- Department of Chemistry, Biochemistry, and Pharmaceutical Sciences (DCBP), Faculty of Science, University of Bern, Bern, Switzerland
The continuing rapid expansion of 99mTc diagnostic agents always calls for scaling up 99mTc production to cover increasing clinical demand. Nevertheless, 99mTc availability depends mainly on the fission-produced 99Mo supply. This supply is seriously influenced during renewed emergency periods, such as the past 99Mo production crisis or the current COVID-19 pandemic. Consequently, these interruptions have promoted the need for 99mTc production through alternative strategies capable of providing clinical-grade 99mTc with high purity. In the light of this context, this review illustrates diverse production routes that either have commercially been used or new strategies that offer potential solutions to promote a rapid production growth of 99mTc. These techniques have been selected, highlighted, and evaluated to imply their impact on developing 99mTc production. Furthermore, their advantages and limitations, current situation, and long-term perspective were also discussed. It appears that, on the one hand, careful attention needs to be devoted to enhancing the 99Mo economy. It can be achieved by utilizing 98Mo neutron activation in commercial nuclear power reactors and using accelerator-based 99Mo production, especially the photonuclear transmutation strategy. On the other hand, more research efforts should be devoted to widening the utility of 99Mo/99mTc generators, which incorporate nanomaterial-based sorbents and promote their development, validation, and full automization in the near future. These strategies are expected to play a vital role in providing sufficient clinical-grade 99mTc, resulting in a reasonable cost per patient dose.
1 Introduction
Short-lived radionuclides continue to prove their crucial role in the development of nuclear medicine utilization in both diagnostic and therapeutic procedures. Effective therapy involves the delivery of a specific radiation dose to the tumor tissues either by the use of an external radiation source or internal radiotherapy techniques. For the former, hard gamma-ray emitters are favorably used, while a great need for the radionuclides with a low range of highly ionizing radiation emissions, such as alpha, beta and/or Auger electrons, are required to achieve internal therapy successfully (IAEA, 2009; Qaim, 2019). Definitely, a profitable therapy protocol strongly depends on the delivery of accurate information about the cancer lesion, such as the shape, size, and blood flow to the affected organ. To achieve this step, high-quality tumor imaging must be performed with the help of in-vivo diagnostic investigation tools. This method can be divided into two main categories, Positron Emission Tomography (PET), which includes the use of several positron emitters, and Single Photon Emission Computed Tomography (SPECT), in which radionuclides emitting low energetic gamma radiation are of great interest (Pimlott and Sutherland, 2011).
Particularly, SPECT radionuclides should have one or at least one main gamma energy line in the range of 100–200 keV. Table 1 displays the most commonly used radionuclides in SPECT imaging processes (Pimlott and Sutherland, 2011; Tàrkànyi et al., 2019).
To describe the pivotal role of 99mTc, S. Esarey called it a vitally crucial diagnostic isotope used nearly once a second worldwide (IAEA, 2011). Undoubtedly, 99mTc is the most extensively used diagnostic radionuclide in the SPECT family. It is currently involved in 80–85% of all in-vivo nuclear medicine diagnostic examinations (Boschi et al., 2017; Gumiela, 2018; Martini et al., 2018; Osso et al., 2012; Owen et al., 2014; Sakr et al., 2017). Annually, 30–40 million SPECT imaging studies have been conducted worldwide using 99mTc solely (half of which in the United States). 99mTc utilization is estimated to increase by 3–8% every year (Banerjee et al., 2000; Eckelman, 2009; Guerin et al., 2010; IAEA, 2013a; Chakravarty et al., 2013; Owen et al., 2014; Dilworth and Pascu, 2015; Gumiela, 2018; Haroon and Nichita, 2021). 99mTc decays by a relatively short half-life (T1/2 = 6.01 h) and emits only one-gamma photon at low energy of 140.51 keV. This energy is ideally suited to penetrate the tissues and can be efficiently detected through SPECT cameras from outside the body, resulting in a high-quality image of the target organs with minimum radiation exposure dose to the patient. In addition, 99mTc has unique coordination chemistry, which allows conjugation with a broad spectrum of diverse pharmaceutical molecules (IAEA, 2009; Dilworth and Pascu, 2015; Boschi et al., 2017; Gumiela, 2018). These 99mTc-labelled compounds have been involved in the visualization of most organs (Vallabhajosula et al., 1989; Zolle, 2007; Leggett and Giussani, 2015; Araz et al., 2020; Urbano et al., 2020; Parihar et al., 2021). Moreover, the global on-demand availability of 99mTc through the user-friendly 99Mo/99mTc generators with reasonable cost-effectiveness has greatly encouraged nuclear medicine departments to conduct many research studies to explore its pivotal role in diagnostic imaging in detail (Mikaeili et al., 2018; Gan et al., 2020; Ávila-Sánchez et al., 2020). Table 2 highlights the wide-scale applicability of 99mTc-based radiopharmaceuticals and provides the injection activity and the estimated exposure dose for each diagnostic procedure. Furthermore, based on its accessible availability and favorable nuclear properties, 99mTc received considerable attention in multi-disciplinary fields. Recently, it has been involved in a variety of industrial radiotracer applications (Fauquex et al., 1983; Kantzas et al., 2000; Bandeira et al., 2002; Borroto et al., 2003; Berne and Thereska, 2004; Hughes et al., 2004; Thyn and Zitny, 2004; Dash et al., 2012; Pavlovicl et al., 2020).
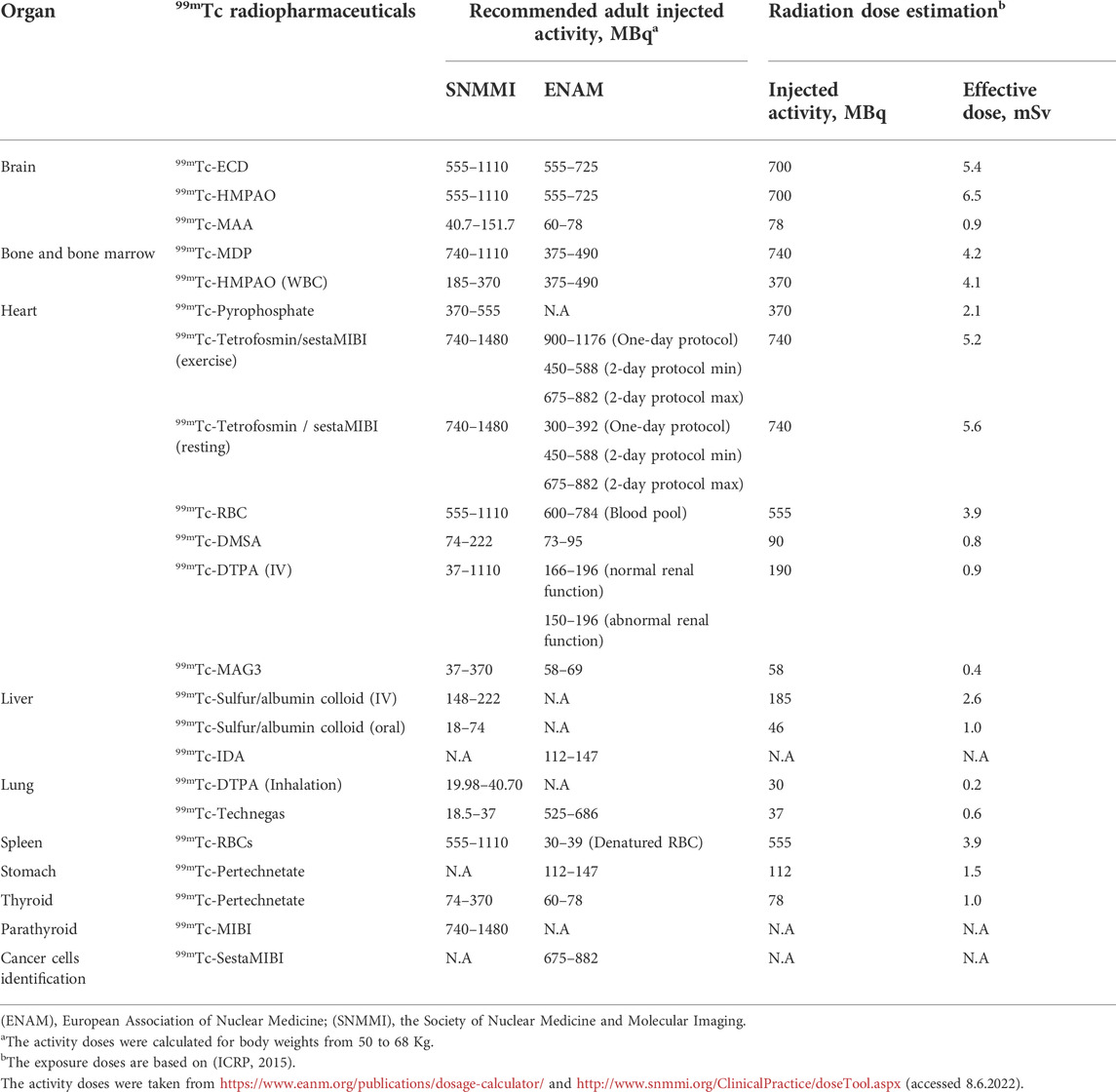
TABLE 2. The most commonly used 99mTc-based radiopharmaceuticals with their recommended injection activity and the estimated exposure dose.
Despite the central role of 99mTc for different diagnostic medical investigations as well as recent industrial implementations, its limited availability at the usage sites is a real difficulty. The primary objective of this review paper is to provide a detailed and comprehensive evaluation of the different production routes of 99mTc that have commercially been exploited. In addition, new production strategies that could potentially promote efficient utilization of 99mTc in the near future are highlighted. In the following sections, the paper discusses the different production routes of the parent 99Mo either by nuclear reactors or particle accelerators, the direct production technology of 99mTc at a cyclotron, and the different 99Mo/99mTc generator systems and their role in supplying 99mTc. Furthermore, the paper underlines the advantages, outcomes, and technical challenges of the different 99mTc production approaches along with their current situation and the possible future perspectives.
2 Production and supply of 99Mo
The increasing interest in 99mTc-labelled radiopharmaceuticals for diagnostic purposes has heightened the need for securing a sufficient supply of 99Mo. Practically, almost all of the used clinical-grade 99mTc in nuclear medicine is derived from 99Mo/ 99mTc generators. In this system, 99Mo (T1/2 = 65.94 h) yields 99mTc by beta β− decay, as shown in Figure 1 (Boyd, 1982a; Hidalgo et al., 1967; IAEA, 2017, 2013a; NEA, 2017, 2010). 99Mo is produced with the use of one of two main facilities; nuclear reactors or particle accelerators. Figure 2 displays the potential production routes of 99Mo and 99mTc.
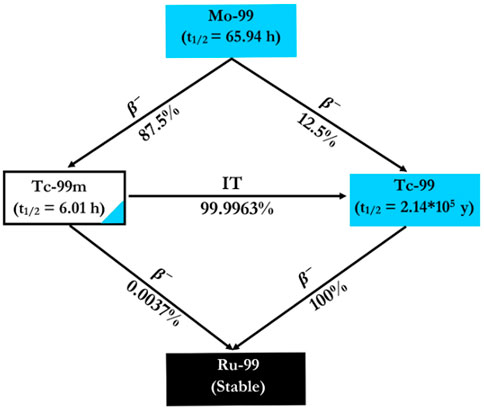
FIGURE 1. The decay scheme of 99Mo and 99mTc (Hidalgo et al., 1967).
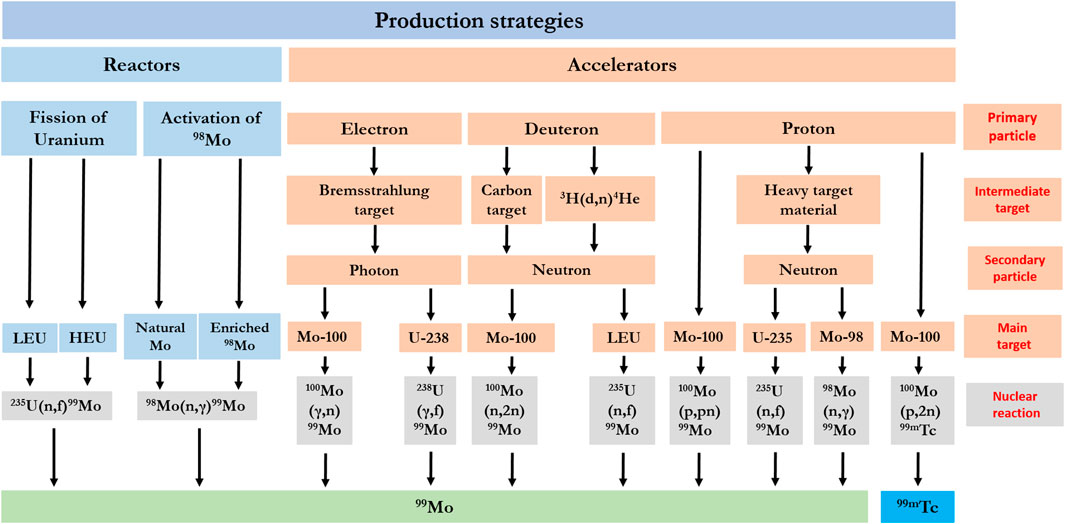
FIGURE 2. The potential production routes of 99Mo and 99mTc. Abbreviations: HEU: Highly Enriched Uranium; LEU: Low Enriched Uranium.
2.1 Reactor-based 99Mo production
The medical isotope 99Mo can be produced in nuclear reactors through two primary routes (IAEA, 1999):
2.1.1 Fission-produced 99Mo
Undoubtedly, the neutron-induced uranium fission technique is widely considered the “gold standard” for the large-scale 99Mo supply for medical applications. Actually, over 95% of all 99Mo used for the production of medical-grade 99mTc is available from the fission of uranium targets in nuclear reactors. These targets can be categorized according to the content of the fissile 235U radioisotope (IAEA, 1999, 2002, 2005; NAP, 2016):
1. Natural uranium: 235U content is about 0.72%.
2. Low Enriched Uranium (LEU): 0.72% < 235U content <20%.
3. Highly Enriched Uranium (HEU): 20% ≤ 235U content ≤90%.
4. Weapons-grade Enriched Uranium: 235U content ≥90%.
Generally, a neutron-induced fission reaction occurs when a target of a heavy fissionable element, such as 235U, is introduced into the reactor core. Hence, the 235U nucleus absorbs a thermal neutron and undergoes a fission reaction, resulting in two fission fragments. These comprise about 200 different radionuclides, including 99Mo, according to the reaction
2.1.1.1 Global supply chain of fission-produced 99Mo
The availability of a sufficient 99Mo supply involves a sequence of connected steps with high complexity. These steps can be defined as the 99Mo supply chain. In the first step, uranium targets are fabricated and tested. Then, these targets are shipped to the irradiation facilities where the production of 99Mo takes place. After that, the irradiated targets are transported to well-equipped processing facilities comprising hot cells, where the separation and purification of 99Mo from other fission products are accomplished. Afterward, the purified carrier-free 99Mo solutions are eventually used for the 99Mo/99mTc generator assembly process. Subsequently, these generators are globally distributed to provide 99mTc of high quality, ready to use for different medical needs (NAP, 2018, 2016).
Based on the fact that 99Mo decays with a relatively short half-life (T1/2 = 65.94 h), all previous steps need to be achieved as rapidly as possible to minimize radioactive decay losses. The quantity of 99Mo produced is defined with the term “six-day Curie”. This term represents how much 99Mo radioactivity remains 6 days after the end of processing. Figure 3 highlights the six-day Curie concept through the 99Mo supply chain (Paterson et al., 2015). The “six-day Curie” concept has been introduced by the manufacturers as a base for calibrating the sales price. However, it is not very suitable to express the losses of 99Mo from the end of bombardment to arrival at the end user since neither transport to the processing facility, processing time, chemical yield, and transport times to the end user are accounted for.
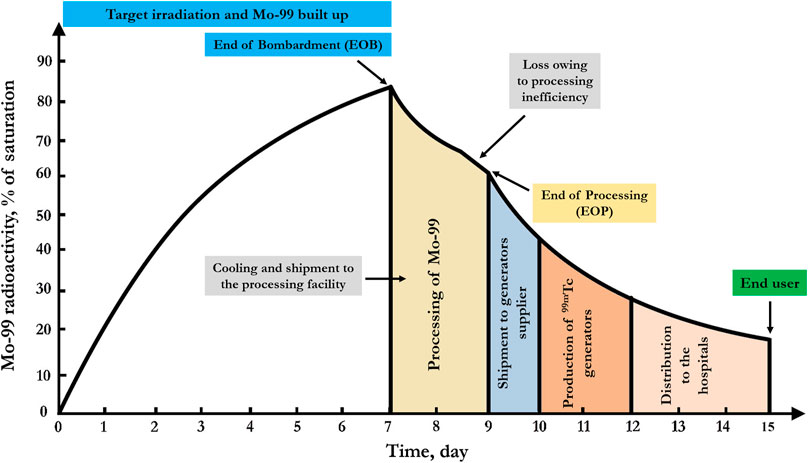
FIGURE 3. The schematic demonstration of the fission-produced 99Mo supply chain, including target irradiation, the production of 99Mo, and the 6-day Curie expression. The produced 99Mo activity from the supply chain is approximately 22% of the total irradiated activity in case of 100% recovery of 99Mo from the irradiated targets. In the same manner, if the processing efficiency is 90%, the expected remaining activity is about 17% of the total produced activity.
Close to 10,000 uranium targets (among them 8,000 targets involving HEU) are consumed annually to afford a 99Mo supply for nuclear medicine utilization. There are, at present, only four agencies that can manufacture and supply targets to the irradiation facilities. These laboratories are operated under the authority of the Atomic Energy Commission of Argentina (CNEA), Argentina; Canadian Nuclear Laboratories (CNL), Canada; Company for the Study and Production of Atomic Fuels (CERCA), France; and Nuclear Technology Products Radioisotopes (NTP), South Africa (NAP, 2018, 2016).
The neutron bombardment of the targets can be carried out in research reactors of high neutron flux, which is usually in the range of ≥ 1014 n/cm2s. Table 4 illustrates the potential irradiation reactors and their production volume (Zhuikov, 2014; NAP, 2018; NEA, 2019). The 99Mo production capability of these reactors covers more than 95% of the global medical need for 99Mo. The target irradiation process typically lasts five to 7 days until the 99Mo growth reaches 80% of the saturation yield. At this point, the overall 99Mo activity remains unchanged. That is because the quantity of 99Mo produced by the target irradiation equals the loss of 99Mo activity due to the radioactive decay (Paterson et al., 2015; NEA, 2017).
After the irradiation step, the targets are left to cool down, allowing short-lived and ultra-short-lived fission products to decay. Then, the irradiated targets are moved to the processing facilities where the recovery of 99Mo from other fission products is conducted, and a pure 99Mo-molybdate solution is prepared. Currently, there exist only five large-scale processing centers that can perform this task. Table 5 shows the five main processing facilities and their global 99Mo supply (IAEA, 2013a, 2015; NAP, 2016,; NEA, 2019).
The uranium fission method deserves careful attention owing to its capability to provide adequate amounts of 99Mo in a carrier-free form with high specific activity, which can satisfy the global medical need. However, the application of this technology faces inherent critical challenges. For instance, proliferation concerns are dramatically growing owing to the use of massive amounts of HEU targets. Nearly 80% of the global demand of 99Mo is produced using uranium targets containing up to 93% of enriched 235U. During the production steps, about 50 kg of weapons-grade HEU are usually handled, and only a very minute amount (∼3%) is utilized in the entire process (NAP, 2009; NEA, 2010; IAEA, 2015). In order to eliminate these nuclear proliferation issues, HEU targets have to be fully substituted by LEU or natural uranium targets (Wu et al., 1995; Cols et al., 2000; Conner et al., 2000). In addition, the aging fleet of the currently used irradiation facilities is also of concern. Approximately 95% of the global supply of 99Mo depends on only six reactors. With the exclusion of OPAL, all the other five reactors have been in service for more than 50 years. Lately, a number of unplanned shutdowns have taken place, and consequently, the international medical community has severely suffered from a 99Mo shortage (Gumiela, 2018). In case of any unexpected future interruption, a renewed 99Mo supply crisis cannot be ruled out and could seriously affect many countries due to the expected rise in the cost of dose per patient (NEA, 2011).
Moreover, the separation and purification of 99Mo from fission products is a sophisticated process and calls for large and complex infrastructures, professional technical skills, and well-equipped laboratories. Only few centers worldwide are well-prepared to conduct this task (Ali and Ache, 1987; NAP, 2016, 2018; NEA, 2019). Furthermore, the generation of massive quantities of toxic radioactive waste containing medium and long-lived radionuclides has to be considered. A significant number of Curies (∼50 Ci) of fission radioactive waste are generated per production of one Curie (Ci) of 99Mo (Boyd, 1987; IAEA, 1998; Tur, 2000). The difficulties mentioned above are clearly reflected in the continuing need for long-term capital investments and routine operating expenditures. As a result, the production cost of 1 Ci of 99Mo from the fission route is four times higher than that of 1 Ci of neutron-capture-produced 99Mo (Boyd, 1982a).
2.1.2 Neutron-capture-produced 99Mo
99Mo can also be produced through the neutron activation of natural or enriched 98Mo targets in the thermal flux of typical research reactors. This technology has been used for 99Mo production for more than five decades. Molybdenum has seven different naturally occurring stable isotopes, as shown in Table 6. 98Mo is the most naturally abundant molybdenum isotope with 24.13%.
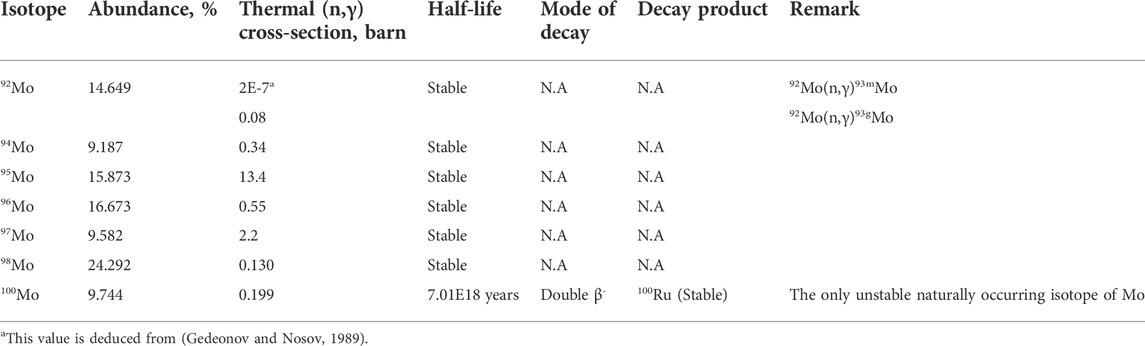
TABLE 6. The naturally occurring molybdenum isotopes (Magill et al., 2022).
During the irradiation process, the target nucleus, 98Mo, captures one thermal neutron and emits gamma rays to form 99Mo according to the nuclear reaction
Where;
S is the specific activity of the produced 99Mo in mCi/g or Bq/g,
L is the Avogadro number (6.022 × 1023),
σ is the thermal activation cross-section (0.13 barns, i.e., 0.13 × 10–24 cm2),
a is the abundance of 98Mo isotope (∼24.14%),
A is the atomic mass of molybdenum (95.94),
t is the total irradiation time,
λ is the radioactive decay constant (ln2/T1/2),
T1/2 is the half-life of the 99Mo product (T1/2 = 65.94 h), and it is in the same unit as t.
Based on Eq 1, 2, the 99Mo specific activity at the End of Bombardment (EOB) mainly depends on some essential parameters, such as the value of the neutron flux in the irradiation channel, the effective cross-section, the irradiation period, and the 98Mo content in the irradiated target (Binney and Scherpdz, 1978; Hetherington and Boyd, 1999).
The use of enriched 98Mo target material provides an adequate opportunity to enhance the specific activity. This increase is proportional to the enrichment factor. Therefore, the specific activity yield of the produced 99Mo can be improved by a factor of four using enriched targets, which have a 98Mo content ≥96%.
98Mo targets can also be defined according to the chemical form of the target material, which involves the use of MoO3 or Mo metal. The irradiated MoO3 powder can be easily dissolved compared to Mo metal. However, when molybdenum metal is used, the amount of 98Mo in the same irradiation space is higher, resulting in higher 99Mo yields per Gram material.
One of the main parameters that limit the specific activity that results from this method is the small neutron capture cross-section of 98Mo targets, which is 0.13 barns for thermal neutrons. However, this value can be increased for the same target to 6.7 barns when the irradiation is conducted in the epithermal energy region (Lavi, 1978; IAEA, 2013a). In practice, specific activities of 10–15 Ci/g Mo have been achieved (Ryabchikov et al., 2004). The produced specific activity of 99Mo from neutron irradiation of 98Mo targets is particularly low compared to 99Mo provided by the uranium fission method (see Table 3). However, the specific activity can be increased by a factor of eight if an enriched 98Mo target of a particular geometry is irradiated in a high flux reactor (Ali and Ache, 1987; Hetherington and Boyd, 1999; Hasan and Prelas, 2020).
In principle, commercial power reactors offer an adequate neutron spectrum and neutron flux for producing Low Specific Activity (LSA) 99Mo. However, power reactors are usually not equipped for the introduction of samples for irradiation during operation. This fact significantly limits the use of power reactors as irradiation sources. There are exceptions. The new EPR reactors and the older KONVOI type Pressurized Water Reactors (PWR) have a built-in, so-called aeroball system for in-situ flux measurements. During operation, 51V spheres of 1.7 mm diameter can be filled in columns in the reactor, irradiated for a short time period, and extracted again. The produced activity is then measured on a so-called measuring table (Konheiser et al., 2016; Framatome, 2019a). This system could be repurposed to introduce metallic Mo-spheres or MoO3 ceramic spheres for irradiation (Framatome, 2019b). As the columns are several meters long and there are a large number of columns, sufficient amounts of Mo can be irradiated.
Furthermore, one of the potential projects that utilize neutron-capture-produced 99Mo has been implemented by Northstar Company in the United States. Northstar uses the Missouri University Research Reactor (MURR) to irradiate both natural and enriched 98Mo targets. The estimated production capacity of this project will be about 4500 six-day Curie 99Mo per week by 2024 (NEA, 2019). Northstar is supplying LSA 99Mo solution to be used with a generator system of RadioGenix, which produces 99mTc in a computer-controlled generator system.
Clearly, the neutron activation technology eliminates proliferation concerns, as it offers a clean method to provide 99Mo without the need to handle any fissile materials. In addition, it asks for fewer technological requirements and financial budgets compared to the uranium fission approach (Boyd, 1982a). Moreover, based on the fact that only molybdenum targets are irradiated, this process involves less demanding chemical separation and purification steps as well as the generated radioactive waste level can be neglected. Furthermore, the good global distribution of reactors with high neutron flux capability could significantly support the 99Mo economy. The method is fast, and a time span of 3 days from the EOB to the arrival of the generator to the end-user is feasible. On the one hand, the 99Mo decay loss can be minimized, as the 99Mo activity can be delivered in a short period directly after production. On the other hand, a stable supply of 99Mo can be guaranteed even in case of any unplanned interruptions that may arise from the fission production line.
Despite the potential advantages of producing neutron-capture-produced 99Mo, its utility for the production of 99mTc generators faces some potential challenges, especially with conventional alumina columns. Using such materials leads to a large elution volume with a very low 99mTc radioactive concentration and a significant 99Mo breakthrough risk. Recently, extensive research efforts have been conducted for alternative strategies to overcome these problems. In this regard, the challenges and the current progress are therefore discussed in more detail in Sections 3.2, 3.3.
2.2 Accelerator-based 99Mo production
Recently, there has been a growing interest in using modern particle accelerators as a promising solution to produce 99Mo for medical applications. This approach allows the production of 99Mo without the need for HEU targets and generates relatively low amounts of radioactive waste compared to nuclear reactors (Van der Marck et al., 2010). The idea is built on the acceleration of charged particles, such as protons, deuterons, or electrons, to induce nuclear reactions in the target material. In some cases, the accelerated particles can interact with the target material and directly produce 99Mo or even 99mTc. In other cases, the reaction becomes more complex and takes place in two steps. In the first step, the primary charged particles are successfully accelerated and strike an intermediate target material to produce secondary particles, such as neutrons or high-energy photons. Then, the secondary particles interact with the main target material to produce 99Mo (NEA, 2010). The choice of one of these techniques mainly depends on the post-irradiation 99Mo production capability. The primary accelerator-based 99Mo − or direct 99mTc production approaches are summarized in Table 7. In Table 7, the irradiation yields were calculated under modern-day, realistic assumptions concerning beam intensities using publicly available nuclear codes. For photonuclear reactions, it was assumed that the electron beam is delivered by an IBA TT-300 HE rhodotron (IBA, 2018) impinging on a high-power converter target (Türler et al., 2021). The photon spectrum and the yield of 99Mo were calculated by Vagheian (Vagheian, 2022). For proton and deuteron-induced reactions, it was assumed that the beam is being delivered by a IBA Cyclone IKON-1000 cyclotron as a typical representative of accelerators in the 30 MeV energy range (IBA, 2022). It was assumed that a solid target station could accept a maximum proton beam current of 400 μA (i.e., Nirta High Power Solid target (90–400 μA)), since molybdenum is a very refractive element. For the calculations, the web-based “Medical Isotope Browser” was employed (see the footnote in Table 7). As can be seen from Table 7, the production of 99Mo with an electron accelerator from 100Mo appears to be most promising due to the large activities that can be produced. Proton irradiations of 100Mo yield significantly less yield of 99Mo, while producing more undesired by-products. The direct production of 99mTc from 100Mo is a viable option but significantly reduces the distribution radius of the product (see Section 3.1). In the following, the accelerator-based production of 99Mo is discussed in more detail.
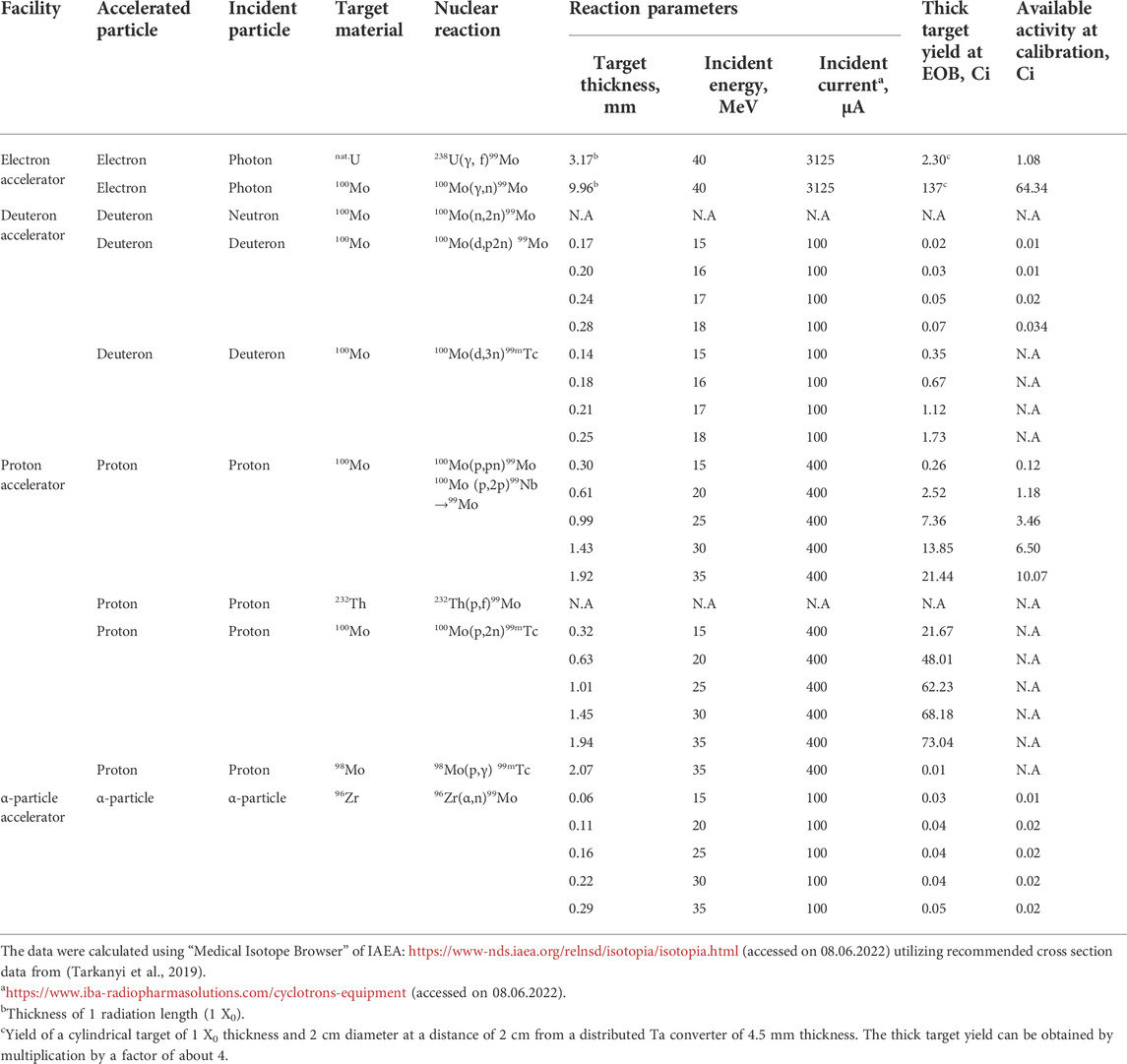
TABLE 7. The main accelerator-based 99Mo and 99mTc production routes and their production capabilities per day.
2.2.1 Photonuclear reactions
With electron accelerators, a high-intensity electron beam is allowed to impinge on a dense target material to produce Bremsstrahlung photons. These photons can be used to produce 99Mo by two main strategies:
1- Photon-induced fission (photofission) reaction.
2- Photon-induced nuclear (photonuclear) reaction.
In the photo-fission method, high specific activity 99Mo can be obtained from the reaction
The photonuclear transmutation reaction offers a promising strategy for 99Mo production according to the reaction
2.2.2 14.1 MeV neutron-induced reactions
99Mo can also be produced through the bombardment of a 100Mo target with high energy neutrons according to the nuclear reaction 100Mo(n,2n)99Mo. Here, the 14.1 MeV neutrons are generated in the reaction 3H(d,n)4He. The reaction cross-section for the reaction 100Mo(n,2n)99Mo is about 1.5 barn at the neutron energy level of 14.1 MeV, which is ten times higher than the cross-section of the 98Mo(n,γ)99Mo reaction in the thermal region. In addition, a long irradiation period of more than 8 days and a high-intensity neutron flux ≥ 1013 n/cm2s is required (Nagai and Hatsukawa, 2009). Even so, the production of low specific 99Mo with large amounts of inactive carrier is still present even with 100Mo targets of 100% enrichment. Under the conditions mentioned above, the specific activity of the produced 99Mo was estimated at about 2 Ci/g (79 GBq/g).
In another approach, 14 MeV neutrons from a D-T generator are directed at a low enriched 235U salt solution, where 99Mo is produced as a fission product and periodically separated. This approach is being commercially exploited by Shine Medical Technologies Inc. (Wisconsin, United States) (Ruth, 2020).
2.2.3 Spallation neutron-induced reactions
Similar to the concept of neutron-induced fission or capture reactions in a nuclear reactor, 99Mo can be produced with the use of an Accelerator-Driven Subcritical Reactor (ADSR) through 235U(n,f)99Mo and 98Mo(n,γ)99Mo reactions (Abderrahim et al., 2010; Bertsche, 2010; NEA, 2010; Owen et al., 2014; Syarip et al., 2018). However, the used neutrons have a different origin. High-intensity neutron flux can be generated following the bombardment of a heavy mass target material with an accelerated proton beam, such as lead, uranium, tungsten, or tantalum. This reaction produces fast neutrons with a kinetic energy level of more than 1 MeV up to almost the beam energy of the proton beam, which then is slowed down to the epithermal energy level with the help of a moderator. These conditions offer a unique opportunity to obtain a reasonable 99Mo yield with a relatively high specific activity using the 98Mo(n,γ)99Mo option. Uranium targets have to undergo routine chemical processing to provide purified
2.2.4 Other production methods
Many research groups have proposed other possibilities through proton- or deuteron-induced 99Mo production (Beaver and Hupf, 1971; Lagunas-Solar et al., 1991; IAEA, 1999; Hermanne et al., 2007). For example, the
3 99mTc production and supply strategies: Challenges and progress
3.1 Cyclotron-produced 99mTc
The severe 99Mo production shortage during the past few years, along with the widespread concerns of unexpected future 99Mo supply inadequacy, have generated a growing need for establishing an independent sustainable supply of 99mTc. Consequently, many research projects have been established to promote the direct production of 99mTc exploiting cyclotron production. The cyclotron-based 99mTc production can provide an independent option for domestic utilization (IAEA, 1999; Ruth, 2009, 2014; Gagnon et al., 2011; Hanemaayer et al., 2014; Owen et al., 2014; Metello, 2015; Boschi et al., 2017; Martini et al., 2018).
Several approaches have been proposed for direct 99mTc production, such as 100Mo(p,2n)99mTc, 98Mo(p,γ)99mTc, 100Mo(d,3n)99mTc, 98Mo(d,n)99mTc, 97Mo(d,γ)99mTc, and 96Mo(α,p)99mTc. It is pertinent to point out that the proton-induced reactions produce the highest production yields compared to deuteron or alpha particle-induced reactions. In addition, accelerators with high beam intensity of deuterons or alpha particles are currently rare. For these reasons, they received less attention (Lagunas-Solar et al., 1991; IAEA, 1999, 2017; Takács et al., 2003; Guerin et al., 2010; Owen et al., 2014; Stolarz et al., 2015).
The direct production of the 99mTc using energetic proton beams involves two reactions;
The most commonly used molybdenum target material is the Mo metal. In addition, the utilization of MoO3 and Mo2C is also possible (Richards et al., 2013). The fabrication of the molybdenum targets can be developed with the help of several technical advances. These approaches involve preparing foils, pressing and thermal sintering of molybdenum powder, vacuum sputtering technology, laser beam pressed molybdenum powder method, electrochemical plating technique, and electrophoretic deposition followed by thermal sintering process (Richards et al., 2013; Hanemaayer et al., 2014; Stolarz et al., 2015; Hou et al., 2016; Martini et al., 2018). The target purity plays a crucial role and greatly affects the quality of the final 99mTc product. The contribution of other molybdenum isotopes with 100Mo in the target material leads to producing different impurities such as Tc, Nb, Ru, and Zr (Beaver and Hupf, 1971; Lagunas-Solar et al., 1991; IAEA, 1999; Guerin et al., 2010; Owen et al., 2014; Hou et al., 2016). To reduce the impurity level, the isotopic abundance of 100Mo targets must not be less than 99.5%. Some of the produced impurities can be removed during the separation and purification processes. However, the different Tc isotopes have the same chemical behavior as 99mTc. Therefore, the separation of these isotopes poses considerable laborious challenges, especially with current conventional separation technology. The Tc isotopes can be classified into two groups. The first group involves the long-lived radioisotopes, such as 99gTc (T1/2 = 2.11×105 y), 98Tc (T1/2 = 4.2×106 y), and 97gTc (T1/2 = 4.21×106 y). These radioisotopes decrease the final specific activity of the product, but they may not be involved in an additional dose for the patient (Beaver and Hupf, 1971; Lagunas-Solar et al., 1991). On the other hand, the second group contains the shorter half-lives radioisotopes, such as 93mTc (T1/2 = 43.5 min), 93gTc (T1/2 = 2.75 h), 94mTc (T1/2 = 52 min), 94gTc (T1/2 = 4.9 h), 95mTc (T1/2 = 61 d), 95gTc (T1/2 = 20 h), 96mTc (T1/2 = 51.5 min), 96gTc (T1/2 = 4.28 h), and 100Tc (T1/2 = 15.8 s). This group not only substantially drops the specific activity of the final product, but also delivers up to 30% extra unjustified dose to the patient (Beaver and Hupf, 1971; Lagunas-Solar et al., 1991; IAEA, 1999, 2017; Hou et al., 2012; Lededa et al., 2012; Owen et al., 2014; Meléndez-Alafort et al., 2019). Accordingly, the optimization of irradiation parameters offers another opportunity to minimize the produced technetium impurities. These parameters include the bombarding beam energy, irradiation time, and incident beam intensity.
As a generic rule, the higher the proton energy and the longer irradiation time, the higher the production yield. However, these two factors have to be well-controlled to reduce the co-production of undesirable impurities, resulting in a high level of specific activity product obtained. The threshold proton energy for the reactions
In order to achieve a high production yield, the beam power intensity should be set to the highest possible value. This beam current crucially depends on the target material, the applied incident beam angle, and the cooling system during the irradiation process (Beaver and Hupf, 1971; IAEA, 1999). It is worth mentioning that molybdenum targets have preferable chemical and physical properties reflected by their high melting point and excellent thermal conductivity. Therefore, they can withstand high beam currents. The target cooling process is also influenced by the thermal conductivity of the target material and the temperature of the used cooling item. Effective target cooling can be achieved by applying helium flow on the front side and water-cooling of the supporting material of the target during the irradiation process. Some research studies proposed the use of liquid nitrogen instead of water-cooling for more effective target cooling (IAEA, 2017, 1999).
After completing the irradiation process, the target is left to cool down to reduce the incorporation of short-lived impurities in the final product, followed by chemical processing. This step is crucial and should be achieved in the shortest possible time to minimize 99mTc decay loss (Qaim, 2012). The post-production target processing can be achieved in two steps: target dissolution and chemical separation and purification. The efficient dissolution of the molybdenum targets is paramount for an effective 99mTc recovery. The target dissolution can be performed either by chemical or electrochemical procedures. The chemical dissolution can be performed with the help of concentrated sodium hydroxide, hydrogen peroxide, and heating. In the case of foil targets, concentrated acids can also be used. The electrochemical dissolution system consists of the molybdenum target as an anode, a platinum layer as a cathode, and potassium hydroxide, which works as an electrolyte. Electrochemical methods may involve violent reactions and need double the time to achieve the same task compared to chemical methods (Gumiela, 2018; IAEA, 2017, 1999).
Many potential techniques have been implemented for the 99mTc recovery from dissolved 100Mo targets. Because of the short half-live of 99mTc, a fast separation reaction is a key to selecting the extraction method. The most commonly used methods include solvent extraction, which can be carried out with the use of Methyl Ethyl Ketone (MEK) or Cetyl Trimethyl Ammonium Bromide (CTAB) (Martini et al., 2018). Column chromatographic separation methods involve the use of ion exchanger sorbent materials with high radiation resistance, such as Dowex-1 or Polyethylene Glycol (PEG). Some other techniques have also been proposed, such as dry thermo-chromatographic extraction and the chemical precipitation of the molybdenum as a hetero-poly acid salt. The former generates small quantities of waste, and the latter could provide a reasonable separation yield. Nevertheless, both strategies are not widely utilized (IAEA, 1999; Boschi et al., 2017; Gumiela, 2018).
Based on these discussions and as mentioned earlier, the cyclotron-produced 99mTc technology has been extensively studied in recent years, and some success has been achieved. Therefore, this method can be regarded as a backup solution for 99mTc supply to deal with emergencies. However, its potential is inadequate to replace the current 99Mo/99mTc generator production completely; as the following critical issues still call for convincing answers:
• Only for regional consumption: The short half-life of 99mTc (T1/2 = 6.01 h) restricts its wide-scale distribution. As a result, the delivery advantage is only limited to hospitals located near the production sites. Therefore, the implementation of this supply strategy necessarily asks for an even geographic distribution of cyclotrons.
• High cost per dose: The end-user should receive the required dose at a reasonable price, and the dose price should be competitive with that delivered from a 99Mo/99mTc generator. The use of enriched 100Mo targets to ensure the production of high-quality 99mTc increases the production cost. In addition, the recycling process of the used target material adds an extra production expenditure. Furthermore, the long post-irradiation processing step and the transportation requirements are reflected by the loss of 99mTc radioactivity. All these parameters can be translated into a significant cost factor.
• A low specific activity product delivers extra radiation dose to the patients: The utilization of highly enriched 100Mo targets is vital to produce 99mTc with high specific activity suitable for imaging applications. A 100% isotopic purity of 100Mo targets is hard to achieve, and small quantities of other stable Mo isotopes are constantly incorporated into the target material. Consequently, considerable amounts of different technetium isotopes are always co-produced. On the one hand, these impurities reduce the specific activity of the produced 99mTc product, which significantly affects the labeling efficacy of 99mTc-radiopharmaceutical kits. On the other hand, it may contribute to additional radiation exposure to the patient (Hou et al., 2012; Lededa et al., 2012; Selivanova et al., 2015; Meléndez-Alafort et al., 2019).
• Uncertainty of long-term supply capability: The direct production of 99mTc has been proposed since 1971, and it has not been thoughtfully implemented as a long-term supply strategy. Many recent efforts have focused on establishing a large-scale 99mTc supply on a daily routine basis. However, several problems need to be solved. These challenges can be briefly summarized in the need for a sufficient daily availability of patient doses at a reasonable price. Additionally, this strategy continues asking for a realistic supply plan design that can deal with the production difficulties and find innovative logistic solutions to meet the rapid product delivery requirements.
3.2 99Mo/99mTc generators
Despite the fast global spread of diagnostic scans using 99mTc-radiopharmaceuticals, the 99mTc availability at the hospitals and nuclear pharmacies continues to be a potential challenge. These challenges include the production difficulties, notable price, and remarkable radioactivity loss due to frequent deliveries to the usage sites. Accordingly, its effective utilization is only restricted to sites with a well and close connection to the production centers (Sakr et al., 2017). In the light of this perceived need, different 99Mo/99mTc generator strategies have been proposed and developed. 99mTc generators are considered the most widely used strategy to ensure the ease of 99mTc availability in a cost-efficient manner. In other words, it allows conducting a variety of 99mTc scans independently of on-site production requirements (Knapp and Mirzadeh, 1994; Chakravarty et al., 2012a, 2012b; Knapp and Baum, 2012; Chakravarty and Dash, 2013; Sakr et al., 2017).
3.2.1 99Mo/99mTc radioactive equilibrium in the generator system
The 99Mo/99mTc generator technology has been developed to offer an easy and repetitive on-site supply of 99mTc at desirable time intervals. Historically, 99Mo/99mTc generators were named “cows”, from which a fresh 99mTc radioactivity can be periodically “milked” or extracted. Based on the fact that 99Mo and 99mTc are radioisotopes of two different elements, they have unique differences in their chemical and physical properties, and the generator system can supply 99mTc with high specific activity, i.e., Non-Carrier-Added grade (NCA) and with high radionuclidic purity acceptable for radiopharmaceutical preparations (Lebowitz and Richards, 1974; Molinski, 1982; IAEA, 2013b).
99Mo/99mTc generators are efficient radiochemical separation tools, by which a convincingly purified yield of the daughter, 99mTc, can be effectively extracted from the decay of the parent 99Mo. In other words, the 99Mo/99mTc generator concept is based on housing 99Mo to decay. Then, a complete separation of the generated 99mTc can be performed without any disturbance of 99Mo. This separation process is managed through the 99Mo-decay/99mTc-growth concept (Lebowitz and Richards, 1974; Molinski, 1982; Guillaume and Brihaye, 1986; Saha, 1992; Osso and Knapp, 2011; Knapp and Baum, 2012; Chakravarty and Dash, 2013; Gumiela, 2018).
Generally, the generator concept is based on the parent/daughter relationship, by which the parent, with a longer half-life, decays to yield its shorter-lived daughter. Hence, a sort of radioactive equilibrium is established. This state is called secular or transient equilibrium, depending on the ratio between the two physical half-lives of the parent and its daughter. The secular equilibrium arises when the parent’s half-life is 100 times longer than the half-life of the daughter. Whereas the transient equilibrium, as in the 99Mo/99mTc generator system, is recognized when the parent’s half-life is ten-fold longer than that of the daughter (Sakr et al., 2017). Figure 4 illustrates the transient equilibrium between 99Mo and 99mTc.
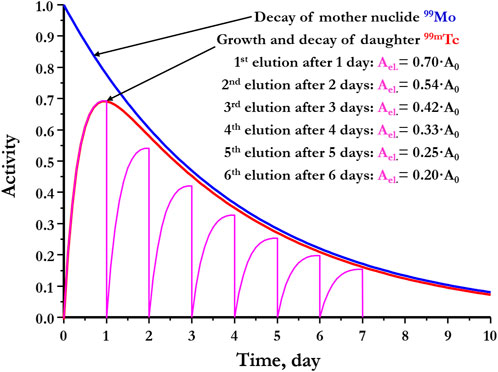
FIGURE 4. Transient radioactive equilibrium in 99Mo/99mTc generator system. Ael: Eluted 99mTc activity. A0: 99Mo activity at calibration.
The equilibrium between 99Mo and 99mTc is reached after approximately four elapsed half-lives of 99mTc. During the 99mTc ingrowth, its production is remarkably faster than its decay. After the equilibrium is established, the 99mTc growth and decay rates are equal. At this point, 99mTc apparently decays with the longer half-live of 99Mo. This advantage considerably reduces the 99mTc radioactivity loss during shipment to remote users. However, it decays with its physical half-life directly after its elution from the generator. Directly after the elution, the generator starts the same process from the beginning, as 99Mo decays to give rise to new fresh 99mTc. Accordingly, 99mTc begins to be re-built-up again (Lebowitz and Richards, 1974; IAEA, 2013b). The growth, extraction, and regrowth of 99mTc are non-stop procedures. Consequently, the elution step can be repeated as long as sufficient 99Mo radioactivity is available to conduct the required applications. Therefore, 99mTc can be eluted from the generator at periodical elution batches.
The mathematical correlation that describes the 99mTc growth from the decay of 99Mo can be explained using the Batemann equations, as follows (Hidalgo et al., 1967; Lebowitz and Richards, 1974; Lamson et al., 1976; Ekelman and Coursey, 1982; IAEA, 2013b):
The decay of 99Mo can be expressed as
Where:
λ: is the 99Mo decay constant,
The production of 99mTc is the same as the decay of 99Mo. Considering that 99mTc decays at a rate of. Hence, the 99mTc growth rate can be represented by:
Accordingly, the Eq 6 can be reformulated as:
Taking into account that only about 87.5% of 99Mo decays to 99mTc (IAEA, 2017).
In order to give units of disintegration per second (A) rather than atoms (N), both sides of Eq 8 are multiplied by
At the time (t), when the radioactive equilibrium between 99Mo and 99mTc activities is reached, the growth rate of 99mTc is equal to its decay rate:
Hence, the maximum activity of the 99mTc, which is present at the time (t), can be calculated from the following equation:
Clearly, the radioactive relationship between 99Mo and 99mTc offers an excellent opportunity to introduce an ideal generator system, by which the separation of the short-lived 99mTc at favorable time intervals can easily be achieved. The eluted radioactivity can be quantified using equation number (6). Nevertheless, it is significantly influenced by the elution process efficiency. Hence, the maximum 99mTc radioactivity can be eluted on a daily basis, which is one of the potential advantages of the generator system.
3.2.2 Criteria of a clinical 99Mo/99mTc generator
The effective 99Mo/99mTc generator system should fulfill the following characteristics (Lebowitz and Richards, 1974; Sakr et al., 2017; IAEA, 2018):
• Use simple, safe, and user-friendly operational protocols that avoid any violent chemical reactions and support a feasible implementation at hospitals and nuclear medicine centers.
• Assure rapid radiochemical separation to minimize the 99mTc radioactivity decay loss.
• Provide the highest possible elution yield with a reproducible separation efficiency over the generator operation lifetime.
• Capable of sustainable elutions with a negligible amount of radioactive waste.
• Provide a carrier-free 99mTc radioactivity with high specific activity without any need for purification processes.
• Supply sterile, isotonic, and pyrogen-free 99mTc radioactive solution at any elution cycle without any further need for chemical treatment.
• Produce 99mTc with high radioactive concentration adequate for the radiopharmaceuticals synthesis without any need for post-elution concentration steps.
• Supply the 99mTc radioactivity with high chemical, radiochemical, and radionuclidic purities. The chemical purity expresses how much of the produced 99mTc is free from inactive impurities that arise from the target material and/or the used chemicals. The radiochemical purity is the percentage of 99mTc radioactivity present in the pertechnetate chemical form, 99mTcO4-. The radionuclidic purity represents the percentage of the 99mTc radioactivity to the total radioactivity of its solution.
• Minimize the personnel involved during the elution process to reduce the radiation exposure dose.
• Involve the use of chemical solutions and materials with high radiation stabilities to avoid any possible contamination to 99mTc radioactivity.
3.2.3 Production technologies of 99Mo/99mTc generators
Historically, the idea of a 99Mo/99mTc generator system was first proposed in the middle of the 1950S during the development of 132Te/132I generators at Brookhaven National Laboratory (BNL). In this experiment, the 99mTc was detected as a trace impurity in the 132I eluted solution. Afterward, it was understood that this 99mTc was produced from its parent, 99Mo, which took the same separation path of 132Te throughout the chemical processing of the fission products. This unique parent-daughter chemistry between 99Mo and 99mTc has encouraged the fabrication of the first 99Mo/99mTc generator (Richards et al., 1982; Anderson et al., 2019). In 1957, the first 99Mo/99mTc generator was produced at BNL (Tucker et al., 1958; Molinski, 1982; Richards et al., 1982). Then, in early 1960, the first 99Mo/99mTc generator was used for clinical research at Brookhaven medical department, which paved the way for the first utilization of 99Mo/99mTc generators in clinical investigations at Argonne Cancer Research Hospital in 1961 (Richards, 1965; Lebowitz and Richards, 1974; Atkins, 1975). Later on, through the following seven decades, the idea was developed, and several 99Mo/99mTc generator structures were established based on the different physical and chemical behaviors of 99Mo and 99mTc radionuclides (Allen, 1965; Boyd, 1987; IAEA, 1995, 2013b; Maoliang, 1997; Osso and Knapp, 2011; Knapp and Baum, 2012; Mostafa et al., 2016).
The careful selection of the radiochemical separation strategy is considered the cornerstone for the fundamental development of 99mTc generators. In fact, this selection focuses explicitly on simplifying the extraction process and avoiding any technical complexities for the users. In addition, it supplies the possible maximum 99mTc yield with the minimum quantity of radioactive waste. Furthermore, it supports a rapid 99mTc elution with high-performance quality, which consequently; can be easily adopted as a mature commercial technology. In the light of this context, the following subsidiary sections underline the critical features of potential 99Mo/99mTc generators production strategies. Table 8 highlights a comparison between the potential development technologies of 99mTc radioisotope generators.
3.2.3.1 Sublimation generators
Sublimation is a physical phenomenon, which can be defined as the direct conversion of solids into a gas without moving through the liquid state. Perrier and Segrè were the first who described the possibility of a 99mTc thermal extraction from 99Mo based on the difference in the sublimation temperatures or volatilities of their oxides (Perrier and Segrè, 1937). Tc2O7 and MoO3 become volatile at temperatures of 550 and 1,000°C, respectively (Perrier and Segrè, 1937; Childs, 2017). The separation steps were reported through several research studies. The irradiated 99MoO3 target is carefully placed inside a muffle furnace, in which a flow of oxygen stream is allowed to pass through. Then, the temperature is gradually elevated to about 800 °C. On heating, Tc2O7 is formed, which sublimates at 550°C. After that, the volatile Tc2O7 vapors are carried by the oxygen stream and trapped using a cooled surface. Finally, they are allowed to be completely dissolved in water or saline solution to obtain 99mTcO4− (Hallaba and El-Asrag, 1975; Molinski, 1982; Zsinka, 1987; Miller, 1995; Christian et al., 2000; IAEA, 2013b).
This generator strategy generates a small volume of radioactive waste. In addition, it provides high specific activity 99mTc even if 99Mo of low specific activity origin is available. However, attempts to implement this technique in nuclear medicine departments as a clinical-based generator system suffered from several critical constraints. For instance, it includes the manipulation of bulk, complex, and fragile equipment, which needs a meticulous operational methodology and an advanced level of precautions and safety standards. Moreover, the 99mTc separation yield is very low. Furthermore, it uses high heating temperatures, which may lead to a serious radiation contamination risk in case of any operational failure. Accordingly, these potential drawbacks discouraged further development plans in this direction.
3.2.3.2 Solvent extraction generators
The solvent extraction-based generator is a multi-step separation technique by which 99mTcO4- can be recovered from an aqueous solution that contains the 99Mo/99mTc mixture by the extraction into an organic solvent (IAEA, 2013b). The separation of 99mTc from its parent with the help of organic extractants was originally demonstrated by Gerlit in 1956 (Gerlit, 1956; Anders, 1960; Lebowitz and Richards, 1974; Molinski, 1982).
The separation process involves the dissolution of the irradiated 99Mo target in an alkaline solution. Then, an organic solvent is added, which is followed by multiple-extraction cycles. Each cycle includes a vigorous shaking of the two immiscible phases to allow the 99mTcO4− to migrate from the original aqueous solution to the organic extractant phase leaving its parent behind. After that, the extractant is segregated, washed several times, and thermally evaporated. Finally, the residual precipitate is redissolved in a saline solution. The process efficiency strongly depends on the selective capability of the extractant solvent for the 99Mo/99mTc pair. In other words, the process relies on the relative distribution or solubility ratio of the two radionuclides between the extractor and the original aqueous solution (Chattopadhyay et al., 2010, 2014; IAEA, 2013b; Mitra et al., 2020).
Therefore, the ideal extractant should show a considerable selective affinity towards 99mTc and neglected distribution selectivity for 99Mo. The first used organic extractant to achieve the extraction process was Methyl Ethyl Ketone (MEK). After that, various organic solvents were reported (Allen, 1965; Anwar et al., 1968; Baker, 1971; Tachimori et al., 1971; Iqbal and Ejaz, 1974; Noronha, 1986; Bhatia and Turel, 1989; Bhatia and Turel, 1989; Minh and Lengyel, 1989; Taskaev et al., 1995; Chen and Tomasberger, 2001; Zykov et al., 2001; Skuridin and Chibisov, 2010). Even so, MEK is still considered the predominant extractant of choice because of its highly selective behavior for 99mTcO4-, which permits the production of 99mTc with high specific activity (IAEA 2017).
Practically, the solvent extraction generators are associated with a number of serious drawbacks that impede their wide-scale utilization; it is a complicated multi-step process with low extraction efficiency and requires a long separation time, resulting in the loss of 99mTc radioactivity. Moreover, the separation step consumes large volumes of the organic extractant, creating significant radioactive waste. In addition, a strong possibility of fire hazards that result from the flammable nature of MEK. Furthermore, the poor radiation stability of organic solvents may heighten the contamination risk of the final 99mTc product with some organic impurities.
3.2.3.3 Electrochemical generators
The electrochemical-based 99mTc generator can be introduced as a separation technique built on an oxidation-reduction reaction. This reaction is mainly governed by the difference in the formal reduction potential of the 99Mo/99mTc pair. Herein, the generator system consists of an electrochemical cell, in which the 99mTc radionuclide can be separated from the 99Mo/99mTc equilibrium mixture by a reduction reaction on the surface of an electrode under the effect of an external voltage in accordance with its electrochemical reduction potential, i.e., electrochemical deposition. The 99Mo/99mTc pair exhibits different electrochemical reduction potentials, and their electrochemical reduction reactions can be illustrated as follows (Chakravarty et al., 2012b; 2012c, 2011, and 2010a):
It can be observed that the 99mTcO4− species have a higher electrochemical reduction potential than the 99MoO42- ions, which offers a positive advantage in facilitating the separation step.
The process starts with the dissolution of the irradiated 99Mo target in a strong alkaline electrolyte (pH ∼ 13). Then, a constant potential is applied and adjusted for a certain period to permit the 99mTcO4- species to be electro-deposited on the surface of the cathode electrode. After that, this cathode is removed from the working cell and transferred to a new cell, where the deposited 99mTc can be stripped back and redissolved in a saline solution by applying a high reverse potential (reverse electrode polarity) for a short period. By this technique, 99mTc can be oxidized back to the solution in the form of 99mTcO4-. Eventually, 99mTc eluate is allowed to pass through an alumina column to minimize the 99Mo contamination level.
In order to achieve a satisfactory 99mTc separation yield, some parameters have to be well-optimized during the electrolysis process. For instance, careful attention should be devoted to adjusting the effective potential, proper selection of suitable electrodes and electrolysis medium candidates, and successful control of the electrolyte pH and separation time.
The applied potential is the crucial factor for a successful extraction. Generally, the electrochemical separation can be performed by either applying a constant current (galvanostatic) or a constant potential (potentiostatic) conditions. However, the potentiostatic condition offers favorable 99mTc deposition and, in the meantime, limits the precipitation of 99Mo and/or any other contaminants. The electrolysis potential should be adjusted to be more negative than the formal reduction potential of 99mTcO4- species and less negative than the electrochemical reduction potential of 99MoO42- ions. Therefore, the optimum reduction potential for the 99mTc generator system should be in the region of:
Additionally, it needs to be fully compatible with the used electrolyte to avoid any possibility of chemical degradation during the separation process. Several classes of electrolytes of organic or aqueous origin can be used. The aqueous electrolytes show higher radiation stability during the course of the electrolysis compared to organic liquids, which results in fewer chemical impurities associated with the produced 99mTc radioactive solution. However, their use is usually associated with the evolution of hydrogen gas, which lowers the pH of the electrolyte medium and may decrease the separation performance. For the working electrodes, they need to be chemically inert with considerable electrochemical and radiation resistance. The temperature of the medium should be adjusted sufficiently below the electrolyte boiling point to avoid its rapid evaporation, which results in unacceptable 99mTc solution purity. The reaction time should be established to achieve the highest possible 99mTc yield in the shortest period to minimize 99mTc radioactivity loss (Chakravarty et al., 2012b; 2012c, 2011; 2010a; 2010b).
Based on this method, Chakravarty et al., 2010a, reported the production of 99mTc from a 99Mo/99mTc generator. The daughter extraction was performed in an electrochemical cell by applying a constant potential of 5 V in sodium hydroxide electrolyte (pH ∼ 13). The electrolysis process lasted for approximately 1 hour. Hence, the 99mTc selectively accumulated on the platinum electrode (cathode). The 99mTc deposited was recovered in a 0.9% saline solution. Then, it was purified with the use of an alumina column (Chakravarty et al., 2010a).
The prospect of 99Mo/99mTc electrochemical-based generators provide an adequate 99mTc yield in a non-carrier added form with a satisfactory purity level for radiopharmaceutical applications. In addition, their production capacity neither relies on the amount of the used sorbent, nor the extractant volume. Nevertheless, this production strategy is implicated with some opposing weaknesses that preclude its practical medical use. For example, the electrolysis step is accompanied by the evolution of oxygen and hydrogen gases, which may pose an explosion hazard. In addition, the oxygen gas may inhibit the reduction of 99mTcO4- species, which drastically decreases the separation yield. Moreover, the working electrodes require tedious cleaning processes directly after each separation cycle. Furthermore, it is a relatively time-consuming production technique, including different consecutive separation and purification steps, which consequently result in a marked loss of 99mTc radioactivity. Finally, the procedure needs high shielding requirements to reduce the exposure dose to the working personnel. For these reasons, this strategy strongly requires a very rigorous operating regime with a high degree of safety and precautionary measures and well-trained operators with high technical awareness of electro- and radiochemistry fundamentals.
3.2.3.4 Supported Liquid Membrane generators
This generator system can be introduced as a modification or an updated version of the solvent extraction method (Dhami et al., 2007; Dutta and Mohapatra, 2013; Chang, 2016; Davarkhah et al., 2018). It involves the separation of 99mTc species from 99Mo with the use of a membrane as a semi-stationary phase and two other mobile phases that are located on both sides. These three compartments can be described as follows (Sastre et al., 1998; Chaudry, 2000; Yassine, 2000):
1. The primary aqueous solution (the feeding phase): It consists of the 99Mo/99mTc mixture.
2. The stationary porous membrane: This membrane is of hydrophobic nature and serves as a barrier between the other two liquids. It is incorporated with an organic liquid of a high selective tendency for 99mTc and delivers the 99mTcO4- ions from the feed solution to the receiving liquid. Meanwhile, it restricts the flow of 99Mo species.
3. The eluate receiving solution (the stripping phase): this partition contains the recovered 99mTc.
The extraction process is performed in three simultaneous steps. In the first step, the 99mTc species are selectively collected from the feed mixture by the extractant. Then, they travel through the membrane to the other side, where they are eventually exchanged with another species from the clean receiving solution. This journey of 99mTc from the primary solution to the membrane and from the membrane to the receiving solution is only controlled by separation reaction kinetics without the help of any external driving force. Therefore, it is only attributed to the concentration difference on both sides of the membrane.
Many different supported liquid membrane frameworks have been studied to separate 99mTc on the laboratory scale (Sastre et al., 1998; Gyves and Rodríguez, 1999; Chaudry, 2000; Yassine, 2000; Chen et al., 2001). Nonetheless, a number of inherent practical difficulties still ask for more research efforts. For instance, it is a time-consuming strategy and involves the loss of the majority of 99mTc radioactivity attributable to the remarkably slow extraction kinetics. In addition, it supplies unsatisfactory 99mTc separation yield with high 99Mo breakthrough and organic chemical impurities due to the low radiation resistance of the organic liquids and limited life-course of the used membrane. Furthermore, it generates substantial amounts of radioactive waste.
3.2.3.5 Column chromatographic generators
Several 99Mo/99mTc generator production strategies have been explored and developed over the last few decades. Nevertheless, the column chromatography-based generator type has attracted considerable interest as a reliable source to supply 99mTc ready for nuclear medicine investigations. As a result, the chromatographic column generator is considered the most predominant choice for the hospitals and nuclear medicine departments owing to many valuable benefits, including (Nawar and Türler, 2019):
• One-step process: The 99mTc radioactivity can be extracted in only one step, effectively minimizing the radioactive decay losses.
• Simple to use: The operation is feasible, user-friendly, and does not involve any operational difficulties or chemical and radiation hazards.
• Safe to use: The small generator size facilitates its shielding. Thereby, it can be handled and eluted safely with a minimum radiation dose to the operators.
• Rapid elution process: The generator offers a short elution time due to the fast separation kinetics of the daughter.
• High elution efficiency: The generator system provides a high separation yield of 99mTc from its parent mixture with a favorable carrier-free grade.
• High eluate purity: The 99mTc solution can be collected free from 99Mo with high chemical, radiochemical, and radionuclidic purity (without any need for post-elution purification steps).
• Small elution volume: The generator is designed to give the entire 99mTc radioactivity yield in a very minute quantity with a high radioactive concentration adequate for immediate use, which avoids any further concentration challenges.
• Reproducible 99mTc supply: The generator is capable of frequent multiple elutions with sustainable and stable supply in terms of 99mTc yield and purity over its lifetime.
• Marginal generation of radioactive waste: It is reflected in a clean working environment and the absence of any contamination risk.
In generic terms, this generator system consists of two phases, i.e., a stationary phase (the sorbent material) and an external liquid phase (the eluent solution). The central concept is built on the retention of 99Mo radionuclide on a column incorporated with proper sorbent material. Then, the 99Mo decays to yield 99mTc, followed by the elution of the generated daughter with a suitable isotonic saline solution. The separation of the 99mTc species is mainly based on the difference between their low retention affinity on the column material and their high solubility in the eluent solution. The parent 99Mo exists in the molybdate form,
The formed species with six negative charges offer favorable conditions for interacting with the positively charged sorbent surface. After that, the polymoybdate
The generator performance greatly depends on the sorption–elution kinetics and the degree of the sorbent selectivity for the parent Mo (containing 99Mo). Therefore, the careful selection of the column material is the prime factor for the success of this generator system. Favorable sorbent material should have a considerable selectivity for Mo and, at the same time, a very limited or no selective capability for 99mTc. Based on this parameter, an optimum separation environment can be established between the two radionuclides, and thereby, the 99mTc can be extracted in high purity. Furthermore, fast elution kinetics of the daughter can be obtained.
Moreover, the sorbent material should show a high sorption capacity for Mo (containing 99Mo). The sorption capacity indicates the quantity of Mo the column material can retain. It mainly depends on the number of available active sites on the sorbent surface for the parent element. Higher sorption capacity is directly reflected by a lesser amount of column material needed to achieve the desirable radioactivity level. Consequently, it is translated to a higher radioactive concentration of the eluted 99mTc solution.
Furthermore, the column material needs to have a high radiation resistance and suitable chemical stability to withstand the intense radioactive irradiation and the chemical environments (Nawar and Türler, 2019). In this regard, varieties of column chromatographic generators have been developed. The differences among these generators are the sorbent material and the origin of 99Mo. The following subsections highlight different column chromatographic generators approaches and their utilization to produce 99mTc for medical use.
3.2.3.5.1 Alumina-based chromatographic generators
This strategy is the dominant option to afford a reliable and widespread supply of 99mTc for medical harnessing. The generator system consists of a small column filled with a few grams of acidic alumina (Al2O3) as a sorbent matrix. First, the parent 99Mo is extracted from the fission mixture in an alkaline solution as a molybdate anion (99MoO42−). Then, this solution is acidified to maintain favorable sorption conditions. After that, it is loaded on the pre-acidified column, and finally, the 99mTc can be easily eluted in the form of (99mTcO4−) using 0.9% NaCl solution (IAEA, 2013b; Nawar and Türler, 2019).
The earliest produced generator was first eluted with 1 M HNO3. Then, the eluent was changed to HCl until Hammersmith Hospital-London reported the efficient use of the isotonic NaCl solution. These attempts were designed to provide a perfectly compatible eluate solution with the human biological system and to lower the contamination impurity level of 99Mo in the eluted 99mTc solution (Matthews and Mallard, 1965; Richards et al., 1982).
As previously mentioned in Section 2.1.1, the fission production method is associated with a variety of critical difficulties. In addition, it is only restricted to very few centers worldwide, and it is not formally adopted as an integrated routine production approach in developing countries. In order to overcome these challenges, alternative strategies have been proposed using neutron-capture-produced 99Mo of low and medium-specific activity. Figure 5 graphically illustrates a comparison between the alumina generators based on neutron-capture-produced and fission-produced 99Mo.
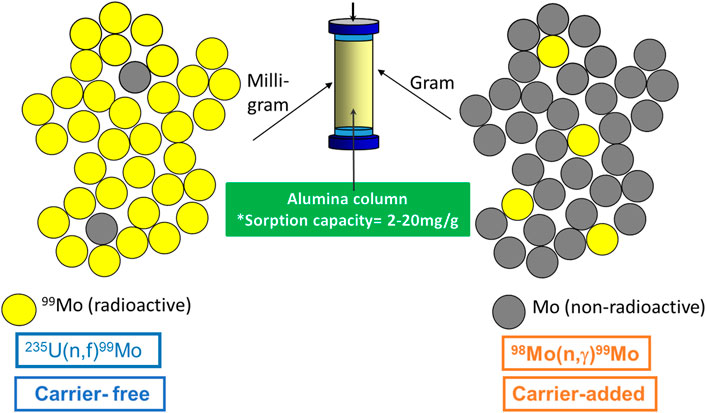
FIGURE 5. Comparison between the alumina generators based on neutron-capture-produced and fission-produced 99Mo.
The first 99mTc generator, which was based on the loading with neutron-capture-produced 99Mo onto the conventional alumina columns, was produced and commercially distributed by Nuclear Consultants Inc. of St Louis, which was merged afterward with Mallinckrodt and Union Carbide Nuclear Corporation in New York in 1966 (Richards et al., 1982). Owing to the distinctly limited sorption capacity of conventional alumina (2–20 mg Mo/g) (Molinsky, 1982) and the fairly low specific activity of 99Mo, the effective practical implementation of this technology suffered from a variety of inherent roadblocks. These limitations include the vital need for considerable amounts of alumina to build a useful generator of a suitable radioactivity level, which results in the elution of the 99mTc in a relatively large volume with a very low Radioactive Concentration (RAC), which accompanies by more tedious post elution concentration steps. However, the use of enriched 98Mo targets increase the radioactivity of the produced 99Mo and accordingly improves the elution profile of the eluted 99mTc, the enriched 98Mo target are costly and cannot be easily recycled (Maoliang, 1997; NAP, 2018). Therefore, these 99mTc generators, which were built on traditional alumina column and neutron-capture-produced 99Mo, were abandoned.
3.2.3.5.2 Gel-type chromatographic generators
The possibility of using neutron-capture-produced 99Mo to produce chromatographic 99mTc generators has promoted the need to explore the gel generator concept (Evans et al., 1987; Moore et al., 1987; Boyd, 1997; Maoliang, 1997). Generally, the gel generator strategy is a multi-step production approach based on using 99Mo-labeled molybdate matrices as column materials. The preparation of these matrices involves many consecutive steps, such as the dissolution of the irradiated 99Mo targets and the precipitation of insoluble solid material with the help of chemical reagents, followed by several filtrations, drying, fragmentation, and column packing steps. At this point, the column is ready for 99mTc elution (IAEA, 1999).
In more detail, the 99Mo-molybdate matrices can be prepared via two main approaches:
• Pre-irradiation formed gel technology: This method includes the preparation of an inactive molybdate gel matrix. After that, it is irradiated in the nuclear reactor and consecutively packed into a column for 99mTc extraction (Narasimhan et al., 1984; Vanaja et al., 1987; Shafiq and Yousif, 1995).
• Post-irradiation formed gel technology: This route starts with the irradiation of the 98Mo target. Then, the molybdate-99Mo matrix is precipitated from its solution as an insoluble gel material. After that, the dried precipitate is packed into a column for 99mTc elution (Boyd, 1982a, 1982b, 1987, 1997; Evans et al., 1987; Moore et al., 1987; El-Kolaly, 1993; IAEA, 1995; Junior et al., 1998; Monroy-Guzman et al., 2003, 2007, 2008; Saraswathy et al., 2004; Davarpanah et al., 2009; Mostafa et al., 2016).
These technologies introduce some critical limitations. For example, the former method suffers from the degradation of the pre-formed gel. The intense radiation level in the reactor induces the reduction of Mo(VI) and Tc(VII) to lower oxidation states, which inevitably results in unsatisfactory elution performance and extremely low 99mTc elution yield owing to the retention of the 99mTc on the column matrix. Moreover, the irradiation of the entire material strongly leads to the production of undesirable radioactive impurities, which consequently lower the radionuclidic purity of the eluted 99mTc solution. The post-irradiation formed gel technology is a multi-step procedure that is hampered by the loss of 99Mo radioactivity, generation of significant amounts of radioactive waste, and excessive radiation exposure to the working personnel (Boyd, 1987; Evans et al., 1987; Zaidi et al., 1990; Saraswathy et al., 2004; Davarpanah et al., 2009). In conclusion, the perceived need for high purity and an adequate radioactive concentration of the produced 99mTc from the generator set severe technical limitations on the mandatory minimum of the required specific activity of 99Mo. As a result, this strategy could not have been able to afford a reliable supply of 99mTc for clinical use.
3.2.3.5.3 Generator systems using nanomaterial-based sorbents
Because of the fact that the sorbent material is the heart of column chromatographic generators, many recent studies have focused on developing a new generation of sorbents that possess a high 99Mo sorption capacity to facilitate the efficient utilization of 99Mo of low and medium-specific activity. These materials are fabricated on the basis of the nanotechnology. Nano-materials attracted considerable attention due to their unique characteristics and innovative applications in multi-disciplinary fields, such as the drug industry, water decontamination development, and different materials production technology (Kharisov et al., 2014). Currently, more than 1000 nanotechnology-based products have become commercially available (Sebastian et al., 2014).
Nanosorbents are size-dependent materials, and their properties not only depend on their chemical composition, but also on their nano-size range and shape. As the particle size decreases, the relative surface area increases, which results in a more significant number of active atoms present on the material surface. These active sites can be translated into a considerable chemical reactivity. In other words, since the chemical reactions occur at the surface, a certain quantity of nano-sized sorbents will be much more reactive than the same amount of macro or micro-scaled materials (Bhatia, 2016).
Based on the formerly mentioned strategies for preparing column chromatographic generators, it is pertinent to point out that the dominant practical challenge is the relatively low sorption capacity of the traditionally used sorbents. This low capacity requires high specific activity 99Mo to produce 99mTc generators of a suitable radioactivity level. This 99Mo can only be made available from the fission of 235U. Some other technologies attempted using neutron-capture-produced 99Mo. However, their applicability is associated with many potential limitations, as discussed above. These problems can be avoided with the use of nanosorbents. That is because reducing the particle size to the nano-scale range offers new opportunities and gives this group of sorbents a number of unique characteristics. For example, these sorbents exhibit novel physical and chemical properties, resulting in extraordinary sorption reactivity and rapid extraction kinetics. In addition, their high chemical and radiation stability draw a clear distinction between them and the conventional bulk material (Baron et al., 2008; Yousefi et al., 2012; Kharisov et al., 2014; Schmid, 2011). Therefore, the use of nanoparticle-based sorbents can offer the following advantages:
• High parent sorption capacity and selectivity due to their large surface area and the increased number of active sites. Consequently, the preparation of a clinical-scale 99Mo/99mTc generator using neutron-capture-produced 99Mo without the limitations of the sorbent capacity can possibly be achieved.
• Simple 99Mo loading process with a minor generation of radioactive waste.
• Considerable chemical stability under different concentrations of acidic and basic solutions leads to the production of 99mTc with high chemical purity.
• High radiation resistance prevents 99Mo breakthrough and provides the eluted 99mTc with adequate radionuclidic purity for nuclear medicine applications.
• Favorable elution performance with a sharp 99mTc elution profile, which supplies the 99mTc eluate with a high radioactive concentration and limits any need for additional concentration efforts.
Table 9 underlines several research attempts using different nanomaterial-based sorbents to develop 99Mo/99mTc generator systems (Chakravarty et al., 2008, 2010c, 2012a, 2013; Fasih et al., 2016; Nawar, 2021). It can be observed that the new sorbents exhibit a much higher sorption capacity for 99Mo compared to the commercially used alumina. Its sorption capacity ranges from (2–20 mg Mo/g) (Molinsky, 1982). Furthermore, the eluted 99mTc can be collected with adequate elution yield, radionuclidic, radiochemical, and chemical purity levels.
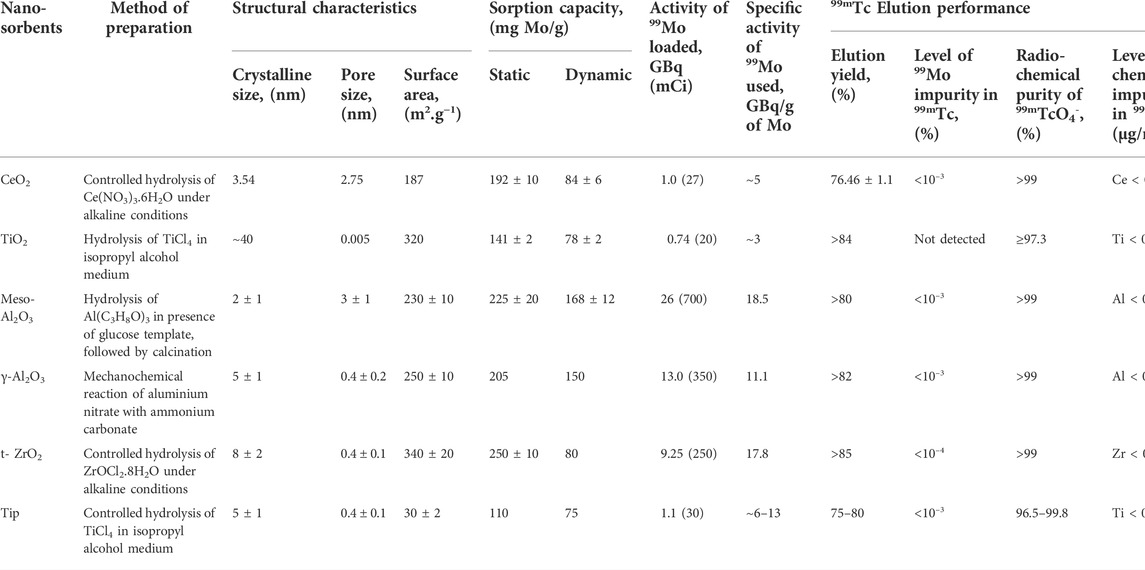
TABLE 9. The summary of 99Mo/99mTc generators developed using nanosorbents: preparation, structural characterization, loading capacity, and elution quality control data.
Even though some milestones have been achieved, it is of importance to recognize that this approach is still in its infancy, and further research areas need to be covered. The developed methods are not currently standard practice in 99Mo/99mTc generators. The generators capacities demonstrated in Table 9 are not sufficient for clinical use. Future studies should intend to scale up for clinical use, which typically involves 99Mo activities of 40–700 GBq. These studies need to investigate the performance of the column matrices in terms of 99mTc purity and carefully assess its labeling efficacy using the conventional kit formulations. Since utilizing nano-sorbent materials and LSA 99Mo represents a new method to produce 99mTc generators, local and international regulatory approvals are needed. This approval is a prerequisite before any clinical administration to the patients to prove that the quality and the efficacy of the produced 99mTc are equal or even show some advantages over the 99mTc obtained from the classical alumina column chromatographic generator containing fission-produced 99Mo (i.e., purity and dose cost).
3.3 Modified 99Mo/99mTc separation systems
3.3.1 99mTc master milker
Many recent studies have focused on improving the 99Mo/99mTc generator performance to provide an efficient extraction of 99mTc. In this context, a modified version of a column chromatographic 99mTc generator, namely the 99mTc Master Milker (TcMM), was designed by Kaken Inc. Mito; Japan in 2014 (Tatenuma et al., 2016a, 2014). The TcMM technique is a new separation concept, which can provide a local supply of 99mTc solution recovered from 99Mo of low and medium-specific activity. The idea is mainly built on the separation of 99mTc from its parent through the use of three different column materials; Activated Carbon (AC), Ion Exchange Resin (IER), and activated acidic Alumina (AL) columns. Figure 6 illustrates the TcMM process scheme.
In more detail, TcMM is a multi-step process that involves the separation of 99mTc from 99Mo in six primary stages, as follows (Sekimoto et al., 2017; Tatenuma et al., 2016b, 2014):
3.3.1.1 Preparation of the 99Mo solution
Following the delivery of the irradiated 99Mo targets, a radioactive 99Mo/99mTc mixture is prepared by dissolving the irradiated targets in 2M NaOH according to the following reaction:
3.3.1.2 Sorption of 99mTc
The formed Na299MoO4 solution is allowed to pass through a stainless steel column loaded with 5 g of AC, by which a particularly selective extraction of 99mTc can take place. The AC column has high sorption efficiency towards 99mTc compared to 99Mo and other radio-contaminants present in the solution. The reason for this selective sorption behavior capability is still under investigation.
3.3.1.3 Removal of 99Mo impurities
During the second step, small amounts of 99Mo contaminants may be adsorbed on the activated charcoal column. In order to remove these impurities, three successive elution steps have to be performed. In the first step, the column is rinsed with a sufficient amount of water. Then, 99Mo is eluted with sodium hydroxide solution. Finally, the column is rewashed with water to remove any residual quantities of 99Mo and NaOH. The flushed 99Mo solution can be allowed to decay to be reused again. This step may be repeated many times until the 99Mo no longer has enough activity to be utilized.
3.3.1.4 Elution of 99mTc
In this step, the stainless steel column containing the activated carbon is heated up to 80–90°C. In the meantime, a sufficient amount of water is passed through the column. Hence, the 99mTc radioactivity can be collected as a warm, weakly alkaline solution.
3.3.1.5 Treatment of the 99mTc eluate
The obtained solution from the previous step is mixed with sodium chloride solution to form a 0.9% saline solution.
3.3.1.6 Refining and purification of 99mTc
After completing the elution process (step 4), the 99mTc solution may still contain some undesirable radio-impurities such as 92mNb, 95Nb, and 96Nb, which arise from the neutron activation of stable radioisotopes of molybdenum, in addition to the parent 99Mo. In order to purify the 99mTc eluate from these radio-contaminants, additional purification steps have to be carried out. Therefore, the resulting 99mTc solution from step 5 is allowed to pass through two successive columns; IER and AL columns, respectively.
The TcMM technology offers a new possibility for separating highly purified 99mTc from 99Mo of low and medium-specific activity. The produced 99mTc shows high labeling efficacy with several pharmaceutical kits. Nevertheless, this technology still requires approval for making human-use 99mTc radiopharmaceuticals. The TcMM can utilize 99Mo radioactivity in the range of kBq to TBq and produce 99mTc of a maximum of 500 Ci (18.5 TBq) per batch (Tatenuma et al., 2016a, 2014). However, the produced 99mTc can only be distributed locally to the usage sites near the separation centers. In addition, the loss of some of the 99mTc radioactivity during the multi-separation and purification steps and the transportation to more distant usage sites is a marked disadvantage.
3.3.2 Northstar radiogenix
Another new modified version of the 99mTc generator is the Radiogenix system, which was designed as a closed system by Northstar Medical Isotopes, LLC, Beloit, Wisconsin; United States (Harvey, 2019). Northstar has received approval from the US Food and Drug Administration (FDA) for using the Radiogenix system to supply 99mTc for nuclear medicine applications (Harvey, 2019; Karcz, 2019). Northstar Radiogenix is an automated, computer-controlled platform designed to separate 99mTc daughter from non-uranium produced 99Mo of low specific activity in a highly alkaline solution.
Radiogenix is based on separating 99mTc with the use of two different separation cartridges, namely the Primary Separation Cartridge (PSC) and 99mTc Product Cartridge (TPC) (Northstar, 2020). Once the irradiated Mo targets are received, they are dissolved, and the 99Mo solution is contained inside the source vessel. After that, the 99Mo/99mTc mixture is pumped through PSC containing Polyethylene Glycol (PEG)-based Aqueous Biphasic Extraction Chromatography (ABEC) resin. This resin selectively retains the 99mTc, and the parent, 99Mo, passes through into the transfer vessel (Rogers et al., 1997; Morley et al., 2012; ANL, 2016). Then, with a stripping solution, 99mTc is washed off from the ABEC resin and passed through the TPC into the 99mTc collection vial. This step ensures the purification of 99mTc from any 99Mo impurities. Eventually, the 99Mo is returned to the source vessel for reuse (Northstar, 2020). When the 99Mo solution reaches its expiration date and is no longer usable, it is returned to the manufacturer, primarily when enriched Mo targets are used (ANL, 2020).
Northstar Radiogenix is a high-tech technology that provides high-quality 99mTc concentrations similar to the high-specific activity-based generators. Nevertheless, it has a physically large design. Moreover, since this method involves a different system and new protocol, the main question is whether the nuclear medicine community will be willing to adopt this approach in the near future.
4 Summary, conclusions, and future perspectives
This paper highlights the fundamental role of 99mTc in medical investigations and its potential production strategies. 99mTc (T1/2 = 6.01 h) is the most widely used diagnostic radionuclide in nuclear medicine. The 99mTc labeled radiopharmaceuticals are prepared mostly in-house by using kit formulations. 99mTc is conveniently derived from 99Mo/99mTc generator systems. The parent 99Mo (T1/2 = 65.94 h) is obtained by chemical separation from neutron-irradiated 235U targets, by which Gram quantities of highly enriched 235U targets are irradiated in the thermal spectrum of research reactors. Then, it is shipped to special processing facilities, where the 99Mo is chemically separated and purified. The obtained specific activity of 99Mo is very high. Nonetheless, the process is associated with generating substantial amounts of nuclear waste, and the handling of 235U generates serious proliferation issues. Furthermore, the global 99Mo supply is fragile and insecure, as it only relies on few aging nuclear reactors. Since the 99mTc supply depends significantly on the fission-produced 99Mo availability, it seriously suffered during renewed emergency periods, such as the past 99Mo production crisis and the current COVID-19 pandemic. To solve this challenge, many alternative ideas were put forward to mediate the problem. However, the commercial aspect should not be overlooked. These new methods to supply 99mTc should be competitive in terms of production volume, price, and ease of applicability for the end-user. One method is to obtain 99mTc by direct cyclotron production via the 100Mo (p,2n)99mTc reaction. However, many nuclear medicine departments are hard to persuade to switch from the column 99mTc generators and kit formulations to the 99mTc-radiopharmaceuticals produced in centralized radiopharmacies that obtain 99mTc by this route. Mainly, its supply advantage is limited to the centers close to the production site, and the daily transport costs can develop into a significant cost factor. In addition, this strategy still seeks to design a realistic supply plan that can deal with the production difficulties and find innovative logistic solutions to meet rapid product delivery requirements. Thereby, this strategy is not clearly the answer for a routine 99mTc supply.
In order to establish a sufficient 99mTc supply sustainably, two challenges need to be optimized:
• First, enhance the 99Mo economy by guaranteeing a reliable and decentralized worldwide supply source of Low Specific Activity (LSA) 99Mo. This concept can be made available by fostering the neutron irradiation of 98Mo targets in nuclear power reactors. Commercial nuclear power reactors provide intense neutron fluxes. More importantly, high fluxes of epi-thermal neutrons are available. Due to the activation of 98Mo solely, no chemical separation is required, and consequently, very marginal quantities of radioactive waste are generated. In addition, this method is proliferation-resistant, as no fissile materials are involved. Moreover, it needs less technical arrangements and financial budgets compared to the uranium fission approach. Another promising approach aims at producing 99Mo through photonuclear reactions through the 100Mo(γ,n)99Mo reaction. The currently available specific activity is about 6 Ci/g of 99Mo after a 24 h bombardment. The 99Mo produced has a low specific activity. Therefore, the issue of the low sorption capacity of the conventional sorbents persists (2–20 mg Mo/g).
• Second, the key lies in developing a new generation of column materials that possess high sorption capacity and selectivity to recompense for the low specific activity of the obtained 99Mo. A promising approach is the development of a chromatographic column 99mTc generator with the use of nanomaterial-based sorbents. The use of nanomaterial-based sorbents deserves considerable attention due to their high sorption capacity, which arises from their large surface area, high radiation resistance, and chemical stability compared to conventionally used sorbents. These unique characteristics could be the cornerstone towards developing a new generation of 99Mo/99mTc generators that produce 99mTc with high purity and radioactivity concentration adequate for existing kit formulations. Consequently, this approach would encourage the possibility of using 99Mo of low specific activity without the limitations of the sorbent capacity.
Since the 99mTc production techniques are usually selected based on different economic, technical, and logistical circumstances, the proposed solution in this article is inexpensive, realistic, and could be implemented in a short period. Furthermore, it has the potential to ensure a secure supply source of clinical-grade 99mTc for millions of patients worldwide, especially for many countries which do not have access to fission 99Mo technology.
Author contributions
MN suggested the idea, collected the data, and drafted the original manuscript. Furthermore, MN planned for the acquisition of the financial support for the project leading to this publication. AT supervised the project, performed calculations on nuclear reactions, and reviewed the original manuscript.
Funding
The research was funded by the Swiss National Science Foundation (grant number CRSII5_180352). MN gratefully acknowledges the funding support of the Swiss Government Excellence fellowships program (fellowship No: 2017.1028).
Acknowledgments
The authors would like to thank Dr. Alaa El-Daoushy (Egyptian Atomic Energy Authority) for her fruitful discussion and comments. Furthermore, the authors wish to acknowledge Dr. Abdallah Zaki (University of Geneva) for his valuable assistance in drafting some figures. Finally, the authors would like to thank Dr. Mehran Vagheian (University of Bern) for his contribution to calculating the 99Mo production yield in photonuclear reactions.
Conflict of interest
The authors declare that the research was conducted in the absence of any commercial or financial relationships that could be construed as a potential conflict of interest.
Publisher’s note
All claims expressed in this article are solely those of the authors and do not necessarily represent those of their affiliated organizations, or those of the publisher, the editors and the reviewers. Any product that may be evaluated in this article, or claim that may be made by its manufacturer, is not guaranteed or endorsed by the publisher.
References
Abderrahim, H. A., Baeten, P., Bruyn, D. D., Heyse, J., Schuurmans, P., and Wagemans, J. (2010). MYRRHA, a multipurposehybrid research reactor for high-end applications. Nucl. Phys. News 20 (1), 24–28. doi:10.1080/10506890903178913
Ali, S. A., and Ache, H. G. (1987). Production techniques of fission molybdenum-99. Radiochim. Acta. 41 (2-3), 65–72. doi:10.1524/ract.1987.41.23.65
Allen, J. F. (1965). An improved technetium-99m generator for medical applications. Int. J. Appl. Radiat. Isot. 16 (5), 332–334. doi:10.1016/0020-708X(65)90058-X
Anders, E. (1960). The radiochemistry of technetium, National Academy of Sciences —National Research Council, Nuclear Science Series: NAS-NS 3021, U.S.A. Available at: https://www.osti.gov/servlets/purl/4073069.
Anderson, C. J., Ling, X., Schlyer, D. J., and Cutler, C. S. (2019). “A Short History of Nuclear Medicine,” in Radiopharmaceutical chemistry. Editors J. S. Lewis, A. D. Windhorst, and B. M. Zeglis (Cham, Switzerland: Springer Nature Switzerland AG), 11–26. doi:10.1007/978-3-319-98947-1_2
ANL (2016). Argonne National Laboratory Report. ANL/NE-16/43. Available at: https://publications.anl.gov/anlpubs/2017/04/134741.pdf.Development of ABEC Column for Separation of Tc-99 from Northstar Dissolved Target Solution
ANL (2020). Argonne National Laboratory Report. ANL-20/20.Recycling Isotopically Enriched Mo from the RadioGenix Waste Stream
Anwar, M., Lathrop, K., Rosskelly, D., Harpen, P. V., Lathrop, K., and Rosselly, D. (1968). Pertechnetate production from 99Mo by liquid-liquid extraction. J. Nucl. Med. 9, 298–299.
Araz, M., Çayır, D., Köybaşıoğlum, F. F., Karabacak, H., and Çakal, E. (2020). Four a Typical Parathyroid Adenomas Detected by Dual Phase Tc-99m MIBI SPECT. Mol. Imaging Radionucl. Ther. 29 (1), 33–36. doi:10.4274/mirt.galenos.2019.09326
Atkins, H. L. (1975). Radiopharmaceuticals. Phys. Rep. 21 (6), 315–367. doi:10.1016/0370-1573(75)90013-7
Ávila-Sánchez, M., Ferro-Flores, G., Jiménez-Mancilla, N., Ocampo-Garcia, B., Bravo-Villegas, G., Luna-Gutierrez, M., et al. (2020). Synthesis and preclinical evaluation of the 99mTc-/177Lu-CXCR4-L theranostic pair for in vivo chemokine-4 receptor-specific targeting. J. Radioanal. Nucl. Chem. 324 (1), 21–32. doi:10.1007/s10967-020-07043-6
Baker, R. J. (1971). A system for the routine production of concentrated technetium-99m by solvent extraction of molybdenum-99. Int. J. Appl. Radiat. Isot. 22 (8), 483–485. doi:10.1016/0020-708X(71)90170-0
Bandeira, J. V., Sabino, C. S., Aun, P. E., Mendes, V. L., and Agudo, E. G. (2002). Development of a technique for using 99mTc as an absorbable tracer for hydrodynamic studies of fine sediments in suspension. Appl. Radiat. Isot. 57 (1), 85–92. doi:10.1016/s0969-8043(02)00068-4
Banerjee, S., Pillai, M. R. A., and Ramamoorthy, N. (2000). Evolution of Tc-99m in Diagnostic Radiopharmaceuticals. Semin. Nucl. Med. 31 (4), 260–277. doi:10.1053/snuc.2001.26205
Baron, R., Campbellm, F. W., Streeterm, I., Xianom, L., and Comptonm, R. G. (2008). Facile Method for the Construction of Random Nanoparticle Arrays on a Carbon Support for the Development of Well-defined Catalytic Surfaces. Int. J. Electochem. Sci. 3 (5), 556. Available at: http://www.electrochemsci.org/papers/vol3/3050556.pdf.
Beaver, J. E., and Hupf, H. B. (1971). Production of 99m Tc on a medical cyclotron: A feasibility study. J. Nucl. Med. 12 (11), 739–741. Available at: http://jnm.snmjournals.org/content/12/11/739.full.pdf.
Berne, P. P., and Thereska, J. (2004). Simulation of a radiotracer experiment by flow and detection-chain modelling: A first step towards better interpretation. Appl. Radiat. Isot. 60 (6), 855–861. doi:10.1016/j.apradiso.2003.12.010
Bertsche, K. (2010). Accelerator Production Options for 99Mo, University of North Texas Libraries, United States. Available at: https://digital.library.unt.edu/ark:/67531/metadc845983/.
Bhatia, D. S., and Turel, Z. R. (1989). Solvent extraction of 99mTc/VII/ with methylene blue into nitrobenzene. J. Radioanalytical Nucl. Chem. Lett. 135 (2), 77–83. doi:10.1007/BF02163538
Bhatia, S. (2016). “Nanoparticles types, classification, characterization, fabrication methods and drug delivery applications,” in Natural polymer drug delivery systems (Cham, Switzerland: Springer International Publishing), 33–93. doi:10.1007/978-3-319-41129-3_2
Binney, S. E., and Scherpdz, R. I. (1978). A review of the delayed fission neutron technique. Nucl. Instrum. Methods 154 (3), 413–431. doi:10.1016/0029-554X(78)90069-1
Borroto, J. I., Dominguez, J., Griffith, J., Fick, M., and Leclerc, J. P. (2003). Technetium-99m as a tracer for the liquid RTD measurement in opaque anaerobic digester: Application in a sugar wastewater treatment plant. Chem. Eng. Process. Process Intensif. 42 (11), 857–865. doi:10.1016/S0255-2701(02)00109-5
Boschi, A., Martini, P., Pasquali, M., and Uccelli, L. (2017). Recent achievements in Tc-99m radiopharmaceutical direct production by medical cyclotrons. Drug Dev. Ind. Pharm. 43 (9), 1402–1412. doi:10.1080/03639045.2017.1323911
Boyd, R. E. (1987). Technetium Generators: Status and Prospects. Radiochim. Acta. 41 (2-3), 59–64. doi:10.1524/ract.1987.41.23.59
Boyd, R. E. (1982a). Technetium-99m generators – The available options. Int. J. Appl. Radiat. Isot. 33 (10), 801–809. doi:10.1016/0020-708X(82)90121-1
Boyd, R. E. (1997). The gel generator: A viable alternative source of 99mTc for nuclear medicine. Appl. Adiat. Isot. 48 (8), 1027–1033. doi:10.1016/S0969-8043(96)00298-9
Chakravarty, R., Dash, A., and Pillai, M. R. A. (2012b). Electrochemical Separation is an Attractive Strategy for Development of Radionuclide Generators for Medical Applications. Curr. Radiopharm. 5 (3), 271–287. doi:10.2174/1874471011205030271
Chakravarty, R., Dash, A., Pillai, M. R. A., and Venkatesh, M. (2010b). Post elution concentration of 188Re by an electrochemical method. Appl. Radiat. Isot. 68 (12), 2302–2305. doi:10.1016/j.apradiso.2010.06.022
Chakravarty, R., Dash, A., and Venkatesh, M. (2010a). A novel electrochemical technique for the production of clinical grade 99mTc using (n, γ) 99Mo. Nucl. Med. Biol. 37 (1), 21–28. doi:10.1016/j.nucmedbio.2009.08.010
Chakravarty, R., and Dash, A. (2013). Role of Nanoporous Materials in Radiochemical Separations for Biomedical Applications. J. Nanosci. Nanotechnol. 13 (4), 2431–2450. doi:10.1166/jnn.2013.7349
Chakravarty, R., Ram, R., Dash, A., and Pillai, M. R. A. (2012a). Preparation of clinical-scale 99Mo/99mTc column generator using neutron activated low specific activity 99Mo and nanocrystalline γ-Al2O3 as column matrix. Nucl. Med. Biol. 39 (7), 916–922. doi:10.1016/j.nucmedbio.2012.03.010
Chakravarty, R., Ram, R., Mishra, R., Sen, D., Mazumder, S., Pillai, M. R. A., et al. (2013). Mesoporous Alumina (MA) based double column approach for development of a clinical scale 99Mo/99mTc generator using (n, γ) 99Mo: An enticing application of nanomaterial. Ind. Eng. Chem. Res. 52 (33), 11673–11684. doi:10.1021/ie401042n
Chakravarty, R., Sarkar, S. K., Venkatesh, M., and Dash, A. (2012c). An electrochemical procedure to concentrate 99mTc availed from a zirconium [99Mo] molybdate gel generator. Appl. Radiat. Isot. 70 (2), 375–379. doi:10.1016/j.apradiso.2011.09.024
Chakravarty, R., Shukla, R., Gandhi, S., Ram, R., Dash, A., Venkatesh, M., et al. (2008). Polymer Embedded Nanocrystalline Titania Sorbent for 99Mo-99mTc Generator. J. Nanosci. Nanotechnol. 8 (9), 4447–4452. doi:10.1166/jnn.2008.280
Chakravarty, R., Shukla, R., Ram, R., Tyagi, A. K., Dash, A., Venkatesh, M., et al. (2010c). Practicality of Tetragonal Nano-Zirconia as a Prospective Sorbent in the Preparation of 99Mo/99mTc Generator for Biomedical Applications. Chromatographia 72 (9), 875–884. doi:10.1365/s10337-010-1754-z
Chakravarty, R., Venkatesh, M., and Dash, A. (2011). A novel electrochemical 99Mo/99mTc generator. J. Radioanal. Nucl. Chem. 290 (1), 45–51. doi:10.1007/s10967-011-1113-z
Chang, S. H. (2016). Types of bulk liquid membrane and its membrane resistance in heavy metal removal and recovery from wastewater. Desalination Water Treat. 57 (42), 19785–19793. doi:10.1080/19443994.2015.1102772
Chattopadhyay, S., Barua, L., Das, S. S., De, A., Kumar, U., Mitra, A., et al. (2014). Pharmaceutical grade sodium [99mTc] pertechnetate from low specific activity 99Mo using an automated 99Mo/99mTc-TCM-autosolex generator. J. Radioanal. Nucl. Chem. 302, 781–790. doi:10.1007/s10967-014-3211-1
Chattopadhyay, S., Das, S. S., and Barua, L. (2010). A simple and rapid technique for recovery of 99mTc from low specific activity (n, γ)99Mo based on solvent extraction and column chromatography. Appl. Radiat. Isot. 68 (1), 1–4. doi:10.1016/j.apradiso.2009.07.024
Chaudry, M. A. (2000). Acid effect on 99mTc and 99Mo mutual separation and their transport across supported liquid membrane extraction system Czechoslov. J. Phys. 50 (2), 271–279. doi:10.1023/A:1022894825658
Chen, J., Boerrigter, H., and Veltkamp, A. C. (2001). Technetium(VII) transport across supported liquid membranes (SLMs) containing 2-nitrophenyl octyl ether (NPOE). Radiochim. Acta 89 (8), 523–528. doi:10.1524/ract.2001.89.8.523
Chen, J., and Tomasberger, T. (2001). Solvent extraction of Tc(VII) by the mixture of TBP and 2-nitrophenyl octyl ether. J. Radioanal. Nucl. Chem. 247 (3), 519–523. doi:10.1023/A:1010622208450
Childs, B. C. (2017). Volatile Technetium Oxides: Implications for Nuclear Waste Vitrification. PhD thesis (Las Vegas: University of Nevada). Available at: https://digitalscholarship.unlv.edu/thesesdissertations/2958.
Christian, J. D., Petti, D. A., Kirkham, R. J., and Bennett, R. G. (2000). Advances in Sublimation Separation of Technetium from Low-Specific-Activity Molybdenum-99. Ind. Eng. Chem. Res. 39 (9), 3157–3168. doi:10.1021/ie9903792
Cols, H. J., Cristinin, P. R., and Manzini, A. C. (2000). “Mo–99 From Low–Enriched Uranium,” in Presented in part at International Meeting on Reduced Enrichment for Research and Test Reactors, Argentina. Available at: https://inis.iaea.org/collection/NCLCollectionStore/_Public/42/024/42024785.pdf.
Conner, C., Sedlet, J., Wiencek, T,C., McGann, D. J., Hofman, G. L., Vandegrift, G. F., et al. (2000). “Production of Mo-99 from LEU targets acid-side processing,” in Presented in part at International Meeting on Reduced Enrichment for Research and Test Reactors, Las Vegas, Nevada, U.S.A. Available at: https://inis.iaea.org/collection/NCLCollectionStore/_Public/32/025/32025774.pdf.
Crasta, R., Naik, H., Suryanarayana, S. V., Prajapati, P. M., Jagadisan, K. C., Thakare, S. V., et al. (2011). Photo-neutron cross-section of 100Mo. J. Radioanal. Nucl. Chem. 290, 367–373. doi:10.1007/s10967-011-1247-z
Dash, A., and Chakravarty, R. (2016). Nanomaterial based adsorbent: Promises, opportunities, and challenges to develop column chromatography radionuclide generators for nuclear medicine. Sep. Purif. Rev. 46 (2), 91–107. doi:10.1080/15422119.2016.1205089
Dash, A., Chakravarty, R., Ram, R., Pillai, K. T., Yadav, Y. Y., Wagh, D. N., et al. (2012). Development of a 99Mo/99mTc generator using alumina microspheres for industrial radiotracer applications. Appl. Radiat. Isot. 70 (1), 51–58. doi:10.1016/j.apradiso.2011.07.012
Davarkhah, R., Farahmand, E., Samadfam, M., Tavasoli, M., Zaheri, P., and Shamsipur, M. (2018). Selective separation of yttrium(III) through a liquid membrane system using 2-thenoyltrifluoroacetone as an extractant carrier. Chem. Pap. 72 (6), 1487–1497. doi:10.1007/s11696-018-0407-9
Davarpanah, M. R., Nosrati, S. A., Fazlali, M., Boudani, M. K., Khoshhosn, H., Maragheh, M. G., et al. (2009). Influence of drying conditions of zirconium molybdate gel on performance of 99mTc gel generator. Appl. Radiat. Isot. 67 (10), 1796–1801. doi:10.1016/j.apradiso.2009.06.011
Dhami, P. S., Naik, P. W., Dudwadkar, N. L., Kannan, R., Achuthan, P. V., Moorthy, A. D., et al. (2007). Studies on the development of a two stage SLM system for the separation of carrier-free 90Y using KSM-17 and CMPO as carriers. Sep. Sci. Technol. 42 (5), 1107–1121. doi:10.1080/01496390601174323
Dilworth, J. R., and Pascu, S. (2015). “The radiopharmaceutical chemistry of technetium and rhenium,” in The chemistry of molecular imaging. Editors N. Long, and W. T. Wong (Hoboken, New Jersey: John Wiley & Sons), 137–164.
Dutta, S., and Mohapatra, P. K. (2013). Studies on the separation of 90Y from 90Sr by solvent extraction and supported liquid membrane using TODGA: Role of organic diluent. J. Radioanal. Nucl. Chem. 295 (3), 1683–1688. doi:10.1007/s10967-012-2233-9
Eckelman, W. C., and Coursey, B. M. (1982). Technetium-99m: Generators, chemistry and preparation of radiopharmaceuticals. Int. J. Appl. Radiat. Isot. 33 (10), 793–806.
Eckelman, W. C. (2009). Unparalleled Contribution of Technetium-99m to Medicine Over 5 Decades. JACC Cardiovasc. Imaging 2 (3), 364–368. doi:10.1016/j.jcmg.2008.12.013
El-Kolaly, M. T. (1993). A 99Mo/99mTc generator based on the use of zirconium molybdophosphate-99Mo gel. J. Radioanalytical Nucl. Chem. Articles 170 (2), 293–298. doi:10.1007/BF02041464
Esposito, J., Vecchi, G., Pupillo, G., Taibi, A., Uccelli, L., Boschi, A., et al. (2013). Evaluation of 99Mo and 99mTc Productions Based on a High-Performance Cyclotron. Sci. Technol. Nucl. Installations 2013, 1–14. doi:10.1155/2013/972381
Evans, J. V., Moore, P. W., Shying, M. E., and Sodeau, J. M. (1987). Zirconium molybdate gel as generator for technetium-99m: The concept and its evaluation. Int. J. Radiat. Appl. Instrum. Part A. Appl. Radiat. Isotopes 38 (1), 19–23. doi:10.1016/0883-2889(87)90230-9
Fasih, T. W., Sakr, T. M., Ayoub, R. R., and Amin, M. (2016). Preparation and evaluation of nano-crystalline titania as sorbent for 99Mo/99mTc generator. Sep. Sci. Technol. 51 (13), 2115–2121. doi:10.1080/01496395.2016.1202976
Fauquex, P. F., Flaschel, E., Renken, A., Do, H. P., Friedli, C., Lerch, P., et al. (1983). 24Na and 99mTc tracers applied to the characterization of liquid–solid fluidized bed and hydraulic transport reactors. Int. J. Appl. Radiat. Isot. 34 (10), 1465–1468. doi:10.1016/0020-708x(83)90048-0
Gagnon, K., Bénard, F., Kovacs, M., Ruth, T. J., Schaffer, P., Wilson, J. S., et al. (2011). Cyclotron production of 99mTc: Experimental measurement of the 100Mo(p, x)99Mo, 99mTc and 99gTc excitation functions from 8 to 18 MeV. Nucl. Med. Biol. 38 (6), 907–916. doi:10.1016/j.nucmedbio.2011.02.010
Gan, Q., Song, X., Zhang, X., and Zhang, J. (2020). Preparation and evaluation of 99mTc-labeled HYNIC-palbociclib analogs for cyclin-dependent kinase 4/6-positive tumor imaging. Eur. J. Med. Chem. 188, 112032. doi:10.1016/j.ejmech.2019.112032
Gedeonov, A. D., and Nosov, A. A. (1989). NOBIUM-93-M production with the help of the reaction. Isotopenpraxis Isotopes Environ. Health Stud. 25 (7), 294–298. doi:10.1080/10256018908624135
Gerlit, J. (1956). “Some chemical properties of technetium,” in Presented in part at Conference Peaceful Uses of Atomic Energy, Geneva.
Grummitt, W., and Wilkinson, G. (1946). Fission Products of 235U. Nature 158 (4005), 163. doi:10.1038/158163a0
Guérin, B., Tremblay, S., Rodrigue, S., Rousseau, J. A., Dumulon-Perreault, V., Lecomte, R., et al. (2010). Cyclotron production of 99mTc: An approach to the medical isotope crisis. J. Nucl. Med. 51 (4), 13N–16N.
Guillaume, M., and Brihaye, C. (1986). Generators for short-lived gamma and positron emitting radionuclides: Current status and prospects. Int. J. Radiat. Appl. Instrum. Part B. Nucl. Med. Biol. 13 (2), 89–100. doi:10.1016/0883-2897(86)90223-0
Gumiela, M. (2018). Cyclotron production of 99mTc: Comparison of known separation technologies for isolation of 99mTc from molybdenum targets. Nucl. Med. Biol. 58, 33–41. doi:10.1016/j.nucmedbio.2017.11.001
Gyves, J. D., and Miguel, E. R. (1999). Metal ion separations by supported liquid membranes. Ind. Eng. Chem. Res. 38 (6), 2182–2202. doi:10.1021/ie980374p
Hallaba, E., and El-Asrag, H. A. (1975). Kurze originalmitteilungen on the sublimation of 99mTe from irradiated molybdenum trioxide. Isotopenpraxis Isotopes Environ. Health Stud. 11 (8), 290–292. doi:10.1080/10256017508543805
Hanemaayer, V., Benard, F., Buckley, K. R ., Klug, J., Kovacs, M., Leon, C., et al. (2014). Solid targets for 99mTc production on medical cyclotrons. J. Radioanal. Nucl. Chem. 299 (2), 1007–1011. doi:10.1007/s10967-013-2626-4
Haroon, J., and Nichita, E. (2021). PHWR Reactivity Device Incremental Macroscopic Cross Sections and Reactivities for a Molybdenum-Producing Bundle and a Standard Bundle. Nucl. Technol. 208 (2), 246–267. doi:10.1080/00295450.2021.1929768
Harvey, J. (2019). “NorthStar: The new producer and distributor of Mo-99,” in Presented at the 2019 NNSA Mo-99 Stakeholders Meeting, Chicago, IL, USA. Available at: https://mo99.ne.anl.gov/2019stakeholders/pdfs/2.3_Harvey.pdf.
Hasan, S., and Prelas, M. A. (2020). Molybdenum-99 production pathways and the sorbents for 99Mo/99mTc generator systems using (n, γ) 99Mo: A review. SN Appl. Sci. 2, 1782. doi:10.1007/s42452-020-03524-1
Hermanne, A., Tárkányi, F., and Takács, S. (2007). “Production of medically relevant radionuclides with medium energy deuterons,” in Presented in part at International Conference on Nuclear Data for Science and Technology. doi:10.1051/ndata:07153
Hetherington, E. L. R., and Boyd, R. E. (1999). “Production technologies for molybdenum-99 and technetium-99m,” in IAEA-TECDOC-1065 (Vienna, Austria: International Atomic Energy Agency), 19–22. Available at: https://www-pub.iaea.org/MTCD/Publications/PDF/te_1065_prn.pdf.
Hidalgo, J. U., Wright, R. M., and Wooten, M. M. (1967). Prediction of Technetium-99m Yield from Molybdenum-99 Generators. J. Nucl. Med. 8 (6), 426–429.
Hou, X., Celler, A., Grimes, J., Bénard, F., and Ruth, T. (2012). Theoretical dosimetry estimations for radioisotopes produced by proton-induced reactions on natural and enriched molybdenum targets. Phys. Med. Biol. 57 (6), 1499–1515. doi:10.1088/0031-9155/57/6/1499
Hou, X., Tanguay, J., Buckley, K. R., Schaffer, P., Bénard, F., Ruth, T. J., et al. (2016). Molybdenum target specifications for cyclotron production of 99mTc based on patient dose estimates. Phys. Med. Biol. 61 (2), 542–553. doi:10.1088/0031-9155/61/2/542
Hughes, C. E., Airey, P. L., Duran, E. B., Miller, B. M., and Sombrito, E. (2004). Using radiotracer techniques for coastal hydrodynamic model evaluation. J. Environ. Radioact. 76 (1-2), 195–206. doi:10.1016/j.jenvrad.2004.03.026
Hyde, E. K. (1964). The Nuclear Properties of the Heavy Elements: Fission Phenomena. vol 3. New Jersey: Prentice-Hall International Series in Chemistry, Prentice-Hall, Englewood Cliffs.
IAEA (1995). Alternative technologies for 99mTc generators: Final report of a co-ordinated research programme 1990-1994. IAEA TECDOC-582. Vienna: Austria International Atomic Energy Agency. Avaialble at: https://www-pub.iaea.org/MTCD/publications/PDF/te_852_prn.pdf.
IAEA (2017). Cyclotron Based Production of Technetium-99m: IAEA Radioisotopes and Radiopharmaceuticals Reports No. 2. Vienna, Austria: International Atomic Energy Agency. Available at: https://www-pub.iaea.org/MTCD/Publications/PDF/P1743_web.pdf.
IAEA (2015). Feasibility of producing molybdenum-99 on a small scale using fission of low enriched uranium or neutron activation of natural molybdenum. Technical reports series, in Technical Report Series No. 478. Vienna, Austria: International Atomic Energy Agency. Available at: https://www-pub.iaea.org/MTCD/Publications/PDF/trs478web-32777845.pdf.
IAEA (2008). Handbook of Nuclear Data for Safeguards: Database Extensions, International Nuclear Data Committee. Vienna, Austria: International Atomic Energy Agency. Available at: https://www-nds.iaea.org/sgnucdat/safeg2008.pdf and https://www-nds.iaea.org/sgnucdat/c1.htm#ref.
IAEA (2011). IAEA Helps to Close Radioisotope Production Gap. Vienna, Austria: International Atomic Energy Agency. Available at: https://www.iaea.org/newscenter/news/iaea-helps-close-radioisotope-production-gap.
IAEA (2002). IAEA Safeguards Glossary (2001 Edition). International Nuclear Verification Series No. 3. Vienna, Austria: International Atomic Energy Agency.
IAEA (2005). Management of High Enriched Uranium for Peaceful Purposes: Status and Trends. IAEA-TECDOC-1452. Vienna, Austria: International Atomic Energy Agency.
IAEA (1998). Management of radioactive waste from 99Mo production. IAEA-TECDOC-1051. Vienna, Austria: International Atomic Energy Agency.
IAEA (2013a). Non-HEU production technologies for Molybdenum-99 and Technetium-99m. IAEA Nuclear Energy Series No. NF-T-5.4. Vienna, Austria: International Atomic Energy Agency. Available at: https://www-pub.iaea.org/MTCD/Publications/PDF/Pub1589_web.pdf.
IAEA (1999). Production Technologies for Molybdenum-99 and Technetium-99m. Vienna, Austria: International Atomic Energy Agency.
IAEA (2018). Quality Control in the Production of Radiopharmaceuticals. IAEA TECDOC SERIES: IAEA-TECDOC-1856. Vienna, Austria: International Atomic Energy Aganecy. Available at: https://www-pub.iaea.org/MTCD/Publications/PDF/TE-1856web.pdf.
IAEA (2013b). Radiotracer generators for industrial applications: IAEA Radiation Technology Series No. 5. Vienna, Austria: International Atomic Energy Agency. Available at: https://www-pub.iaea.org/MTCD/Publications/PDF/Pub1579_web.pdf.
IAEA (2009). Technetium-99m Radiopharmaceuticals: Status and Trends. Vienna, Austria: International Atomic Energy Agency. IAEA Radioisotopes and Radiopharmaceuticals Series No. 1. Available at: https://www-pub.iaea.org/MTCD/Publications/PDF/Pub1405_web.pdf.
IBA (2022). Ready to accelerate into the future? CYCLONE®IKON by IBA: Louvain-la-Neuve, Belgium. Available at: https://www.iba-radiopharmasolutions.com/sites/default/files/2021-08/Cyclone%20IKON%20Final%20R01%20_LD.pdf (Accessed 6 8, 2022).
ICRP (2015). Radiation dose to patients from radiopharmaceuticals: a compendium of current information related to frequently used substances. Ontario, Canada: ICRP publication 128.
Iqbal, M., and Ejaz, M. (1974). Solvent extraction of technetium(VII) by 4-(5-nonyl)pyridine and its separation from uranium and some fission products. J. Radioanal. Chem. 23 (1-2), 51–62. doi:10.1007/BF02514344
Junior, J. A. O., Lima, A. L. V. P., Da Silva, N. C., Da Silva, R. C., Nieto, R. C., and De Velosa, A. C. (1998). “Preparation of a gel of zirconium molybdate for use in the generators of 99Mo-99mTc prepared with 99Mo produced by the 98Mo(n,γ)99Mo reaction,” in Presented in part at International Meeting on Reduced Enrichment for Research and Test Reactors, Sao Paulo, Brazil. Available at: https://inis.iaea.org/collection/NCLCollectionStore/_Public/35/040/35040284.pdf.
Kantzas, A., Hamilton, K., Zarabi, T., Bhargava, A., Wright, I., Brook, G., et al. (2000). Application of gamma camera imaging and SPECT systems in chemical processes. Chem. Eng. J. 77 (1-2), 19–25. doi:10.1016/S1385-8947(99)00148-5
Karcz, P. (2019). “NNSA’s Mo-99 program: Accelerating reliable, non-HEU Mo-99 production capabilities in the United States,” in Presented at the 2019 NNSA Mo-99 Stakeholders Meeting, Chicago, IL, USA. Available at: https://mo99.ne.anl.gov/2019stakeholders/pdfs/1.1_Karcz.pdf.
Kharisov, B. I., Kharissova, O. V., and Berdonosov, S. S. (2014). Radioactive Nanoparticles and their Main Applications: Recent Advances. Recent Pat. Nanotechnol. 8 (2), 79–96. doi:10.2174/187221050802140618143846
Knapp, F. F., and Baum, R. P. (2012). Radionuclide generators – A new renaissance in the development of technologies to provide diagnostic and therapeutic radioisotopes for clinical applications. Curr. Radiopharm. 5 (3), 175–177. doi:10.2174/1874471011205030175
Knapp, F. F., and Mirzadeh, S. (1994). The continuing important role of radionuclide generator systems for nuclear medicine. Eur. J. Nucl. Med. 21 (10), 1151–1165. doi:10.1007/BF00181073
Konheiser, J., Seidl, M., Brachem, C., and Mueller, S. (2016). Study of the uncertainties due to position change of the PWR aeroball measurement system. J. Nucl. Sci. Technol. 53 (11), 1715–1722. doi:10.1080/00223131.2016.1151840
Krishichayan, , Bhike, M., Finch, S. W., Howell, S. R., Tonchev, A. P., and Tornow, W. (2017). Photofission cross-section ratio measurement of 235U/238U using monoenergetic photons in the energy range of 9.0–16.6 MeV. Nucl. Instrum. Methods. Phys. Res. A 854, 40–44. doi:10.1016/j.nima.2017.02.043
Lagunas-Solar, M. C., Kiefer, P. M., Carvacho, O. F., Lagunas, C. A., and Cha, Y. P. (1991). Cyclotron production of NCA 99mTc and 99Mo: An alternative non-reactor supply source of instant 99mTc and 99Mo→99mTc generators. Int. J. Radiat. Appl. Instrum. Part A. Appl. Radiat. Isotopes 42 (7), 643–657. doi:10.1016/0883-2889(91)90035-Y
Lamson, M., Hotte, C. E., and Ice, R. D. (1976). Practical generator kinetics. J. Nucl. Med. Technol. 4, 21–26. Available at: https://tech.snmjournals.org/content/jnmt/4/1/21.full.pdf.
Lavi, N. (1978). The study of conditions for the preparation and application of 99Mo/99mTc generators starting from irradiated molybdenum metal. J. Radioanal. Chem. 42 (1), 25–34. doi:10.1007/BF02520623
Lebeda, O., van Lier, E. J., Štursa, J., Ráliš, J., and Zyuzin, A. (2012). Assessment of radionuclidic impurities in cyclotron produced 99mTc. Nucl. Med. Biol. 39 (8), 1286–1291. doi:10.1016/j.nucmedbio.2012.06.009
Lebowitz, E., and Richards, P. (1974). Radionuclide Generator Systems. Semin. Nucl. Med. 4 (3), 257–268. doi:10.1016/S0001-2998(74)80013-9
Leggett, R., and Giussani, A. (2015). A bio kinetic model for systemic technetium in adult humans. J. Radiol. Prot. 35 (2), 297–315. doi:10.1088/0952-4746/35/2/297
Magill, J., Dreher, R., and Soti, Z. (2022). Karlsruher Nuklidkarte: Chart of the nuclides 11th Edition. Karlsruher, Germany: Nucleonica GmbH.
Mang’era, K., Ogbomo, K., Zriba, R., Fitzpatrick, J., Brown, J., Pellerin, E., et al. (2015). Processing and evaluation of linear accelerator-produced 99Mo/99mTc in Canada. J. Radioanal. Nucl. Chem. 305, 79–85. doi:10.1007/s10967-015-3997-5
Maoliang, L. (1997). “Production of gel-type Tc-99m generator for nuclear medicine,” in Presented in part of Lecture of IAEA Expert Mission for Brazil, Sao Paulo, Brazil.
Martini, P., Boschi, A., Cicoria, G., Zagni, F., Corazza, A., Uccelli, L., et al. (2018). In-house cyclotron production of high-purity Tc-99m and Tc-99m radiopharmaceuticals. Appl. Radiat. Isot. 139, 325–331. doi:10.1016/j.apradiso.2018.05.033
Matthews, C. M. E., and Mallard, J. R. (1965). Distribution of 99mTc and tumor/brain concentrations in rats. J. Nucl. Med. 6 (6), 404–408. Available at: http://jnm.snmjournals.org/content/6/6/404.full.pdf+html.
Meléndez-Alafort, L., Ferro-Floresm, G., De Nardo, L., Bello, M., Paiusco, M., Negri, A., et al. (2019). Internal radiation dose assessment of radiopharmaceuticals prepared with cyclotron-produced 99m Tc. Med. Phys. 46 (3), 1437–1446. doi:10.1002/mp.13393
Metello, L. F. (2015). 99mTc-Technetium Shortage: Old Problems Asking for New Solutions. J. Med. Imaging Radiat. Sci. 46 (3), 256–261. doi:10.1016/j.jmir.2015.07.003
Mikaeili, A., Erfani, M., Shafiei, M., Kobarfard, F., Abdi, K., Sabzevari, O., et al. (2018). Development of a 99mTc-Labeled CXCR4 Antagonist Derivative as a New Tumor Radiotracer. Cancer biother. Radiopharm. 33 (1), 17–24. doi:10.1089/cbr.2017.2226
Miller, J. (1995). “Procedure for production of 99mTc sublimation generators based on eutectic SiC–MoO3–V2O5,” in Alternative technologies for 99mTc generators (Vienna: Internatoinal Atomic Energy Agency), 44–46.
Minh, T. L., and Lengyel, T. (1989). On the separation of molybdenum and technetium crown ether as extraction agent. J. Radioanal. Nucl. Chem. 135 (6), 403–407. doi:10.1007/BF02164767
Mitra, A., Chattopadhyay, S., Chandak, A., Lad, S., Baruaet, L., De, A., et al. (2020). Clinical Efficacy of Sodium [99mTc] Pertechnetate from Low Specific Activity 99Mo/99mTc Autosolex Generator in Hospital Radiopharmacy Centre. Nucl. Med. Rev. Cent. East. Eur. 23 (1), 1–14. doi:10.5603/NMR.a2020.0001
Molinski, V. J. (1982). A review of 99mTc generator technology. Int. J. Appl. Radiat. Isot. 33 (10), 811–819. doi:10.1016/0020-708X(82)90122-3
Monroy-Guzman, F., Diaz-Archundia, L. V., and Contreras, R. A. (2003). Effect of Zr:Mo ratio on 99mTc generator performance based on zirconium molybdate gels. Appl. Radiat. Isot. 59 (1), 27–34. doi:10.1016/s0969-8043(03)00150-7
Monroy-Guzman, F., Díaz-Archundia, L. V., and Hernández-Cortés, S. (2008). 99Mo/99mTc generators performances prepared from zirconium molybdate gels. J. Braz. Chem. Soc. 19, 380–388. doi:10.1590/S0103-50532008000300003
Monroy-Guzman, F., Romero, O. C., and Velázquez, H. D. (2007). Titanium molybdate gels as matrix of 99Mo/99mTc generators. J. Nucl. Radiochem. Sci. 8 (1), 11–19. doi:10.14494/jnrs2000.8.11
Moore, P. W., Shying, M. E., Sodeau, J. M., Evans, J. V., Maddalena, D. J., Farrington, K. H., et al. (1987). Zirconium molybdate gel as a generator for technetium-99m—II. High activity generators. Int. J. Radiat. Appl. Instrum. Part A. Appl. Radiat. Isotopes 38 (1), 25–29. doi:10.1016/0883-2889(87)90231-0
Morley, T. J., Dodd, M., Gagnon, K., Hanemaayer, V., Wilson, J., McQuarrie, S. A., et al. (2012). An automated module for the separation and purification of cyclotron-produced 99mTcO4−. Nucl. Med. Biol. 39 (4), 551–559. doi:10.1016/j.nucmedbio.2011.10.006
Mostafa, M., Saber, H. M., El-Sadek, A. A., and Nassar, M. Y. (2016). Preparation and performance of 99Mo/99mTc chromatographic column generator based on zirconium molybdosilicate. Radiochim. Acta 104 (4), 257–265. doi:10.1515/ract-2015-2488
Nagai, Y., and Hatsukawa, Y. (2009). Production of 99Mo for Nuclear Medicine by 100Mo(n, 2n)99Mo. J. Phys. Soc. Jpn. 78 (3), 033201. doi:10.1143/JPSJ.78.033201
NAP (2009). Medical Isotope Production without Highly Enriched Uranium. Washington D.C., U.S.A.: National Academies of Sciences, Engineering, and Medicine.
NAP (2016). Molybdenum-99 for Medical Imaging. Washington D.C., U.S.A.: National Academies of Sciences, Engineering, and Medicine.
NAP (2018). Opportunities and approaches for supplying molybdenum-99 and associated medical isotopes to global markets: proceedings of a symposium. Washington D.C., U.S.A.: National Academies of Sciences, Engineering, and Medicine.
Narasimhan, D. V. S., Vanaja, P., and Mani, R. S. (1984). A new method for 99mTc generator preparation. J. Radioanalytical Nucl. Chem. Lett. 85 (6), 345–355. doi:10.1007/BF02164058
Nawar, M. F. (2021). Development of a New Generation of 99mTc radionuclide generators Based on Low Specific Activity 99Mo. Ph.D. thesis (Bern, Switzerland: University of Bern).
Nawar, M. F., and Türler, A. (2019). “Development of New Generation of 99Mo/99mTc Radioisotope Generators to Meet the Continuing Clinical Demands,” in Presented in part at 2nd International Conference on Radioanalytical and Nuclear Chemistry (RANC 2019), Budapest, Hungary.
NEA (2017). Medical isotope supply review: 99Mo/99mTc market demand and production capacity projection 2017-2022. Nuclear Development NEA/SEN/HLGMR. Paris, France: Nuclear Energy Agency.
NEA (2019). The Supply of Medical Radioisotopes: 2019 Medical Isotope Demand and Capacity projection for the 2019-2024 period. Nuclear Development NEA/SEN/HLGMR. Paris, France: Nuclear Energy Agency. Available at: https://www.oecd-nea.org/med-radio/docs/sen-hlgmr2019-1.pdf.
NEA (2010). The supply of medical radioisotopes: review of potential molybdenum-99/technetium-99m production technologies. Nuclear Development NEA/SEN/HLGMR. Paris, France: Nuclear Energy Agency.
NEA (2011). The Supply of Medical Radioisotopes: the path to reliability, NEA No. 6985. Nuclear Development NEA/SEN/HLGMR. Paris, France: Nuclear Energy Agency. Available at: https://www.oecd-nea.org/med-radio/reports/med-radio-reliability.pdf.
Noronha, O. P. D. (1986). Solvent extraction technology of 99Mo–99mTc generator system. An Indian experience: Process design considerations. Isotopenpraxis Isotopes Environ. Health Stud. 22 (2), 53–57. doi:10.1080/10256018608623597
Northstar (2020). Licensing Guidance for Medical Use Licensees, Medical Use Permittees, and Commercial Nuclear Pharmacies. Available at: https://www.nrc.gov/docs/ML2000/ML20009C049.pdf.
Osso, J. A., Catanoso, M. F., Barrio, G., Brambilla, T. P., Teodoro, R., Dias, C. R. B. R., et al. (2012). Technetium-99m: New Production and Processing Strategies to Provide Adequate Levels for SPECT Imaging. Curr. Radiopharm. 5 (3), 178–186. doi:10.2174/1874471011205030178
Osso, J., and Knapp, F. F. (2011). Sampson’s Textbook of Radiopharmacy. 4th edition. London, U.K.: Pharmaceutical Press.
Owen, H. L., Manchester, U., Ballinger, J., Buscombe, J., Clarke, R., Denton, E., et al. (2014). “Options for U.K. Technetium-99m production using accelerators,” in Presented in part at 5th International Particle Accelerator Conference, Dresden, Germany. Available at: https://accelconf.web.cern.ch/IPAC2014/papers/thoab02.pdf.
Parihar, A, S., Singh, H., Bhansali, A., Karthikeyan, M. B. B. S., Vadi, S. K., and Parmar, M. (2021). Hepato-cardiac juxtaposition secondary to right hemi-diaphragmatic eventration on 99mTc-Sestamibi myocardial perfusion scintigraphy. J. Nucl. Cardiol. 28 (2), 750–751. doi:10.1007/s12350-020-02033-3
Paterson, A., Druce, M., and Killen, E. (2015). Six problems with the 6-day Curie and a solution. J. Radioanal. Nucl. Chem. 305 (1), 13–22. doi:10.1007/s10967-015-4050-4
Pavlović, M. M., Pavlović, M. R. P., Bartl, P., Stevanović, J., and Radak, B. (2020). Optimization of injected radiotracer volume for flow rate measurement in closed conduits. Hem. Ind. 74 (5), 305–312. doi:10.2298/HEMIND20050325P
Perrier, C., and Segrè, E. (1937). Some Chemical Properties of Element 43. J. Chem. Phys. 5 (9), 712–716. doi:10.1063/1.1750105
Pillai, M. R. A., Dash, A., and Knapp, F. F. (2013). Sustained Availability of 99mTc: Possible Paths Forward. J. Nucl. Med. 54 (2), 313–323. doi:10.2967/jnumed.112.110338
Pimlott, S. L., and Sutherland, A. (2011). Molecular tracers for the PET and SPECT imaging of disease. Chem. Soc. Rev. 40 (1), 149–162. doi:10.1039/b922628c
Qaim, S. M. (2012). The present and future of medical radionuclide production. Radiochim. Acta 100 (8-9), 635–651. doi:10.1524/ract.2012.1966
Qaim, S. M. (2019). Theranostic radionuclides: Recent advances in production methodologies. J. Radioanal. Nucl. Chem. 322 (3), 1257–1266. doi:10.1007/s10967-019-06797-y
Richards, P. (1965). The Technetium-99m Generator. Upton, NY, USA: BNL-9601 Brookhaven National Lab. Available at: https://www.osti.gov/servlets/purl/4589063.
Richards, P., Tucker, W. D., and Srivastava, S. C. (1982). Technetium-99m: An historical perspective. Int. J. Appl. Radiat. Isot. 33 (10), 793–799. Available at: https://inis.iaea.org/search/search.aspx?orig_q=RN:14755407.
Richards, V. N., Mebrahtu, E., and Lapi, S. E. (2013). Cyclotron production of 99mTc using 100Mo2C targets. Nucl. Med. Biol. 40 (7), 939–945. doi:10.1016/j.nucmedbio.2013.06.005
Rogers, R. D., Bond, A. H., Zhang, J., and Horwitz, E. P. (1997). New Technetium-99M Generator Technologies Utilizing Polyethylene Glycol-Based Aqueous Biphasic Systems. Sep. Sci. Technol. 32 (1–4), 867–882. doi:10.1080/01496399708003234
Ruth, T. J. (2009). Accelerating production of medical isotopes. Nature 457 (7229), 536–537. doi:10.1038/457536a
Ruth, T. J. (2014). The medical isotope crisis: How we got here and where we are going. J. Nucl. Med. Technol. 42 (4), 245–248. doi:10.2967/jnmt.114.144642
Ruth, T. J. (2020). The Shortage of Technetium-99m and Possible Solutions. Annu. Rev. Nucl. Part. Sci. 70, 77–94. doi:10.1146/annurev-nucl-032020-021829
Ryabchikov, A. I., Skuridin, V. S., Nesterov, E. V., Chibisov, E. V., and Golovkov, V. M. (2004). Obtaining molybdenum-99 in the IRT-T research reactor using resonance neutrons. Nucl. Instrum. Methods Phys. Res. Sect. B Beam Interact. Mater. Atoms 213, 364–368. doi:10.1016/S0168-583X(03)01592-1
Sakr, T. M., Nawar, M. F., Fasih, T. W., El-Bayoumy, S., and Abd El-Rehim, H. A. (20172017). Nano-Technology Contributions towards the Development of High Performance Radioisotope Generators: The Future Promise to Meet the Continuing Clinical Demand. Appl. Radiat. Isot. 129, 67–75. doi:10.1016/j.apradiso.2017.08.012
Sameh, A., and Ache, H. (1989). Production techniques of fission 99Mo. IAEA-TECDOC-515: Fission Molybdenum for Medical Use in Proceedings of a technical committee meeting, Inertnational Atomic Energy Agency (EAEA), Karlsruhe, Germany. Available at: https://www-pub.iaea.org/MTCD/Publications/PDF/te_515_prn.pdf.
Saraswathy, P., Sarkar, S. K., Arjun, G., Nandy, S. K., and Ramamoorthy, N. (2004). 99mTc gel generators based on zirconium molybdate-99Mo: III: Influence of preparatory conditions of zirconium molybdate-99Mo gel on generator performance. Radiochim. Acta 92 (4-6), 259–264. doi:10.1524/ract.92.4.259.35585
Sastre, A. M., Kumar, A., Shukla, J. P., and Singh, R. K. (1998). Improved techniques in liquid membrane separations: An overview. Sep. Purif. Methods 27 (2), 213–298. doi:10.1080/03602549809351641
Schmid, G. (2011). Nanoparticles: From theory to application. 2nd edition. New Jersey: John Wiley & Sons.
Sebastian, V., Arruebo, M., and Santamaria, J. (2014). Reaction engineering strategies for the production of inorganic nanomaterials. Small 10 (5), 835–853. doi:10.1002/smll.201301641
Sekimoto, S., Tatenuma, K., Suzuki, Y., Tsuguchi, A., Tanaka, A., Tadokoro, T., et al. (2017). Separation and purification of 99mTc from 99Mo produced by electron linear accelerator. J. Radioanal. Nucl. Chem. 311 (2), 1361–1366. doi:10.1007/s10967-016-4959-2
Selivanova, S. V., Lavallée, É., Senta, H., Caouette, L., Sader, J. A., van Lier, E. J., et al. (2015). Radioisotopic Purity of Sodium Pertechnetate 99mTc Produced with a Medium-Energy Cyclotron: Implications for Internal Radiation Dose, Image Quality, and Release Specifications. J. Nucl. Med. 56 (10), 1600–1608. doi:10.2967/jnumed.115.156398
Shafiq, Y. F., and Yousif, Z. M. (1995). Characteristics and behavior of a 99Mo/99mTc generator using irradiated titanium molybdate as column matrix. J. Radioanalytical Nucl. Chem. Lett. 199 (3), 173–181. doi:10.1007/BF02162365
Skuridin, V. S., and Chibisov, E. V. (2010). Development of a small-size extractor for separation of the 99Mo/99mTc couple. Radiochemistry 52 (1), 90–94. doi:10.1134/S1066362210010200
Stolarz, A., Kowalska, J. A., Jasiński, P., Janiak, T., and Samorajczyk, J. (2015). Molybdenum targets produced by mechanical reshaping. J. Radioanal. Nucl. Chem. 305 (3), 947–952. doi:10.1007/s10967-015-3956-1
Syarip, S., Sutondo, T., Budisantoso, E. T., and Susiantini, E. (2018). Design and Development of Subcritical Reactor by Using Aqueous Fuel for 99Mo Production. Proc. Pak. Acad. Sci. Pak. Acad. Sci. A Phys. Comput. Sci. 55 (1), 21–26.
Tachimori, S., Amano, H., and Nakamura, H. (1971). Preparation of Tc-99m by direct adsorption from organic solution. J. Nucl. Sci. Technol. 8 (7), 357–362. doi:10.1080/18811248.1971.9735346
Takács, S., Szűcs, Z., Tárkányi, F., Hermanne, A., and Sonck, M. (2003). Evaluation of proton induced reactions on 100Mo: New cross sections for production of 99mTc and 99Mo. J. Radioanal. Nucl. Chem. 257 (1), 195–201. doi:10.1023/A:1024790520036
Tarkanyi, F. T., Ignatyuk, A. V., Hermanne, A., Capote, R., Carlson, B. V., Engle, J. W., et al. (2019). Recommended nuclear data for medical radioisotope production: Diagnostic gamma emitters. J. Radioanal. Nucl. Chem. 319 (2), 487–531. doi:10.1007/s10967-018-6142-4
Taskaev, E., Taskaeva, M., and Nikolov, P. (1995). Extraction generator for [99mTc] sodium pertechnetate production. Appl. Radiat. Isot. 46 (1), 13–16. doi:10.1016/0969-8043(94)00098-K
Tatenuma, K., Ishikawa, K., Tsuguchi, A., Komatsuzaki, Y., Suzuki, Y., Tanaka, A., et al. (2014). A mass-production process of a highly pure medical use 99mTc from natural isotopic Mo(n, γ)99Mo without using uranium. Radioisotopes 63 (11), 501–513. doi:10.3769/radioisotopes.63.501
Tatenuma, K., Tatenuma, K., Tsuguchi, A., Suzuki, Y., Ishikawa, K., Sekimoto, S., et al. (2016b). “Generator of Highly Concentrated Pure 99mTc from Low Specific Activity 99Mo Produced by Reactor and/or Electron Linear Accelerator,” in Presented in part at Mo-99 Topical Meeting, Missouri, U.S.A. Available at: https://mo99.ne.anl.gov/2016/pdfs/papers/S9P9_Paper_Tatenuma.pdf.
Tatenuma, K., Ueda, T., Kurosawa, K., Ishikawa, K., Tanaka, A., Noguchi, T., et al. (2016a). Method of recovering enriched radioactive technetium and system therefor, U.S. Patent 9236153–B2.
Thyn, J., and Zitny, R. (2004). Radiotracer applications for the analysis of complex flow structure in industrial apparatuses. Nucl. Instrum. Methods Phys. Res. Sect. B Beam Interact. Mater. Atoms 213, 339–347. doi:10.1016/s0168-583x(03)01648-3
Tucker, W. D., Greene, M. W., Weiss, A. J., and Murrenhoff, A. (1958). Methods of Preparation of Some Carrier-Free Radioisotopes Involving Sorption on Alumina. Upton, NY, USA: BNL-3746, Brookhaven National Lab. doi:10.2172/4305138
Tur, Y. (2000). “Linear Electron Accelerator for the Medical Isotopes Production,” in Proceedings of EPAC 2000. Vienna: Austria. Available at: https://accelconf.web.cern.ch/e00/PAPERS/WEP2B12.pdf.
Türler, A., Vagheian, M., and Lüthi, M. (2021). High Power Converter Target Assembly, Related Facility and Method to Produce Bremsstrahlung for Photonuclear Reactions, EP21212627.0.
Urbano, N., Scimeca, M., Tancredi, V., Bonanno, E., and Schillaci, O. (2020). 99mTc-sestamibi breast imaging: Current status, new ideas and future perspectives. Semin. Cancer Biol. 84, 302–309. doi:10.1016/j.semcancer.2020.01.007
Vagehian, M. (2022). A point Source High Power Converter Target to Produce Bremsstrahlung for Photonuclear Reaction. Ph.D. thesis (Bern, Switzerland: University of Bern).
Vallabhajosula, S., Zimmerman, R. E., Picard, M., Stritzke, P., Mena, I., Heliman, R. S., et al. (1989). Technetium-99m ECD: A New Brain Imaging Agent: In-vivo Kinetics and Biodistribution Studies in Normal Human Subjects. J. Nucl. Med. 30 (5), 599–604.
Van der Marck, S, C., Koning, A. J., and Charlton, K. E. (2010). The options for the future production of the medical isotope 99Mo. Eur. J. Nucl. Med. Mol. Imaging 37, 1817–1820. doi:10.1007/s00259-010-1500-7
Vanaja, P., Ramamoorthy, N., Iyer, S. P., and Mani, R. S. (1987). Development of a New 99mTc Generator Using Neutron Irradiated Titanium Molybdate as Column Matrix. Radiochim. Acta. 42 (1), 49–52. doi:10.1524/ract.1987.42.1.49
Wu, D., Landsberger, S., Buchholz, B., and Vandegrift, G. F. (1995). “Processing of LEU targets for 99Mo production-testing and modification of the Cintichem process,” in Presented in part at International Meeting on Reduced Enrichment for Research and Test Reactors, Paris, France. Available at: http://www.rertr.anl.gov/MO99/WU95.pdf.
Yassine, T. (2000). Separation of 99mTc from 99Mo by Using TOPO-kerosene supported liquid membrane. J. Radioanal. Nucl. Chem. 246 (3), 665–669. doi:10.1023/A:1006700130611
Yousefi, T., Khanchi, A. R., Ahmadi, S. J., Rofouei, M. K., Yavari, R., Davarkhah, R., et al. (2012). Cerium(III) molybdate nanoparticles: Synthesis, characterization and radionuclides adsorption studies. J. Hazard. Mat. 215-216, 266–271. doi:10.1016/j.jhazmat.2012.02.064
Zaidi, J. H., Karim, H. M. A., Arif, M., Quershi, H. I., Qaim, S. M., and Stocklin, G. (1990). Fission Neutron Spectrum Averaged Cross Sections of some Threshold Reactions on Molybdenum: Estimation of Radioactive Impurities in (n, γ)-Produced 99Mo-99mTc Generator System. Radiochim. Acta. 49 (3), 107–112. doi:10.1524/ract.1990.49.3.107
Zhuikov, B. L. (2014). Production of medical radionuclides in Russia: Status and future — a review. Appl. Radiat. Isot. 84, 48–56. doi:10.1016/j.apradiso.2013.11.025
Zolle, I. (2007). Technetium-99m Radiopharmaceuticals: Preparation and Quality Control in Nuclear Medicine. Berlin, Germany: Springer.
Zsinka, L. (1987). 99mTc sublimation generators. Radiochim. Acta. 41 (2-3), 91–96. doi:10.1524/ract.1987.41.23.91
Keywords: 99mTc radiopharmaceuticals, 99Mo/99mTc generators, nano-materials, photonuclear reaction, electrochemical separation, 99Mo supply chain, solvent extraction, column chromatography
Citation: Nawar MF and Türler A (2022) New strategies for a sustainable 99mTc supply to meet increasing medical demands: Promising solutions for current problems. Front. Chem. 10:926258. doi: 10.3389/fchem.2022.926258
Received: 22 April 2022; Accepted: 27 June 2022;
Published: 22 July 2022.
Edited by:
Gordon James Thorogood, Australian Nuclear Science and Technology Organisation, AustraliaReviewed by:
Paul Ellison, University of Wisconsin-Madison, United StatesThies Thiemann, United Arab Emirates University, United Arab Emirates
Copyright © 2022 Nawar and Türler. This is an open-access article distributed under the terms of the Creative Commons Attribution License (CC BY). The use, distribution or reproduction in other forums is permitted, provided the original author(s) and the copyright owner(s) are credited and that the original publication in this journal is cited, in accordance with accepted academic practice. No use, distribution or reproduction is permitted which does not comply with these terms.
*Correspondence: Mohamed F. Nawar, bW9oYW1lZC5uYXdhckB1bmliZS5jaA==, bW9oYW1lZGZuYXdhckB5YWhvby5jb20=