monnard, 2017; Christ et al., 2020; Serov et al., 2020; Brunk and Marshall, 2021; Glonek, 2021; Ying et al., 2021).
The purpose of this paper is to review the prebiotic synthesis and phosphorylation of several biomolecules with the participation of P3m, such as peptides, nucleotides, oligonucleotides etc. These prebiotic investigations of biomolecules not only have essential guiding value for the development of the life sciences, but also underscore the important role of P3m in the evolution of prebiotic chemistry.
Prebiotic Peptide Synthesis Involved by P3M
The synthesis of prebiotic peptides that occurred under early Earth conditions has long been the focus of the field of the origin of life (Urey, 1952; Leach et al., 2006; Fiore, 2019; Micca Longo et al., 2021). Due to the fact that the synthesis of peptides from amino acids requires energy input and the removal of water molecules (Figure 1), the reaction is often not favored in aqueous solutions, which is also thermodynamically unfavorable for the reaction to occur. As a result, early research on prebiotic peptides was conducted in dry settings (Hayakawa et al., 1967; Yanagawa et al., 1990). Wet-dry cycling is viewed as a mechanism for driving the condensation reaction that generates biopolymers, and numerous publications have described the synthesis of prebiotic peptides under dry-wet cycling circumstances (Figure 2) (Forsythe et al., 2015; Campbell et al., 2019). Additionally, the majority of the early primitive earth environments were aqueous, and adding certain activators to the aqueous environment or altering the reaction temperature, pH, and other conditions in order to make the reaction thermodynamically favorable may be consistent with the emergence of prebiotic peptides. Numerous indications for the condensate’s prebiotic chemistry contribution. For example, urea can help phosphorylate nucleosides and glycerol (Knecht et al., 2002). Certain minerals and clays also have the potential to catalyze the process of prebiotic source phosphorylation, and many minerals are positively charged and can adsorb negatively charged phosphates and phosphate esters, providing a relatively ideal polymerization environment for phosphorus compounds. A study conducted by Gull et al. (2014) demonstrated that inorganic phosphates adsorbed on silica can be condensed at relatively low temperatures (Gull et al., 2014).
P3m appears to stimulate the synthesis of prebiotic-derived peptides under a range of circumstances (Rabinowitz, 1970; Yamanaka et al., 1988; Yamagata and Inomata, 1997; A and E, 2002; Gu et al., 2011; Sibilska et al., 2017; Sibilska et al., 2018; Ying et al., 2018a; Ying et al., 2018b). Numerous variables influence the yield of prebiotic dipeptides, including temperature, pH, reaction system, wet and dry environment, and so on. Although we do not know precisely how prebiotic sources evolved on early Earth, we can alter these reaction conditions and hence examine putative evolutionary pathways for life.
Rabinowitz et al. initially described the condensation reaction of glycine (Gly) and P3m in 1961. Following that, Chung et al. developed a reaction mechanism for the synthesis of Gly2 in an alkaline solution using Gly and P3m (Chung et al., 1971). The amino group of Gly likely attacks P3m first, forming the open-chain molecule Gly-N-triphosphate. Following intramolecular condensation, a cyclic acylphosphoramidate is formed as an active intermediate (CAPA). CAPA is then attacked by another Gly to produce Gly2-N-phosphate, which is quickly hydrolyzed to generate Gly2 (Figure 3). Yamanaka et al. added phosphate and Gly in equimolar quantities to an aqueous solution with a pH of 4.0–9.0 and a temperature of 38°C. The reaction solution was examined using high-performance liquid chromatography and the ninhydrin reaction method to detect the Gly tetramer and hexamer. They discovered that the yield was greatest at a pH of about 7. Additionally, yields varied with different phosphates, and the reaction with P3m was approximately tenfold that of tetrametaphosphate under the identical circumstances (Yamanaka et al., 1988). This emphasizes the critical role of P3m in the evolution of life. Yamanaka and colleagues postulated that the condensation of amino acids with P3m may occur under slightly acidic settings; subsequent analysis confirmed that the condensation of oligoglycines with P3m occurs most efficiently under neutral or weakly acidic conditions. Condensation occurs differently in aqueous solution than it does with Gly. Gly2-N-triphosphate is rarely formed in neutral or acidic circumstances (Yamanaka et al., 1988).
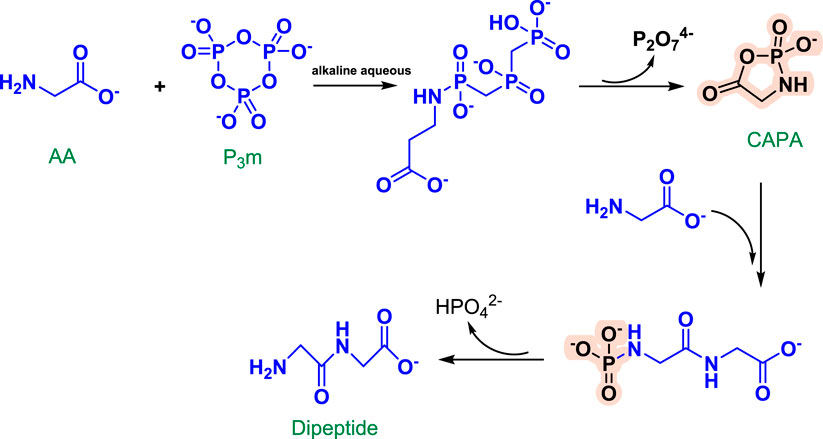
FIGURE 3. Reaction mechanism of Gly2 formation from P3m in alkaline solution [redrawn from (Chung et al., 1971)].
Along with synthesizing peptides in alkaline and acidic conditions, Izabela Sibilska et al. did comparative tests on glycine and alanine in the presence or absence of P3m at temperatures ranging from 0 to 100°C and pH values ranging from 1 to 12. The data indicate that glycine and alanine rapidly produce peptides in the absence of harsh near-boiling temperatures, excessive pH values, or dry solid residues at P3m. In comparison, peptides are synthesized in the presence of P3m under a broader range of circumstances, including ambient temperature, neutral pH, and water (Sibilska et al., 2018). The above indicates that the synthesis of prebiotic peptides is promoted by P3m in acidic, alkaline, or neutral aqueous solutions.
Additionally, similar to some of the conditions outlined previously, P3m can facilitate or accelerate the production of prebiotic-derived peptides. We have discovered that P3m could also act as a catalyst for the synthesis of cyclic dipeptides in an aqueous solution (Ying et al., 2018b; Guo et al., 2021). In addition to the parameters outlined above, there may be a broader range of circumstances for the synthesis of prebiotic peptides in the primordial Earth environment, but we are unable to recreate the prebiotic period’s environment to undertake relevant investigations. This is a perplexing subject in the field of life origins. Through continual investigation, it is possible for us to identify some chemical beginnings of life and to reproduce as many prebiotic-era processes as possible.
Nucleoside Prebiotic Phosphorylation Mediated by P3M
Nucleotides are the fundamental building units of RNA and DNA, the two polymers responsible for life’s biochemistry (Azzam and Liu, 2013; Shivakumar et al., 2019; Miggiano et al., 2020; Cai et al., 2021). The investigation of their synthesis has made a substantial contribution to our understanding of chemical evolution and the chemical origins of life. Thus, from the perspective of the origin of life, how did nucleotides assemble from their various chemical components (ribose, adenine, guanine, cytosine, and uracil) or their respective constituent small molecules (e.g., HCN, HCHO, and phosphate)?
Under replicated pristine Earth circumstances, the synthesis of the bases adenine and guanine, as well as the sugars ribose and deoxyribose, has been demonstrated (Oro, 1963). Monosaccharides have been synthesized by Gabel and Ponnamperuma in 1967 by formaldehyde; purines and pyrimidines have also been shown to be formed by prebiotic reactions by Oro and Kimball and Ponnamperuma et al. (Ponnamperuma and Mack, 1965; Gabel and Ponnamperuma, 1967). Additionally, the nucleobases in meteorites was also reported by Burton et al. (Burton et al., 2012). The process of nucleotide synthesis involves the removal of water molecules: Purines or pyrimidines first react with ribose or deoxyribose to generate nucleosides, which are then phosphorylated to form nucleotides. When several nucleotides are polymerized, an ester link is created between the nucleotide’s phosphate residue and the pentose residue’s hydroxyl group (Mizuno et al., 1975). It is worth mentioning that the preceding series of chemical evolutionary processes result in the formation of water molecules, making such reactions thermodynamically unfavorable in water. Thus, in what kind of environment did such chemical evolution occur during the early Earth’s history? Additionally, the supply of phosphorus during chemical evolution is a point of discussion.
Phosphorus is required for the formation of prebiotic sources of biological macromolecules like RNA and proteins and phosphorylation of nucleosides is a critical link in RNA synthesis (Lin et al., 2011; Pasek et al., 2013; Altwegg et al., 2016; Martínez-Bachs and Rimola, 2019; Toparlak and Mansy, 2019; Liu et al., 2021). The first reagent used for phosphorylation of nucleoside prebiotic sources was a mixture of urea and inorganic phosphate. One important example is nucleoside 5′-triphosphate, a precursor of RNA in modern biology. Kim HyoJoong et al. synthesized large amounts (2-3 percent) of nucleoside 5′-triphosphate by heating nickel (II) by evaporation in the presence of borate, urea, salt, and P3m (Figure 4) (Kim and Benner, 2021). Additionally, phosphorus-containing minerals have been implicated in chemical evolution as a source of phosphorus (Pasek and Lauretta, 2005; Saladino et al., 2006; Saladino et al., 2009; Gull et al., 2015; Burcar et al., 2016; Toner and Catling, 2020).
Human cells have a composition similar to that of seawater in modern biological systems (Kuro, 2021). This fact further suggests that the ocean may have been a reaction medium prior to the origin of life (Zhang and Kim, 2010; Abida et al., 2013). Scientists have done some significant experiments in recent years. In aqueous solutions, the dehydration condensation process was shown to be achievable when paired with the hydrolysis of specific condensates. As a prebiotic condensation agent, P3m plays a critical role in the prebiotic phosphorylation of nucleosides (Schramm et al., 1961; Ponnamperuma et al., 1963a; Ponnamperuma et al., 1963b; Kimball and Oro, 1971; Fuller et al., 1972; Schoffstall, 1976). Specially, Moretti and Muller proposed that RNA oligomers could be constructed by P3m (Moretti and Müller, 2014), and the work by Akoopie et al. and Dolan et al. allow the buildup beyond just dimers (Dolan et al., 2015; Akoopie et al., 2021).
Since the 1960s, chemists have attempted to triphosphorylate nucleosides and other alcohols using P3m. However, it appears as though this route has been abandoned due to low yields. The first successful synthesis of nucleoside triphosphates (NTPs) was described, utilizing P3m as a critical reagent (Figure 5). This was performed by reacting the tetrabutylammonium salt of P3m in pyridine with mesitylenesulfonyl chloride in the presence of DABCO, followed by the addition of properly protected nucleosides and phthalimide (Samy et al., 2016).
Schoffstall proposed a prebiotic phosphorylation pathway for nucleosides in the presence of formamide that is mediated by P3m (Schoffstall, 1976; Schoffstall et al., 1982; Schoffstall and Mahone, 1988). Phosphorylation can occur at any point on the ribose throughout this process, finally resulting in the creation of cyclized nucleotides, nucleoside diphosphates, and nucleoside cyclic phosphates were synthesized in formamide solutions containing phosphate and deoxynucleoside at 70° and 120°. These events can be thought of as examples of a distinct sort of prebiotic phosphorylation known as non-aqueous phosphorylation (Figure 6).

FIGURE 6. Reaction mechanism of dipeptide formation from P3m in acidic solution [redrawn from (Ying et al., 2021)].
Hyo-Joong Kim et al. suggested a prebiotic synthesis of phosphorylated nicotinamide ribose that conveniently provides the adenosine phosphate component of this and other RNA cofactors (Kim and Benner, 2018). The mechanisms of nucleoside triphosphorylation were crucial in the emerging “RNA world” because they provided high-energy substrates for reactions such as RNA polymerization. F. Chizzolini et al. established that P3m is suitable for use in mild prebiotics. Under appropriate conditions, it interacted with nucleosides to generate NTP, hence boosting RNA synthesis by T7 RNA polymerase and polymerase ribozymes (Chizzolini et al., 2021).
P3M-Mediated Phosphorylation of Other Biomolecules
Phosphorylation is required for the biochemical functions of living organisms. As a result, the study of the genesis of life is primarily concerned with its origin. The early Earth most likely contained the majority of the components necessary for the emergence and development of life (Izabela et al., 2017). Phosphorylation happened not just on nucleosides, but also on other organic molecules such as amino acids, alcohols, etc.
Phosphorylation of amino acid is a well-characterized post-translational alteration of proteins involved in biological activities (Lai et al., 2017; Kumar and Thompson, 2019; Zientara-Rytter and Subramani, 2019). Phosphorylation, as a critical post-translational modification of proteins, plays a critical role in cell signaling, functional control, and energy transfer in modern organisms (Derouiche et al., 2012; Hunter, 2012; Petkowski et al., 2019; Hu et al., 2020). The primordial source of phosphorylated amino acids required the early Earth to have an adequate supply of amino acids and phosphorus-containing molecules. On early Earth, there were two primary suppliers of amino acids. They were referred to as the extrinsic and endogenous pathways, respectively. The extrinsic pathway refers to amino acid synthesis occurring outside of the earth and being delivered to the earth via interstellar dust particles, meteorites, and so on, whereas the endogenous pathway refers to the atmospheric mixture within the earth via a series of discharges. Abiotic reactions such as hydrothermal fountains synthesize amino acids (Miller, 1953; Zaia et al., 2008; Lin et al., 2020; Takeuchi et al., 2020).
Amino acid phosphorylation is divided into oxygen phosphorylation (O-phosphorylation) and nitrogen phosphorylation (N-phosphorylation), and the two types of prebiotic amino acid phosphorylation mentioned above are described next. The O-phosphorylation is a process that requires the participation of ATP for modern organisms, which plays an indispensable role in the normal metabolism of living organisms. we have proposed a reaction which contained O-phosphorylated (Figure 7). The P3m can react with amino acid in acid aqueous environment, which results the O-phosphorylation (Hill and Orgelr, 2002). The N-phosphoryl amino acid is the simplest phosphoryl amino acid without an ester group on phosphorus, and its possible formation pathways include the reaction of amino acid with polyphosphate, especially the reaction of amino acid and P3m (Rabinowitz et al., 1969; Feng et al., 2009). The main formation mechanism is as follows (Figure 8), the amino group of the α-amino acid attacks P3m to form the P3-AA intermediate, which subsequently becomes cyclic acyl phosphoramidite (CAPA) and pyrophosphate. Since CAPA is unstable, it is subsequently hydrolyzed by ring-opening to N-phosphoryl amino acids (Feng et al., 2010).
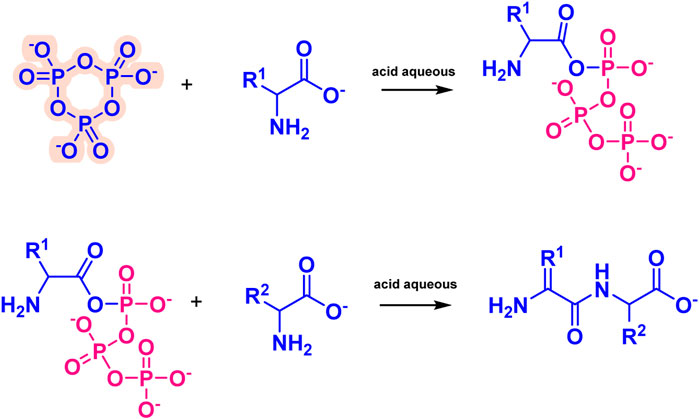
FIGURE 7. Reaction pathway for phosphate-catalyzed synthesis of nucleoside prebiotic sources in the presence of formamide.
Glycerol and glycerophosphate have been shown to be potential prebiotic molecules, Maheen Gull et al. investigate the phosphorylation of glycerol using a variety of inorganic phosphates, including sodium phosphate, P3m, and struvite. P3m was discovered to have a favorable influence on the probiotic phosphorylation of glycerol, and non-aqueous solvents were found to be advantageous for the prebiotic synthesis of biomolecules (Figure 9) (Gull et al., 2017).
We have stressed the function of P3m in fostering chemical evolution in their chemical model of codon and protein co-origin (Ying et al., 2018a). Through a reaction with amino acids and nucleosides in an alkaline aqueous solution, the P3m generates a critical intermediate molecule, nucleotide amidate (aa-N-NMP). The aa-N-NMP is a molecule that joins amino acids and nucleosides via a phosphate group. As the nucleosides and amino acids in the reaction vary, the structure of the resulting aa-N-NMP changes as well, changing the energy required to activate it. As a result, the final dipeptide yields vary (Figure 10A). As we know, aminoacyl-tRNA (aa-tRNA) is generated by transferring an aminoacyl group from 5′-aminoacyladenylates (5′-aa-AMPs) to the tRNA in modern biochemistry. Wonderfully, the structure of aa-N-NMP is comparable to that of 5′-aa-AMPs, a critical activation intermediate in modern organism peptide synthesis. 5′-aa-AMPs are synthesized in living organisms through the interaction of ATP with amino acids, which is catalyzed by a variety of enzymes and cofactors (Figure 10B). Thus, the aa-N-NMP as a mediator of prebiotic tRNA to assist in the activation of amino acids to form peptides could be a potential pathway by which aminoacyl groups from aa-N-NMP are transferred to plausible prebiotic tRNAs. So, in early earth conditions, with environmental changes and other considerations, did 5′-aa-AMP evolve step by step from aa-N-NMPs as an intermediate?
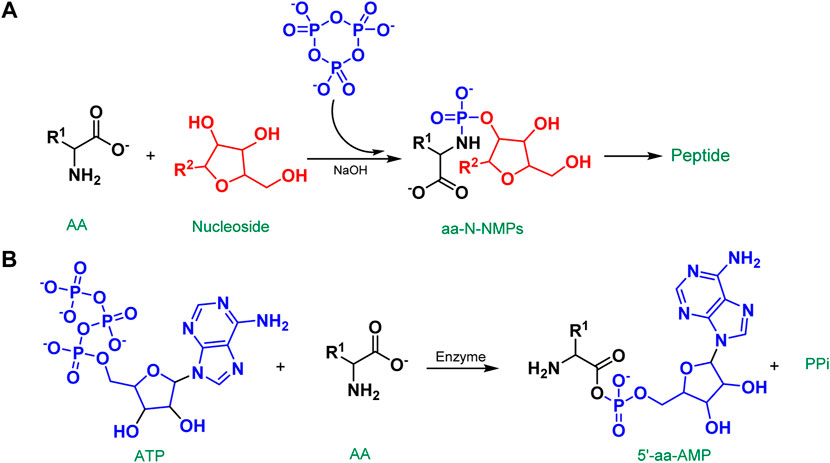
FIGURE 10. (A) The chemical model of the co-origin of codons and proteins; (B) Formation of the 5′-aa-AMP in organism.
Conclusion and Outlook
The beginning of life is an intriguing and perplexing issue. P3m, as a significant condensation agent in prebiotic chemistry, exhibits superior condensation ability and higher water solubility in comparison to other condensation agents, bolstering the theory that life started from the ocean, a shallow pool or other source (Corliss et al., 1979; Kelley et al., 2001; Martin et al., 2008; Burcar et al., 2016). Numerous studies on its involvement in the genesis of life have been conducted in recent years, and P3m is steadily demonstrating its particular appeal in the field of prebiotic chemistry, aiding us in unraveling the riddle of life step by step.
It is unquestionably necessary to investigate non-biosynthetic peptides or nucleotides alone to replicate the early Earth environment in the study of prebiotic chemistry, and scientists have established a certain research base in this area. It is conceivable that chemically selective peptides-nucleotides interactions were involved in the processes of chemical evolution that have contributed to the origin of the genetic code. The shared origin of the genetic code and proteins adds another dimension to the field. While we cannot return to the early Earth to see the entire picture, we can go further and deeper into prebiotic scenarios to identify possible forms of life on the early Earth.
Author Contributions
DG contributed to manuscript preparation, figures preparation and manuscript editing and revision. JY conceived the topic. JY and YZ contributed to manuscript revision.
Funding
This work was supported by the National Natural Science Foundation of China (No. 42003062).
Conflict of Interest
The authors declare that the research was conducted in the absence of any commercial or financial relationships that could be construed as a potential conflict of interest.
Publisher’s Note
All claims expressed in this article are solely those of the authors and do not necessarily represent those of their affiliated organizations, or those of the publisher, the editors and the reviewers. Any product that may be evaluated in this article, or claim that may be made by its manufacturer, is not guaranteed or endorsed by the publisher.
References
Abida, H., Ruchaud, S., Rios, L., Humeau, A., Probert, I., De Vargas, C., et al. (2013). Bioprospecting Marine Plankton. Mar. Drugs 11 (11), 4594–4611. doi:10.3390/md11114594
Akoopie, A., Arriola, J. T., Magde, D., and Müller, U. F. (2021). A GTP-Synthesizing Ribozyme Selected by Metabolic Coupling to an RNA Polymerase Ribozyme. Sci. Adv. 7 (41), eabj7487. doi:10.1126/sciadv.abj7487
Altwegg, K., Balsiger, H., Bar-Nun, A., Berthelier, J.-J., Bieler, A., Bochsler, P., et al. (2016). Prebiotic Chemicals-Amino Acid and Phosphorus-In the Coma of Comet 67P/Churyumov-Gerasimenko. Sci. Adv. 2 (5), e1600285. doi:10.1126/sciadv.1600285
Azzam, G., and Liu, J.-L. (2013). Only One Isoform of Drosophila melanogaster CTP Synthase Forms the Cytoophidium. PLoS Genet. 9 (2), e1003256. doi:10.1371/journal.pgen.1003256
Britvin, S. N., Krivovichev, S. V., Vereshchagin, O. S., Vlasenko, N. S., Shilovskikh, V. V., Krzhizhanovskaya, M. G., et al. (2021). Perryite, (Ni,Fe)16PSi5, from the Mount Egerton Aubrite: the First Natural P-Si-Ordered Phosphide-Silicide. J. Geosci. 66 (4), 189–198. doi:10.3190/jgeosci.331
Brown, M. R. W., and Kornberg, A. (2004). Inorganic Polyphosphate in the Origin and Survival of Species. Proc. Natl. Acad. Sci. U.S.A. 101 (46), 16085–16087. doi:10.1073/pnas.0406909101
Brunk, C. F., and Marshall, C. R. (2021). 'Whole Organism', Systems Biology, and Top-Down Criteria for Evaluating Scenarios for the Origin of Life. Life 11 (7), 690. doi:10.3390/life11070690
Burcar, B., Pasek, M., Gull, M., Cafferty, B. J., Velasco, F., Hud, N. V., et al. (2016). Darwin's Warm Little Pond: A One‐Pot Reaction for Prebiotic Phosphorylation and the Mobilization of Phosphate from Minerals in a Urea‐Based Solvent. Angew. Chem. Int. Ed. 55 (42), 13249–13253. doi:10.1002/anie.201606239
Burton, A. S., Stern, J. C., Elsila, J. E., Glavin, D. P., and Dworkin, J. P. (2012). Understanding Prebiotic Chemistry through the Analysis of Extraterrestrial Amino Acids and Nucleobases in Meteorites. Chem. Soc. Rev. 41 (16), 5459–5472. doi:10.1039/c2cs35109a
Cai, Z., Liu, X., Chen, H., Yang, R., Chen, J., Zou, L., et al. (2021). Variations in Morphology, Physiology, and Multiple Bioactive Constituents of Lonicerae Japonicae Flos under Salt Stress. Sci. Rep. 11 (1), 3939. doi:10.1038/s41598-021-83566-6
Campbell, T. D., Febrian, R., McCarthy, J. T., Kleinschmidt, H. E., Forsythe, J. G., and Bracher, P. J. (2019). Prebiotic Condensation through Wet-Dry Cycling Regulated by Deliquescence. Nat. Commun. 10 (1), 4508. doi:10.1038/s41467-019-11834-1
C, , Gao, X., Zhao, Z.-X., Huang, C., and Zhao, Y.-F. (2010). On the Electrophilicity of Cyclic Acylphosphoramidates (CAPAs) Postulated as Intermediates. Eur. J. Org. Chem. 2009 (18), 3026–3035. doi:10.1002/ejoc.200900227
Chizzolini, F., Kent, A. D., Passalacqua, L. F. M., and Lupták, A. (2021). Enzymatic RNA Production from NTPs Synthesized from Nucleosides and Trimetaphosphate**. ChemBioChem 22, 2098–2101. doi:10.1002/cbic.202100085
Christ, J. J., Willbold, S., and Blank, L. M. (2020). Methods for the Analysis of Polyphosphate in the Life Sciences. Anal. Chem. 92 (6), 4167–4176. doi:10.1021/acs.analchem.9b05144
Chung, N. M., Lohrmann, R., Orgel, L. E., and Rabinowitz, J. (1971). The Mechanism of the Trimetaphosphate-Induced Peptide Synthesis. Tetrahedron 27 (6), 1205–1210. doi:10.1016/S0040-4020(01)90868-3
Corliss, J. B., Dymond, J., Gordon, L. I., Edmond, J. M., von Herzen, R. P., Ballard, R. D., et al. (1979). Submarine Thermal Springs on the Galápagos Rift. Science 203 (4385), 1073–1083. doi:10.1126/science.203.4385.1073
de Graaf, R. M., Visscher, J., and Schwartz, A. W. (1995). A Plausibly Prebiotic Synthesis of Phosphonic Acids. Nature 378 (6556), 474–477. doi:10.1038/378474a0
Derouiche, A., Cousin, C., and Mijakovic, I. (2012). Protein Phosphorylation from the Perspective of Systems Biology. Curr. Opin. Biotechnol. 23 (4), 585–590. doi:10.1016/j.copbio.2011.11.008
Dolan, G. F., Akoopie, A., and Müller, U. F. (2015). A Faster Triphosphorylation Ribozyme. PLoS One 10 (11), e0142559. doi:10.1371/journal.pone.0142559
Dürr-Mayer, T., Qiu, D., Eisenbeis, V. B., Steck, N., Häner, M., Hofer, A., et al. (2021). The Chemistry of Branched Condensed Phosphates. Nat. Commun. 12 (1), 5368. doi:10.1038/s41467-021-25668-3
Ferris, J. P. (1968). Cyanovinyl Phosphate: a Prebiological Phosphorylating Agent? Science 161 (3836), 53–54. doi:10.1126/science.161.3836.53
Fiore, M. (2019). The Origin and Early Evolution of Life: Prebiotic Chemistry. Life 9 (3), 73. doi:10.3390/life9030073
Forsythe, J. G., Yu, S. S., Mamajanov, I., Grover, M. A., Krishnamurthy, R., Fernández, F. M., et al. (2015). Ester‐Mediated Amide Bond Formation Driven by Wet-Dry Cycles: A Possible Path to Polypeptides on the Prebiotic Earth. Angew. Chem. Int. Ed. 54 (34), 9871–9875. doi:10.1002/anie.201503792
Fox, S. W., and Waehneldt, T. V. (1968). The Therml Synthesis of Neutral and Basic Proteinoids. Biochimica Biophysica Acta (BBA) - Protein Struct. 160 (2), 246–249. doi:10.1016/0005-2795(68)90093-7
Fuller, W. D., Sanchez, R. A., and Orgel, L. E. (1972). Studies in Prebiotic Synthesis. J. Mol. Biol. 67 (1), 25–33. doi:10.1016/0022-2836(72)90383-x
Gabel, N. W., and Ponnamperuma, C. (1967). Model for Origin of Monosaccharides. Nature 216 (5114), 453–455. doi:10.1038/216453a0
Gibard, C., Bhowmik, S., Karki, M., Kim, E.-K., and Krishnamurthy, R. (2018). Phosphorylation, Oligomerization and Self-Assembly in Water under Potential Prebiotic Conditions. Nat. Chem. 10 (2), 212–217. doi:10.1038/nchem.2878
Gibard, C., Gorrell, I. B., Jiménez, E. I., Kee, T. P., Pasek, M. A., and Krishnamurthy, R. (2019). Geochemical Sources and Availability of Amidophosphates on the Early Earth. Angew. Chem. Int. Ed. 58 (24), 8151–8155. doi:10.1002/anie.201903808
Glonek, T. (2021). Did Cyclic Metaphosphates Have a Role in the Origin of Life? Orig. Life Evol. Biosph. 51 (1), 1–60. doi:10.1007/s11084-021-09604-5
Griffith, E. J., Ponnamperuma, C., and Gabel, N. W. (1977). Phosphorus, a Key to Life on the Primitive Earth. Orig. Life Evol. Biosphere 8 (2), 71–85. doi:10.1007/bf00927976
Gu, L., Kim, Y. K., Liu, Y., Ryou, H., Wimmer, C. E., Dai, L., et al. (2011). Biomimetic Analogs for Collagen Biomineralization. J. Dent. Res. 90 (1), 82–87. doi:10.1177/0022034510385241
Gulick, A. (1957). Phosphorus and the Origin of Life. Ann. N. Y. Acad. Sci. 69 (2), 309–313. doi:10.1111/j.1749-6632.1957.tb49666.x
Gull, M., Cafferty, B., Hud, N., and Pasek, M. (2017). Silicate-Promoted Phosphorylation of Glycerol in Non-aqueous Solvents: A Prebiotically Plausible Route to Organophosphates. Life 7 (3), 29. doi:10.3390/life7030029
Gull, M., Mojica, M. A., Fernández, F. M., Gaul, D. A., Orlando, T. M., Liotta, C. L., et al. (2015). Nucleoside Phosphorylation by the Mineral Schreibersite. Sci. Rep. 5, 17198. doi:10.1038/srep17198
Gull, M., Zhou, M., Fernández, F. M., and Pasek, M. A. (2014). Prebiotic Phosphate Ester Syntheses in a Deep Eutectic Solvent. J. Mol. Evol. 78 (2), 109–117. doi:10.1007/s00239-013-9605-9
Guo, Y., Ying, J., Sun, D., Zhang, Y., Zheng, M., Ding, R., et al. (2021). Cyclic Dipeptides Formation from Linear Dipeptides under Potentially Prebiotic Earth Conditions. Front. Chem. 9, 675821. doi:10.3389/fchem.2021.675821
Hagan, W. J., Parker, A., Steuerwald, A., and Hathaway, M. (2007). Phosphate Solubility and the Cyanate-Mediated Synthesis of Pyrophosphate. Orig. Life Evol. Biosph. 37 (2), 113–122. doi:10.1007/s11084-006-9020-y
Handschuh, G. J., Lohrmann, R., and Orgel, L. E. (1973). The Effect of Mg2+ and Ca2+ on Urea-Catalyzed Phosphorylation Reactions. J. Mol. Evol. 2 (4), 251–262. doi:10.1007/bf01654094
Hayakawa, T., Windsor, C. R., and Fox, S. W. (1967). Copolymerization of the Leuchs Anhydrides of the Eighteen Amino Acids Common to Protein. Archives Biochem. Biophysics 118 (2), 265–272. doi:10.1016/0003-9861(67)90347-5
Hill, A., and Orgelr, L. E. (2002). Trimetaphosphate-Induced Addition of Aspartic Acid to Oligo(glutamic Acid)s. Helv. Chim. Acta. doi:10.1002/hlca.200290009
Holm, N. G., and Baltscheffsky, H. (2011). Links between Hydrothermal Environments, Pyrophosphate, Na+, and Early Evolution. Orig. Life Evol. Biosph. 41 (5), 483–493. doi:10.1007/s11084-011-9235-4
Hu, Y., Jiang, B., Weng, Y., Sui, Z., Zhao, B., Chen, Y., et al. (2020). Bis(Zinc(II)-Dipicolylamine)-Functionalized Sub-2 μm Core-Shell Microspheres for the Analysis of N-Phosphoproteome. Nat. Commun. 11 (1), 6226. doi:10.1038/s41467-020-20026-1
Hulshof, J. e., and Ponnamperuma, C. (1976). Prebiotic Condensation Reactions in an Aqueous Medium: a Review of Condensing Agents. Orig. Life Evol. Biosphere 7 (3), 197–224. doi:10.1007/bf00926938
Hunter, T. (2012). Why Nature Chose Phosphate to Modify Proteins. Phil. Trans. R. Soc. B 367 (1602), 2513–2516. doi:10.1098/rstb.2012.0013
Karki, M., Gibard, C., Bhowmik, S., and Krishnamurthy, R. (2017). Nitrogenous Derivatives of Phosphorus and the Origins of Life: Plausible Prebiotic Phosphorylating Agents in Water. Life 7 (3), 32. doi:10.3390/life7030032
Kee, T. P., and Monnard, P.-A. (2017). Chemical Systems, Chemical Contiguity and the Emergence of Life. Beilstein J. Org. Chem. 13, 1551–1563. doi:10.3762/bjoc.13.155
Keefe, A., and Miller, S. (1995). Are Polyphosphates or Phosphate Esters Prebiotic Reagents? J. Mol. Evol. 41 (6), 693–702. doi:10.1007/bf00173147
Kelley, D. S., Karson, J. A., Karson, J. A., Blackman, D. K., Früh-Green, G. L., Butterfield, D. A., et al. (2001). An off-axis Hydrothermal Vent Field Near the Mid-Atlantic Ridge at 30° N. Nature 412 (6843), 145–149. doi:10.1038/35084000
Kim, H.-J., and Benner, S. A. (2018). A Direct Prebiotic Synthesis of Nicotinamide Nucleotide. Chem. Eur. J. 24 (3), 581–584. doi:10.1002/chem.201705394
Kim, H.-J., and Benner, S. A. (2021). Abiotic Synthesis of Nucleoside 5′-Triphosphates with Nickel Borate and Cyclic Trimetaphosphate (CTMP). Astrobiology 21 (3), 298–306. doi:10.1089/ast.2020.2264
Knecht, W., Sandrini, M. P., Johansson, K., Eklund, H., Munch-Petersen, B., and Piskur, J. (2002). A Few Amino Acid Substitutions Can Convert Deoxyribonucleoside Kinase Specificity from Pyrimidines to Purines. Embo. J. 21 (7), 1873–1880. doi:10.1093/emboj/21.7.1873
Kumar, R., and Thompson, E. (2019). Role of Phosphorylation in the Modulation of the Glucocorticoid Receptor's Intrinsically Disordered Domain. Biomolecules 9 (3), 95. doi:10.3390/biom9030095
Kuro-o, M. (2021). Phosphate as a Pathogen of Arteriosclerosis and Aging. Jat 28 (3), 203–213. doi:10.5551/jat.RV17045
Lai, S.-J., Tu, I.-F., Wu, W.-L., Yang, J.-T., Luk, L. Y. P., Lai, M.-C., et al. (2017). Site-specific His/Asp Phosphoproteomic Analysis of Prokaryotes Reveals Putative Targets for Drug Resistance. Bmc. Microbiol. 17 (1), 123. doi:10.1186/s12866-017-1034-2
Leach, S., Smith, I. W. M., and Cockell, C. S. (2006). Introduction: Conditions for the Emergence of Life on the Early Earth. Phil. Trans. R. Soc. B 361 (1474), 1675–1679. doi:10.1098/rstb.2006.1895
Lin, L., Caton-Williams, J., Kaur, M., Patino, A. M., Sheng, J., Punetha, J., et al. (2011). Facile Synthesis of Nucleoside 5′-(α-P-Seleno)-Triphosphates and Phosphoroselenoate RNA Transcription. RNA 17 (10), 1932–1938. doi:10.1261/rna.2719311
Lin, R., Wang, Y., Li, X., Liu, Y., and Zhao, Y. (2020). pH-Dependent Adsorption of Peptides on Montmorillonite for Resisting UV Irradiation. Life 10 (4), 45. doi:10.3390/life10040045
Liu, N., Xu, H., Sun, Q., Yu, X., Chen, W., Wei, H., et al. (2021). The Role of Oxidative Stress in Hyperuricemia and Xanthine Oxidoreductase (XOR) Inhibitors. Oxidative Med. Cell. Longev. 2021, 15. doi:10.1155/2021/1470380
Lohrmann, R. (1975). Formation of Nucleoside 5′-polyphosphates from Nucleotides and Trimetaphosphate. J. Mol. Evol. 6 (4), 237–252. doi:10.1007/bf01794633
Lohrmann, R., and Orgel, L. E. (1968). Prebiotic Synthesis: Phosphorylation in Aqueous Solution. Science 161 (3836), 64–66. doi:10.1126/science.161.3836.64
Lohrmann, R., and Orgel, L. E. (1971). Urea-inorganic Phosphate Mixtures as Prebiotic Phosphorylating Agents. Science 171 (3970), 490–494. doi:10.1126/science.171.3970.490
Macia, E., Hernandez, M. V., and Oro, J. (1997). Primary Sources of Phosphorus and Phosphates in Chemical Evolution. Orig. Life Evol. Biosph. 27 (5-6), 459–480. doi:10.1023/a:1006523226472
Martin, W., Baross, J., Kelley, D., and Russell, M. J. (2008). Hydrothermal Vents and the Origin of Life. Nat. Rev. Microbiol. 6 (11), 805–814. doi:10.1038/nrmicro1991
Martínez-Bachs, B., and Rimola, A. (2019). Prebiotic Peptide Bond Formation through Amino Acid Phosphorylation. Insights from Quantum Chemical Simulations. Life 9 (3), 75. doi:10.3390/life9030075
Micca Longo, G., Vialetto, L., Diomede, P., Longo, S., and Laporta, V. (2021). Plasma Modeling and Prebiotic Chemistry: A Review of the State-Of-The-Art and Perspectives. Molecules 26 (12), 3663. doi:10.3390/molecules26123663
Miggiano, R., Morrone, C., Rossi, F., and Rizzi, M. (2020). Targeting Genome Integrity in Mycobacterium Tuberculosis: From Nucleotide Synthesis to DNA Replication and Repair. Molecules 25 (5), 1205. doi:10.3390/molecules25051205
Miller, S. L. (1953). A Production of Amino Acids under Possible Primitive Earth Conditions. Science 117 (3046), 528–529. doi:10.1126/science.117.3046.528
Mizuno, Y., Kitano, S., and Nomura, A. (1975). Nucleotides. VI. Syntheses and Spectral Properties of Some Deazaadenylyl-Deazaadenosines (Dinucleoside Monophosphates with Unusual CD Spectrum) and Closely Related Dinucleoside Monophosphates. Nucl. Acids Res. 2 (12), 2193–2208. doi:10.1093/nar/2.12.2193
Mohamady, S., Taylor, S. D., and Letters, T. O. (2016). Synthesis of Nucleoside Triphosphates from 2'-3'-Protected Nucleosides Using Trimetaphosphate. Org. Lett. 18 (3), 580–583. doi:10.1021/acs.orglett.5b03624
Moretti, J. E., and Müller, U. F. (2014). A Ribozyme that Triphosphorylates RNA 5′-hydroxyl Groups. Nucleic. acids. Res. 42 (7), 4767–4778. doi:10.1093/nar/gkt1405
Omelon, S., Georgiou, J., Henneman, Z. J., Wise, L. M., Sukhu, B., Hunt, T., et al. (2009). Control of Vertebrate Skeletal Mineralization by Polyphosphates. PLoS One 4 (5), e5634. doi:10.1371/journal.pone.0005634
Oro, J. (1963). Studies in Experimental Organic Cosmochemistry. Ann. N. Y. Acad. Sci. 108, 464–481. doi:10.1111/j.1749-6632.1963.tb13402.x
Pasek, M. A., Harnmeijer, J. P., Buick, R., Gull, M., and Atlas, Z. (2013). Evidence for Reactive Reduced Phosphorus Species in the Early Archean Ocean. Proc. Natl. Acad. Sci. U.S.A. 110 (25), 10089–10094. doi:10.1073/pnas.1303904110
Pasek, M. A., Kee, T. P., Bryant, D. E., Pavlov, A. A., and Lunine, J. I. (2008). Production of Potentially Prebiotic Condensed Phosphates by Phosphorus Redox Chemistry. Angew. Chem. Int. Ed. 47 (41), 7918–7920. doi:10.1002/anie.200802145
Pasek, M. A., and Lauretta, D. S. (2005). Aqueous Corrosion of Phosphide Minerals from Iron Meteorites: a Highly Reactive Source of Prebiotic Phosphorus on the Surface of the Early Earth. Astrobiology 5 (4), 515–535. doi:10.1089/ast.2005.5.515
Petkowski, J., Bains, W., and Seager, S. (2019). Natural Products Containing 'Rare' Organophosphorus Functional Groups. Molecules 24 (5), 866. doi:10.3390/molecules24050866
Ponnamperuma, C., and Mack, R. (1965). Nucleotide Synthesis under Possible Primitive Earth Conditions. Science 148 (3674), 1221–1223. doi:10.1126/science.148.3674.1221
Ponnamperuma, C., Mariner, R., and Sagan, C. (1963a). Formation of Adenosine by Ultra-violet Irradiation of a Solution of Adenine and Ribose. Nature 198, 1199–1200. doi:10.1038/1981199a0
Ponnamperuma, C., Sagan, C., and Mariner, R. (1963b). Synthesis of Adenosine Triphosphate under Possible Primitive Earth Conditions. Nature 199, 222–226. doi:10.1038/199222a0
Rabinowitz, J., Chang, S., and Ponnamperuma, C. (1968). Phosphorylation on the Primitive Earth: Phosphorylation by Way of Inorganic Phosphate as a Potential Prebiotic Process. Nature 218 (5140), 442–443. doi:10.1038/218442a0
Rabinowitz, J., Flores, J., Krebsbach, R., and Rogers, G. (1969). Peptide Formation in the Presence of Linear or Cyclic Polyphosphates. Nature 224 (5221), 795–796. doi:10.1038/224795a0
Rabinowitz, J. (1970). Peptide and Amide Bond Formation in Aqueous Solutions of Cyclic or Linear Polyphosphates as a Possible Prebiotic Process. Helv. Chim. Acta 53 (6), 1350–1355. doi:10.1002/hlca.19700530614
Ritson, D. J., Mojzsis, S. J., and Sutherland, J. D. (2020). Supply of Phosphate to Early Earth by Photogeochemistry after Meteoritic Weathering. Nat. Geosci. 13 (5), 344–348. doi:10.1038/s41561-020-0556-7
Saladino, R., Crestini, C., Ciciriello, F., Di Mauro, E., and Costanzo, G. (2006). Origin of Informational Polymers: Differential Stability of Phosphoester Bonds in Ribomonomers and Ribooligomers. J. Biol. Chem. 281 (9), 5790–5796. doi:10.1074/jbc.M512545200
Saladino, R., Crestini, C., Ciciriello, F., Pino, S., Costanzo, G., and Di Mauro, E. (2009). From Formamide to RNA: the Roles of Formamide and Water in the Evolution of Chemical Information. Res. Microbiol. 160 (7), 441–448. doi:10.1016/j.resmic.2009.06.001
Schoffstall, A. M., Barto, R. J., and Ramos, D. L. (1982). Nucleoside and Deoxynucleoside Phosphorylation in Formamide Solutions. Orig. Life Evol. Biosphere 12 (2), 143–151. doi:10.1007/bf00927141
Schoffstall, A. M., and Mahone, S. M. (1988). Formate Ester Formation in Amide Solutions. Orig. Life Evol. Biosphere 18 (4), 389–396. doi:10.1007/bf01808217
Schoffstall, A. M. (1976). Prebiotic Phosphorylation of Nucleosides in Formamide. Orig. Life Evol. Biosphere 7 (4), 399–412. doi:10.1007/bf00927935
Schramm, G., Grotsch, D. H., and Pollmann, W. (1961). Nicht‐enzymatische Synthese von Polysacchariden, Nucleosiden und Nucleinsuren. Angew. Chem. 73 (17-18), 619. doi:10.1021/acs.orglett.5b0362410.1002/ange.19610731707
Schwartz, A., and Fox, S. W. (1964). Thermal Synthesis of Internucleotide Phosphodiester Linkages. Biochimica Biophysica Acta (BBA) - Specialized Sect. Nucleic Acids Relat. Subj. 87, 694–696. doi:10.1016/0926-6550(64)90290-7
Schwartz, A., and Ponnamperuma, C. (1968). Phosphorylation on the Primitive Earth: Phosphorylation of Adenosine with Linear Polyphosphate Salts in Aqueous Solution. Nature 218 (5140), 443. doi:10.1038/218443a0
Schwartz, A. W. (2006). Phosphorus in Prebiotic Chemistry. Phil. Trans. R. Soc. B 361 (1474), 1743–1749. doi:10.1098/rstb.2006.1901
Serov, N. Y., Shtyrlin, V. G., and Khayarov, K. R. (2020). The Kinetics and Mechanisms of Reactions in the Flow Systems Glycine-Sodium Trimetaphosphate-Imidazoles: the Crucial Role of Imidazoles in Prebiotic Peptide Syntheses. Amino Acids 52 (5), 811–821. doi:10.1007/s00726-020-02854-z
Shivakumar, M., Miller, J. E., Dasari, V. R., Gogoi, R., and Kim, D. (2019). Exome-Wide Rare Variant Analysis from the DiscovEHR Study Identifies Novel Candidate Predisposition Genes for Endometrial Cancer. Front. Oncol. 9, 574. doi:10.3389/fonc.2019.00574
Sibilska, I., Chen, B., Li, L., and Yin, J. (2017). Effects of Trimetaphosphate on Abiotic Formation and Hydrolysis of Peptides. Life 7 (4), 50. doi:10.3390/life7040050
Sibilska, I., Chen, B., Li, L., and Yin, J. (2017). Effects of Trimetaphosphate on Abiotic Formation and Hydrolysis of Peptides. Life 7 (4), 50. doi:10.3390/life7040050
Sibilska, I., Feng, Y., Li, L., and Yin, J. (2018). Trimetaphosphate Activates Prebiotic Peptide Synthesis across a Wide Range of Temperature and pH. Orig. Life Evol. Biosph. 48 (3), 277–287. doi:10.1007/s11084-018-9564-7
Stephen-Sherwood, E., Oró, J., and Kimball, A. P. (1971). Thymine: A Possible Prebiotic Synthesis. Science 30173 (3995), 446–447. doi:10.1126/science.173.3995.446
Takeuchi, Y., Furukawa, Y., Kobayashi, T., Sekine, T., Terada, N., and Kakegawa, T. (2020). Impact-induced Amino Acid Formation on Hadean Earth and Noachian Mars. Sci. Rep. 10 (1), 9220. doi:10.1038/s41598-020-66112-8
Toner, J. D., and Catling, D. C. (2020). A Carbonate-Rich Lake Solution to the Phosphate Problem of the Origin of Life. Proc. Natl. Acad. Sci. U.S.A. 117 (2), 883–888. doi:10.1073/pnas.1916109117
Toparlak, O. D., and Mansy, S. S. (2019). Progress in Synthesizing Protocells. Exp. Biol. Med. (Maywood) 244 (4), 304–313. doi:10.1177/1535370218816657
Ulian, G., Moro, D., and Valdrè, G. (2021). Hydroxylapatite and Related Minerals in Bone and Dental Tissues: Structural, Spectroscopic and Mechanical Properties from a Computational Perspective. Biomolecules 11 (5), 728. doi:10.3390/biom11050728
Urey, H. C. (1952). On the Early Chemical History of the Earth and the Origin of Life. Proc. Natl. Acad. Sci. U.S.A. 38 (4), 351–363. doi:10.1073/pnas.38.4.351
V, , Sun, S., Huang, C., Zhao, Y., and Yufen, (2009). N-Phosphorylation of Amino Acids by Trimetaphosphate in Aqueous Solution-Learning from Prebiotic Synthesis. Green Chem. 11 (4), 569–573. doi:10.1039/B817013D
Weber, A. L. (1982). Formation of Pyrophosphate on Hydroxyapatite with Thioesters as Condensing Agents. Biosystems 15 (3), 183–189. doi:10.1016/0303-2647(82)90002-8
Weber, A. L. (1981). Formation of Pyrophosphate, Tripolyphosphate, and Phosphorylimidazole with the Thioester, N, S-Diacetylcysteamine, as the Condensing Agent. J. Mol. Evol. 18 (1), 24–29. doi:10.1007/bf01733208
Westheimer, F. H. (1987). Why Nature Chose Phosphates. Science 235 (4793), 1173–1178. doi:10.1126/science.2434996
Yamagata, Y., and Inomata, K. (1997). Condensation of Glycylglycine to Oligoglycines with Trimetaphosphate in Aqueous Solution. II: Catalytic Effect of Magnesium Ion. Orig. Life Evol. Biosph. 27 (4), 339–344. doi:10.1023/a:1006529421813
Yamagata, Y., Matsukawa, T., Mohri, T., and Inomata, K. (1979). Phosphorylation of Adenosine in Aqueous Solution by Electric Discharges. Nature 282 (5736), 284–286. doi:10.1038/282284a0
Yamagata, Y., Watanabe, H., Saitoh, M., and Namba, T. (1991). Volcanic Production of Polyphosphates and its Relevance to Prebiotic Evolution. Nature 352 (6335), 516–519. doi:10.1038/352516a0
Yamanaka, J., Inomata, K., and Yamagata, Y. (1988). Condensation of Oligoglycines with Trimeta- and Tetrametaphosphate in Aqueous Solutions. Orig. Life Evol. Biosphere 18 (3), 165–178. doi:10.1007/bf01804669
Yanagawa, H., Kojima, K., Ito, M., and Handa, N. (1990). Synthesis of Polypeptides by Microwave Heating I. Formation of Polypeptides during Repeated Hydration-Dehydration Cycles and Their Characterization. J. Mol. Evol. 31 (3), 180–186. doi:10.1007/bf02109494
Ying, J., Ding, R., Liu, Y., and Zhao, Y. (2021). Prebiotic Chemistry in Aqueous Environment:A Review of Peptide Synthesis and its Relationship with Genetic Code. Chin. J. Chem. 39 (8), 9. doi:10.1002/cjoc.202100120
Ying, J., Fu, S., Li, X., Feng, L., Xu, P., Liu, Y., et al. (2018a). A Plausible Model Correlates Prebiotic Peptide Synthesis with the Primordial Genetic Code. Chem. Commun. 54 (62), 8598–8601. doi:10.1039/c8cc04767g
Ying, J., Lin, R., Xu, P., Wu, Y., Liu, Y., and Zhao, Y. (2018b). Prebiotic Formation of Cyclic Dipeptides under Potentially Early Earth Conditions. Sci. Rep. 8 (1), 936. doi:10.1038/s41598-018-19335-9
Zaia, D. A. M., Zaia, C. T. B. V., and De Santana, H. (2008). Which Amino Acids Should Be Used in Prebiotic Chemistry Studies? Orig. Life Evol. Biosph. 38 (6), 469–488. doi:10.1007/s11084-008-9150-5
Zhang, C., and Kim, S.-K. (2010). Research and Application of Marine Microbial Enzymes: Status and Prospects. Mar. Drugs 8 (6), 1920–1934. doi:10.3390/md8061920
Zientara-Rytter, K., and Subramani, S. (2019). The Roles of Ubiquitin-Binding Protein Shuttles in the Degradative Fate of Ubiquitinated Proteins in the Ubiquitin-Proteasome System and Autophagy. Cells 8 (1), 40. doi:10.3390/cells8010040
Keywords: trimetaphosphate, prebiotic chemistry, peptide, nucleotide, origin of life
Citation: Gan D, Ying J and Zhao Y (2022) Prebiotic Chemistry: The Role of Trimetaphosphate in Prebiotic Chemical Evolution. Front. Chem. 10:941228. doi: 10.3389/fchem.2022.941228
Received: 11 May 2022; Accepted: 23 June 2022;
Published: 13 July 2022.
Edited by:
Qinheng Zheng, University of California, San Francisco, United StatesReviewed by:
Brian Cafferty, Beam Therapeutics, United StatesMatthew A. Pasek, University of South Florida, United States
Copyright © 2022 Gan, Ying and Zhao. This is an open-access article distributed under the terms of the Creative Commons Attribution License (CC BY). The use, distribution or reproduction in other forums is permitted, provided the original author(s) and the copyright owner(s) are credited and that the original publication in this journal is cited, in accordance with accepted academic practice. No use, distribution or reproduction is permitted which does not comply with these terms.
*Correspondence: Jianxi Ying, eWluZ2ppYW54aUBuYnUuZWR1LmNu