- School of Mechanical and Materials Engineering, Washington State University, Pullman, WA, United States
The rechargeable lithium-oxygen (Li–O2) batteries have been considered one of the promising energy storage systems owing to their high theoretical energy density. As an alternative to Li−O2 batteries based on lithium peroxide (Li2O2) cathode, cycling Li−O2 batteries via the formation and decomposition of lithium hydroxide (LiOH) has demonstrated great potential for the development of practical Li−O2 batteries. However, the reversibility of LiOH-based cathode chemistry remains unclear at the fundamental level. Here, we review the recent advances made in Li−O2 batteries based on LiOH formation and decomposition, focusing on the reaction mechanisms occurring at the cathode, as well as the stability of Li anode and cathode binder. We also provide our perspectives on future research directions for high-performance, reversible Li−O2 batteries.
Introduction
Lithium–oxygen (Li−O2) batteries, also known as Li–air batteries, use lithium metal as an anode and Earth-abundant O2 as a cathode-active material (Figure 1A) (Aurbach et al., 2016). In general, Li−O2 batteries can be divided into two categories, depending on the type of electrolytes that separate cathode from anode, (ⅰ) aqueous and (ⅱ) non-aqueous Li−O2 batteries. Owing to the high reactivity of water toward Li metal anode, most studies focus on non-aqueous Li−O2 batteries. Non-aqueous Li−O2 batteries were first demonstrated by Abraham and Jiang (1996) using a polymer-based electrolyte. Since then, the research and development of Li−O2 batteries have blossomed (Aurbach et al., 2016; Chang et al., 2017; Lim et al., 2017; Song et al., 2017). Three types of battery chemistries have been reported, including 1e− lithium superoxide (LiO2) (Lu et al., 2016), 2e− lithium peroxide (Li2O2) (McCloskey et al., 2013), and 4e− lithium oxide (Li2O) and lithium hydroxide (LiOH) (Liu et al., 2015; Lei et al., 2022). The corresponding reactions are summarized below.
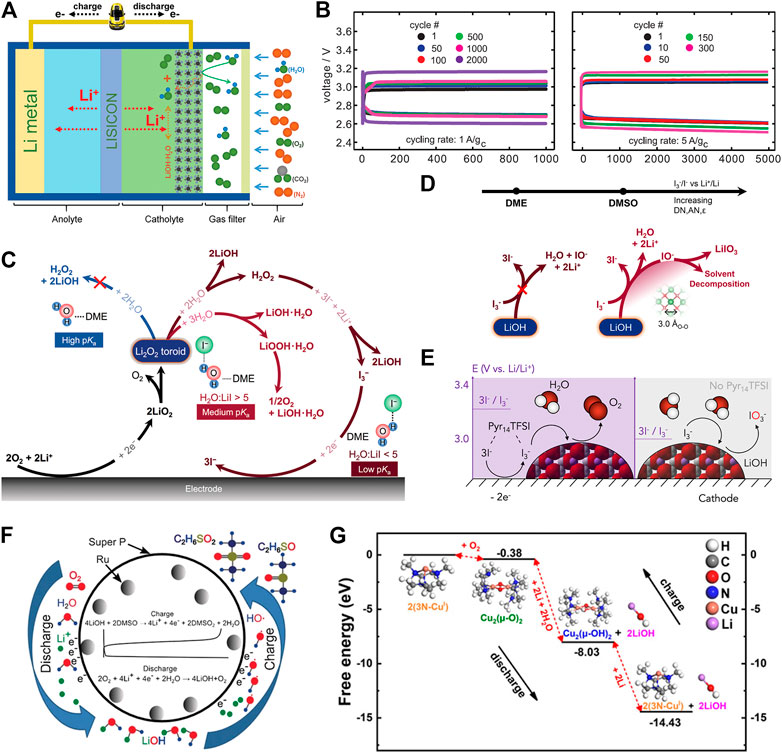
FIGURE 1. Representative Li−O2 batteries based on LiOH formation and decomposition. (A) Schematic illustration of Li−air batteries based on LiOH chemistry (Wu et al., 2016). (B) Cycling performance of Li−air batteries based on LiOH chemistry (Liu et al., 2015). (C) Reaction pathways of the formation of LiOH in LiI-containing electrolytes (Tulodziecki et al., 2017). (D) Solvent-dependent oxidation power of
Li2O2 is the most reported discharge product in non-aqueous Li−O2 batteries because, under ambient conditions, LiO2 is thermodynamically unstable against disproportionation (Zhang et al., 2017), and Li2O is thermodynamically unfavorable owing to a lower electrode potential of E0(O2/Li2O) = 2.91 V. It is also noted that Li2O can be thermodynamically more favorable over Li2O2 as discharge product in a molten-salt electrolyte at >150°C (Xia et al., 2018), which is beyond the scope of this study. When compared with Li2O2 chemistry, Li−O2 batteries based on LiOH chemistry can be operated in humid environments and demonstrate better resistance toward CO2 (Lei et al., 2022), which might enable the operation of Li−O2 batteries in the air. Liu et al. (2015) reported high-performance Li−O2 batteries based on LiOH formation and decomposition via iodide catalysis (Figure 1B). Since then, many research efforts have been devoted to LiOH chemistry (Table 1), which significantly advances the understanding of this chemistry.
Here, we present an overview of Li−O2 batteries based on the formation and decomposition of LiOH. This review begins with the fundamentals of the formation of LiOH, followed by the decomposition of LiOH. Furthermore, we discuss the stability of Li metal anode and cathode binder in brief. Finally, we provide the outlook on how to achieve reversible LiOH chemistry for advanced Li−O2 batteries.
Fundamentals of Lithium Hydroxide Formation at the Cathode
Reported Mechanisms on Discharge
The formation of LiOH in Li−O2 batteries is based on 4e− O2 reduction, which renders this process complicated. The first step involves O2 reduction to form a superoxide intermediate, that is, LiO2 or O2− (Eq. 5). In the following steps, several reaction intermediates and pathways have been reported in the literature, as summarized below.
1) Direct hydrolysis of LiO2 (Wang et al., 2017; Bi et al., 2020).
2) Hydrolysis of LiO2 to form LiHO2 intermediate, followed by disproportionation of LiHO2 (Qiao et al., 2017).
3) Disproportionation of LiO2 to form Li2O2 intermediate, followed by hydrolysis of Li2O2 (Peng et al., 2015; Tulodziecki et al., 2017).
The total reaction for each of the abovementioned three reaction pathways is the 4e− O2 reduction (Eq. 4), leading to LiOH as a discharge product. The reaction pathways depend on several factors, such as electrolytes, water content, and catalysts. The source of water and the role of catalysts are discussed in the following subsections. Representative LiOH systems are summarized in Table 1.
Source of Proton: Wet Electrolyte, Humid O2, or Water-Trapped Electrode
The formation of LiOH involves the hydrolysis of reaction intermediates, which requires the addition of water in Li−O2 cells. Water has been commonly introduced into either electrolyte (wet electrolyte) or O2 gas (humid O2). In early studies, however, the formation of LiOH was observed even in nominal dry electrolyte and dry O2 (Liu et al., 2015). This observation raises the question of where the proton comes from. In early studies, Kwak et al. (2015) and Qiao et al. (2017) reported that the source of the proton is the H-abstraction of electrolyte solvent, leading to electrolyte degradation and water formation. Later, Liu et al. (2019) demonstrated that the source of the proton is the water impurity in the electrolyte using isotope-labeled solid-state nuclear magnetic resonance (NMR) and Raman spectroscopy. We note that water impurity can be either from lithium salt or solvent, and thus the measurement of water content in electrolytes is highly recommended. Moreover, theoretical calculations also reveal that water is a much more favorable proton source than the electrolyte solvent (DME) (Torres and Balbuena, 2018). In addition to the water impurity in the electrolyte, water can be trapped in the cathode. Mu et al. (2019) reported that an activated graphene electrode can adsorb enough water (4.56%) for the formation of LiOH. A similar observation was also reported in the Ag/δ-MnO2 electrode (Dai et al., 2022).
We note that water might also come from the moisture in leaking cells, which is a common issue that causes the discrepancy in discharge capacity and morphology of discharge products in reported Li−O2 batteries. In leaking-free dry cells, film-like discharge products should be observed in low-donor-number and low-acceptor-number electrolytes (such as DME) (Aetukuri et al., 2015), which is a simple method to check if any leakage exists.
Role of Catalyst: Promotion of the Cleavage of O−O Bond
Unlike Li2O2, the formation of LiOH involves the cleavage of strong O−O bond (Eqs 8 and 11), which requires an effective catalyst to lower activation energy. Two types of catalysts have been widely studied, including soluble catalyst in the electrolyte and solid catalyst on the cathode. Representative catalysts are summarized in Table 1.
Soluble catalyst is also known as a redox mediator in Li−O2 batteries, and LiI is one of the most promising catalysts. Kwak et al. (2015) reported that LiI can facilitate the formation of LiOH via a proposed mechanism of iodide catalysis (Eqs 12 and 13).
Qiao et al. (2017) successfully detected the reaction intermediates of
Moreover, Tulodziecki et al. further demonstrated that low pKa of water in electrolyte facilitates Eq. 10 and, thus, is essential to the formation of LiOH. In LiI-containing electrolytes, high water content leads to high pKa of water (lower acidity) and thus sluggish hydrolysis of Li2O2 to H2O2 (Eq. 10). Overall, this lower acidity of water leads to apparent sluggish iodide catalysis and, thus, the formation of a Li2O2/LiOH mixture. Later, Liu et al. (2019) proposed the third explanation for sluggish iodide catalysis. In water-rich electrolytes (>5% H2O), I− ions are embedded in I−(H2O)n ion clusters, as revealed by MD simulations, which makes it difficult to be accessible for iodide catalysis, leading to sluggish iodide catalysis and, thus, the formation of Li2O2/LiOH mixture. In addition to Eq. 15, Liu et al. observed an alternative path for regeneration of I− from
Wang et al. (2020) reported a bio-inspired 3N-CuⅠ enzyme as a soluble catalyst for LiOH chemistry, which is effective for the cleavage of O−O bond (Figure 1G). The reaction intermediate is detected as Cu2(μ-O)2 complex by UV-Vis spectroscopy.
In addition to the abovementioned soluble catalysts, solid catalysts on the cathode have been demonstrated to be effective for the formation of LiOH. Peng et al. (2015) reported Ru@SP/MnO2 catalyst for LiOH chemistry, where MnO2 is effective for catalytic decomposition of H2O2 (Eq. 11). Since then, a few other cathode catalysts have been reported, such as Mn-MOF-74, Co-MOF, and Co3O4 (Kim et al., 2018; Yuan et al., 2019; Zhang et al., 2019; Lu et al., 2020). In our previous study, the authors reported that the Mn-MOF-74 catalyst was still active even if it was coated on a separator that was electrically isolated from the cathode (Zhang et al., 2019), demonstrating that the formation of LiOH can proceed via chemical hydrolysis of reaction intermediates.
Fundamentals of Lithium Hydroxide Decomposition at the Cathode
Reported Mechanisms on Charge
The decomposition of LiOH on charge is much more complex but less explored than the formation of LiOH. This process varies with catalyst and electrolyte, and it usually involves irreversible parasitic reactions. Some reported mechanisms are summarized below.
1) O2 evolution with a solid catalyst, for example, Ru and Co3O4 (Peng et al., 2015; Lu et al., 2020).
2) Irreversible formation of hydroxyl radical (OH·) that degrades electrolyte with a solid catalyst (Liu et al., 2017; Tang et al., 2022).
3) O2 evolution with redox mediator, for example, LiI (Liu et al., 2015; Temprano et al., 2020).
4) Irreversible formation of IO3− after Eq. 20 in LiI-containing electrolyte (Leverick et al., 2019).
Given the complexity of LiOH chemistry, systematic studies are needed to confirm its reversibility, which is discussed in the following subsections.
Reversibility and Parasitic Reactions
The reversibility of LiOH chemistry is defined as how much O2 evolves back to air during charge, which is different from “rechargeability” that typically features by the removal of discharge product. We emphasize that the removal of LiOH after charge does not guarantee good reversibility because it might be due to parasitic reactions (Eqs 19 and 22).
In early studies, Meini et al. (2013) demonstrated that pre-loaded LiOH cannot be decomposed to O2 with or without Pt catalyst in diglyme electrolyte by differential electrochemical mass spectroscopy (DEMS). Moreover, theoretical calculations suggest that oxidation of LiOH needs high overpotential and is controlled by the extraction of Li+ to form an O-rich surface at 4.6–5.0 V (vs. Li+/Li) (Ling et al., 2014). Later, Peng et al. (2015) reported that LiOH can be decomposed to O2 by Ru catalyst in DMSO electrolyte via Eq. 17, as revealed by gas chromatography (GC). However, Liu et al. (2017) demonstrated that oxidation of LiOH in DMSO electrolyte evolves little O2 using DEMS and operando cell pressure measurements, revealing the irreversibility of LiOH chemistry. This irreversibility was ascribed to the formation of hydroxyl radical that attacks the DMSO electrolyte via Eqs 18 and 19 (Figure 1F). Tang et al. (2022) further detected hydroxyl radical using a radical trap by in situ UV-Vis spectroscopy. Yang et al. (2016) also revealed the irreversibility of pre-loaded Li18OH in DMSO electrolytes by isotope-labeled DEMS. We note that pre-loaded LiOH typically shows a high charge potential at ∼5 V (vs. Li+/Li), at which electrolyte might undergo severe electromechanical oxidation.
In addition to solid catalysts, soluble catalysts have also been reported to facilitate the reversible oxidation of LiOH. In early studies, Liu et al. (2015) reported that LiOH can be decomposed to O2 by iodide catalysis at ∼3.0 V (vs. Li+/Li) in DME-based electrolytes via Eqs 20 and 21, as revealed by mass spectrometry. However, Viswanathan et al. (2016) argued that the oxidation of LiOH to O2 (E0 = 3.34 V vs. Li+/Li) by
Shen et al. (2016) and Qiao et al. (2017) demonstrated that LiOH cannot be oxidized by
Routes Toward Reversible Lithium Hydroxide Chemistry
The reversible 4e− oxidation of LiOH to O2 involves the reformation of O−O bond, which generates many reactive intermediates (reactive oxygen species). This process is feasible in an aqueous solution but is very challenging in organic electrolytes, owing to the instability of organic electrolytes toward these reactive intermediates. Therefore, less-reactive intermediates or more stable electrolytes are required to achieve reversible LiOH chemistry.
Given that amorphous Li2O2 may be formed simultaneously with LiOH on discharge, regular DEMS cannot differentiate from which O2 is evolved: Li2O2 or LiOH. As such, the isotope-labeled DEMS is recommended to exclusively determine whether LiOH is reversibly oxidized to O2 on charge. For experimental designs, isotope-labeled DEMS can be achieved by ether labelling O2 (18O2 or 17O2) or labelling H2O (H217O or H218O). An example of labelling H2O by H217O is shown in Eqs 25 and 26. If LiOH can be reversibly oxidized to O2, a mixture of 16O2, 16O17O, and 17O2 should be observed using DEMS.
Recently, Temprano et al. (2020) reported that truly reversible oxidation of LiOH was achieved by
Recently, Lu et al. (2020) reported that the Co3O4 catalyst can facilitate the reversible oxidation of LiOH to evolve O2, as revealed by isotope-labeled DEMS (H217O). This new finding suggests that the catalyst governs the reactivity of intermediates during the oxidation of LiOH, although more systematic studies are needed to understand why reactive hydroxyl species were not formed in this system. Wang et al. (2020) reported that reversible oxidation of LiOH was achieved by bio-inspired 3N-CuⅠ complex (Figure 1G), as revealed by DEMS and DFT calculations. The reversible LiOH chemistry was attributed to the effective cleavage and reformation of O−O bond by 3N-CuⅠ complex. However, we note that reactive oxygen species is embedded in the 3N-CuⅠ complex (Figure 1G), which might make it difficult to attack organic electrolyte, leading to reversible oxidation of LiOH to O2. In addition, we note that the 3N-CuⅠ complex was prepared by CuI, and thus the role of I− anions should be carefully studied in the future. Nonetheless, this new finding demonstrates the great potential of using bio-inspired complex to deactivate reactive oxygen species, which deserves more effort to explore.
Li Metal Anode and Cathode Binder
Stability of Li Anode
Lithium metal anodes have been studied intensely since the 1970s, owing to their ultralow reduction potential of −3.04 V (vs. SHE) and ultrahigh specific capacity of 3,860 mAh g−1 (Cheng et al., 2017). However, their practical application has been hindered owing to (ⅰ) safety concerns caused by dendritic lithium growth and (ⅱ) poor Coulombic efficiency caused by dead lithium and continuous solid electrolyte interphase (SEI) formation (Lu et al., 2011; Qian et al., 2015). During repeated Li plating/stripping processes, the huge mechanical stress generated from the infinite volume change of lithium results in the cracking of the SEI layer, leading to continuous SEI formation and dendritic lithium growth. Moreover, the large concentration gradient of Li+ ions at high current density can significantly accelerate dendritic lithium growth, leading to safety hazards. Substantial research effort has been devoted to stabilizing the lithium metal surface using electrolyte additives and/or an artificial SEI layer. In addition, solid-state electrolytes have emerged to address safety concerns by eliminating flammable organic liquid electrolytes (Manthiram et al., 2017). Recently, it has been reported that cycling Li metal in O2 is much more stable when compared with that in argon, suggesting that O2 is good for robust SEI formation (Qiu et al., 2018). Furthermore, cycling Li metal in CO2 could generate a robust Li2CO3-rich SEI layer, leading to a dendrite-free and moisture-tolerant Li metal anode (Asadi et al., 2018; Chen et al., 2020; Qiu et al., 2020).
Owing to its high reactivity, Li metal anode can severely react with water or some redox mediators in Li−O2 batteries (Guo et al., 2014), leading to parasitic reactions. Therefore, protection of Li metal anode is needed for LiOH chemistry. Recently, water-proof Li metal anodes have been developed, such as wax-infiltrated solid-state electrolyte layer (Wu et al., 2017; Lei et al., 2022). Besides the protection of Li anode, alternative reference electrodes have been developed for studying the effect of water or redox mediators on battery chemistry and performance. The lithium iron phosphate (LiFePO4) electrode has a redox potential of 3.45 V (vs. Li+/Li) with fast reaction kinetics, and thus has been widely used as alternative reference anode when Li metal anode is unstable (Li et al., 2015; Peng et al., 2015; Mahne et al., 2017; Ma et al., 2018). However, it has been reported that LiFePO4 electrode still could electrochemically react with some redox mediators such as tetrathiafulvalene (TTF) (Yang et al., 2016). Therefore, the reactivity of reference electrode with cell components should be carefully examined when selecting a reference electrode.
Stability of Cathode Binders: Polyvinylidene Fluoride and Polytetrafluoroethylene
A binder is essential for holding active materials on current collect when fabricating electrodes. Two binders are commonly used in Li−O2 batteries, polyvinylidene fluoride (PVdF) and polytetrafluoroethylene (PTFE). It has been reported that PVdF is unstable toward LiO2 and LiOH, leading to defluorination (Black et al., 2012; Papp et al., 2017). Therefore, the PTFE binder is encouraged in studies for LiOH chemistry.
Summary and Outlook
LiOH chemistry is promising for advanced Li−O2 batteries that can be operated in humid environments, considering that the requirements for removing moisture from the air down to a few ppm levels in conventional Li−O2 batteries (based on Li2O2) not only increase the cost but also reduce energy density (Gallagher et al., 2014). Moreover, cycling Li−O2 batteries based on LiOH chemistry has demonstrated great energy efficiency and rate capability (Figure 1B) because of soluble intermediates in wet electrolytes and mitigated passivation of the cathode. Since 2015, many research efforts have been devoted to LiOH chemistry. During discharge, the formation of LiOH involves the cleavage of O−O bond by the catalyst and the hydrolysis of reaction intermediates. During charge, the decomposition of LiOH is very complex because of parasitic reactions. The reversible oxidation of LiOH involves the reformation of O−O bond, which may generate many reactive oxygen species that attack organic electrolytes, including redox mediators. In early studies, many reports demonstrated that LiOH chemistry is irreversible in organic electrolytes and O2 evolution is lacking during charge. Markedly, a few recent studies have demonstrated that reversible LiOH chemistry can be achieved by tuning the oxidation power of the redox mediator or deactivating the reactive intermediates. Nonetheless, LiOH chemistry is still at an infant stage. There is still a lack of fundamental understanding of reaction mechanisms, which is essential for achieving truly reversible LiOH chemistry. In addition, most studies by far were performed in ultrapure O2, which is not practical for commercial applications of Li−O2 batteries. Moreover, the use of Li metal anode in Li−O2 batteries may pose a safety concern and low Columbic efficiency issue owing to the uncontrolled dendrite growth of Li and continuous SEI formation (side reactions). Furthermore, an excess of Li metal anode is used in most studies, which significantly decreases the practical energy density of Li−O2 batteries. We, therefore, present the research directions to achieve the reversible LiOH chemistry in Li−O2 batteries as follows.
1) Given the complexity of LiOH chemistry, we encourage systematic studies of reaction mechanisms by combining advanced experimental characterizations such as in situ surface-enhanced Raman spectroscopy and isotope-labeled DEMS with theoretical studies such as DFT calculations and MD simulations. In addition, it is necessary to study the effects of solvents, content of water, and catalysts on reaction mechanisms and electrochemical performance.
2) We encourage systematic studies on revealing the limiting factors that hinder the reversible oxidation of LiOH via 4e− O2 evolution reaction, which will enable the rational design of effective redox mediators or solid catalysts. The reactive intermediates during discharge and charge should be identified, and their reactivity toward degradation of cell components should be carefully evaluated. Moreover, the isotope-labeled DEMS is highly recommended in order to evaluate the reversibility of LiOH chemistry to rule out the false O2 signal from Li2O2 decomposition or leaking cells.
3) We encourage the development of novel catalysts that can mitigate the reactive intermediates such as surface hydroxyl species while maintaining effective catalytic activity toward cleavage and reformation of O−O bond. A bio-inspired metal complex might be one of the promising catalysts for deactivating reactive intermediates toward reversible LiOH chemistry.
4) We encourage systematic studies of cycling Li−O2 batteries in ambient air. The effects of N2, CO2, and moisture on LiOH chemistry and Li metal anode should be carefully studied to fully address their detrimental effects.
5) We encourage the development of Li metal anode protection technologies to address safety issues and low Columbic efficiency caused by the uncontrolled dendrite growth of Li and continuous SEI formation. To be practical, a thin Li metal anode (small excess of Li) should be evaluated for advanced Li−O2 batteries.
Author Contributions
XZ wrote the first draft of the manuscript. All authors contributed to manuscript revision, read, and approved the submitted version.
Funding
This work was financially supported by the Hyundai NGV’s Academy Industry Research Collaboration program.
Conflict of Interest
The authors declare that the research was conducted in the absence of any commercial or financial relationships that could be construed as a potential conflict of interest.
Publisher’s Note
All claims expressed in this article are solely those of the authors and do not necessarily represent those of their affiliated organizations, or those of the publisher, the editors, and the reviewers. Any product that may be evaluated in this article, or claim that may be made by its manufacturer, is not guaranteed or endorsed by the publisher.
Acknowledgments
PD acknowledges the China Scholarship Council (CSC) for the financial support.
References
Abraham, K. M., and Jiang, Z. (1996). A Polymer Electrolyte‐Based Rechargeable Lithium/Oxygen Battery. J. Electrochem. Soc. 143 (1), 1–5. doi:10.1149/1.1836378
Aetukuri, N. B., McCloskey, B. D., García, J. M., Krupp, L. E., Viswanathan, V., and Luntz, A. C. (2015). Solvating Additives Drive Solution-Mediated Electrochemistry and Enhance Toroid Growth in Non-aqueous Li-O2 Batteries. Nat. Chem. 7 (1), 50–56. doi:10.1038/nchem.2132
Asadi, M., Sayahpour, B., Abbasi, P., Ngo, A. T., Karis, K., Jokisaari, J. R., et al. (2018). A Lithium-Oxygen Battery with a Long Cycle Life in an Air-like Atmosphere. Nature 555 (7697), 502–506. doi:10.1038/nature25984
Aurbach, D., McCloskey, B. D., Nazar, L. F., and Bruce, P. G. (2016). Advances in Understanding Mechanisms Underpinning Lithium-Air Batteries. Nat. Energy 1 (9), 16128. doi:10.1038/nenergy.2016.128
Bi, X., Li, M., Liu, C., Yuan, Y., Wang, H., Key, B., et al. (2020). Cation Additive Enabled Rechargeable LiOH‐Based Lithium-Oxygen Batteries. Angew. Chem. Int. Ed. 59 (51), 22978–22982. doi:10.1002/anie.202010745
Black, R., Oh, S. H., Lee, J.-H., Yim, T., Adams, B., and Nazar, L. F. (2012). Screening for Superoxide Reactivity in Li-O2 Batteries: Effect on Li2O2/LiOH Crystallization. J. Am. Chem. Soc. 134 (6), 2902–2905. doi:10.1021/ja2111543
Burke, C. M., Black, R., Kochetkov, I. R., Giordani, V., Addison, D., Nazar, L. F., et al. (2016). Implications of 4 E- Oxygen Reduction via Iodide Redox Mediation in Li-O2 Batteries. ACS Energy Lett. 1 (4), 747–756. doi:10.1021/acsenergylett.6b00328
Chang, Z., Xu, J., and Zhang, X. (2017). Recent Progress in Electrocatalyst for Li-O2Batteries. Adv. Energy Mat. 7 (23), 1700875. doi:10.1002/aenm.201700875
Chen, K., Huang, G., Ma, J. L., Wang, J., Yang, D. Y., Yang, X. Y., et al. (2020). The Stabilization Effect of CO 2 in Lithium-Oxygen/CO 2 Batteries. Angew. Chem. Int. Ed. 59 (38), 16661–16667. doi:10.1002/anie.202006303
Cheng, X.-B., Zhang, R., Zhao, C.-Z., and Zhang, Q. (2017). Toward Safe Lithium Metal Anode in Rechargeable Batteries: a Review. Chem. Rev. 117 (15), 10403–10473. doi:10.1021/acs.chemrev.7b00115
Dai, L., Sun, Q., Yao, Y., Guo, H., Nie, X., Li, J., et al. (2022). Reversible LiOH Chemistry in Li-O2 Batteries with Free-Standing Ag/δ-MnO2 Nanoflower Cathode. Sci. China Mater. 65 (6), 1431–1442. doi:10.1007/s40843-021-1929-5
Gallagher, K. G., Goebel, S., Greszler, T., Mathias, M., Oelerich, W., Eroglu, D., et al. (2014). Quantifying the Promise of Lithium-Air Batteries for Electric Vehicles. Energy Environ. Sci. 7 (5), 1555–1563. doi:10.1039/c3ee43870h
Guo, Z., Dong, X., Yuan, S., Wang, Y., and Xia, Y. (2014). Humidity Effect on Electrochemical Performance of Li-O2 Batteries. J. Power Sources 264, 1–7. doi:10.1016/j.jpowsour.2014.04.079
Kim, H., Kwak, W.-J., Jung, H.-G., and Sun, Y.-K. (2020). Limited Effects of a Redox Mediator in Lithium-Oxygen Batteries: Indecomposable By-Products. J. Mat. Chem. A 8 (11), 5622–5628. doi:10.1039/c9ta13612f
Kim, S. H., Lee, Y. J., Kim, D. H., and Lee, Y. J. (2018). Bimetallic Metal-Organic Frameworks as Efficient Cathode Catalysts for Li-O2 Batteries. ACS Appl. Mat. Interfaces 10 (1), 660–667. doi:10.1021/acsami.7b15499
Kwak, W.-J., Hirshberg, D., Sharon, D., Shin, H.-J., Afri, M., Park, J.-B., et al. (2015). Understanding the Behavior of Li-Oxygen Cells Containing LiI. J. Mat. Chem. A 3 (16), 8855–8864. doi:10.1039/c5ta01399b
Lei, J., Gao, Z. Y., Tang, L. B., Zhong, L., Li, J. J., Zhang, Y., et al. (2022). Coupling Water-Proof Li Anodes with LiOH-Based Cathodes Enables Highly Rechargeable Lithium-Air Batteries Operating in Ambient Air. Adv. Sci. 9 (4), 13. doi:10.1002/advs.202103760
Leverick, G., Tułodziecki, M., Tatara, R., Bardé, F., and Shao-Horn, Y. (2019). Solvent-Dependent Oxidizing Power of LiI Redox Couples for Li-O2 Batteries. Joule 3 (4), 1106–1126. doi:10.1016/j.joule.2018.12.014
Li, F., Wu, S., Li, D., Zhang, T., He, P., Yamada, A., et al. (2015). The Water Catalysis at Oxygen Cathodes of Lithium-Oxygen Cells. Nat. Commun. 6, 7843. doi:10.1038/ncomms8843
Lim, H.-D., Lee, B., Bae, Y., Park, H., Ko, Y., Kim, H., et al. (2017). Reaction Chemistry in Rechargeable Li-O2batteries. Chem. Soc. Rev. 46 (10), 2873–2888. doi:10.1039/c6cs00929h
Ling, C., Zhang, R., Takechi, K., and Mizuno, F. (2014). Intrinsic Barrier to Electrochemically Decompose Li2CO3 and LiOH. J. Phys. Chem. C 118 (46), 26591–26598. doi:10.1021/jp5093306
Liu, T., Kim, G., Carretero-González, J., Castillo-Martínez, E., Bayley, P. M., Liu, Z., et al. (2016). Response to Comment on "Cycling Li-O 2 Batteries via LiOH Formation and Decomposition". Science 352 (6286), 667. doi:10.1126/science.aad8843
Liu, T., Kim, G., Jónsson, E., Castillo-Martinez, E., Temprano, I., Shao, Y., et al. (2019). Understanding LiOH Formation in a Li-O2 Battery with LiI and H2O Additives. ACS Catal. 9 (1), 66–77. doi:10.1021/acscatal.8b02783
Liu, T., Leskes, M., Yu, W., Moore, A. J., Zhou, L., Bayley, P. M., et al. (2015). Cycling Li-O 2 Batteries via LiOH Formation and Decomposition. Science 350 (6260), 530–533. doi:10.1126/science.aac7730
Liu, T., Liu, Z., Kim, G., Frith, J. T., Garcia-Araez, N., and Grey, C. P. (2017). Understanding LiOH Chemistry in a Ruthenium-Catalyzed Li-O2 Battery. Angew. Chem. Int. Ed. 56 (50), 16057–16062. doi:10.1002/anie.201709886
Lu, J., Dey, S., Temprano, I., Jin, Y., Xu, C., Shao, Y., et al. (2020). Co3O4-Catalyzed LiOH Chemistry in Li-O2 Batteries. ACS Energy Lett. 5 (12), 3681–3691. doi:10.1021/acsenergylett.0c01940
Lu, J., Jung Lee, Y., Luo, X., Chun Lau, K., Asadi, M., Wang, H.-H., et al. (2016). A Lithium-Oxygen Battery Based on Lithium Superoxide. Nature 529 (7586), 377–382. doi:10.1038/nature16484
Lu, Y.-C., Kwabi, D. G., Yao, K. P. C., Harding, J. R., Zhou, J., Zuin, L., et al. (2011). The Discharge Rate Capability of Rechargeable Li-O2 Batteries. Energy Environ. Sci. 4 (8), 2999–3007. doi:10.1039/c1ee01500a
Ma, S., Wang, J., Huang, J., Zhou, Z., and Peng, Z. (2018). Unveiling the Complex Effects of H2O on Discharge-Recharge Behaviors of Aprotic Lithium-O2 Batteries. J. Phys. Chem. Lett. 9 (12), 3333–3339. doi:10.1021/acs.jpclett.8b01333
Mahne, N., Schafzahl, B., Leypold, C., Leypold, M., Grumm, S., Leitgeb, A., et al. (2017). Singlet Oxygen Generation as a Major Cause for Parasitic Reactions during Cycling of Aprotic Lithium-Oxygen Batteries. Nat. Energy 2 (5), 1–9. doi:10.1038/nenergy.2017.36
Manthiram, A., Yu, X., and Wang, S. (2017). Lithium Battery Chemistries Enabled by Solid-State Electrolytes. Nat. Rev. Mat. 2 (4), 16103. doi:10.1038/natrevmats.2016.103
McCloskey, B. D., Valery, A., Luntz, A. C., Gowda, S. R., Wallraff, G. M., Garcia, J. M., et al. (2013). Combining Accurate O2 and Li2O2 Assays to Separate Discharge and Charge Stability Limitations in Nonaqueous Li-O2 Batteries. J. Phys. Chem. Lett. 4 (17), 2989–2993. doi:10.1021/jz401659f
Meini, S., Tsiouvaras, N., Schwenke, K. U., Piana, M., Beyer, H., Lange, L., et al. (2013). Rechargeability of Li-Air Cathodes Pre-filled with Discharge Products Using an Ether-Based Electrolyte Solution: Implications for Cycle-Life of Li-Air Cells. Phys. Chem. Chem. Phys. 15 (27), 11478–11493. doi:10.1039/c3cp51112j
Mu, X. W., Jiang, J., Deng, H., Qiao, Y., Zheng, M. B., Zhang, X. P., et al. (2019). H2O Self-Trapping Air Cathode of Li-O2 Battery Enabling Low Charge Potential Operating in Dry System. Nano Energy 64, 7. doi:10.1016/j.nanoen.2019.103945
Papp, J. K., Forster, J. D., Burke, C. M., Kim, H. W., Luntz, A. C., Shelby, R. M., et al. (2017). Poly(vinylidene Fluoride) (PVDF) Binder Degradation in Li-O2 Batteries: A Consideration for the Characterization of Lithium Superoxide. J. Phys. Chem. Lett. 8 (6), 1169–1174. doi:10.1021/acs.jpclett.7b00040
Peng, Z., Chen, Y., Bruce, P. G., and Xu, Y. (2015). Direct Detection of the Superoxide Anion as a Stable Intermediate in the Electroreduction of Oxygen in a Non-aqueous Electrolyte Containing Phenol as a Proton Source. Angew. Chem. Int. Ed. 54 (28), 8165–8168. doi:10.1002/anie.201502039
Qian, J., Henderson, W. A., Xu, W., Bhattacharya, P., Engelhard, M., Borodin, O., et al. (2015). High Rate and Stable Cycling of Lithium Metal Anode. Nat. Commun. 6, 6362. doi:10.1038/ncomms7362
Qiao, Y., Wu, S., Sun, Y., Guo, S., Yi, J., He, P., et al. (2017). Unraveling the Complex Role of Iodide Additives in Li-O2 Batteries. ACS Energy Lett. 2 (8), 1869–1878. doi:10.1021/acsenergylett.7b00462
Qiu, F., Ren, S., Mu, X., Liu, Y., Zhang, X., He, P., et al. (2020). Towards a Stable Li-CO2 Battery: The Effects of CO2 to the Li Metal Anode. Energy Storage Mater. 26, 443–447. doi:10.1016/j.ensm.2019.11.017
Qiu, F., Zhang, X., Qiao, Y., Zhang, X., Deng, H., Shi, T., et al. (2018). An Ultra-stable and Enhanced Reversibility Lithium Metal Anode with a Sufficient O2 Design for Li-O2 Battery. Energy Storage Mater. 12, 176–182. doi:10.1016/j.ensm.2017.12.011
Shen, Y., Zhang, W., Chou, S.-L., and Dou, S.-X. (2016). Comment on "Cycling Li-O 2 Batteries via LiOH Formation and Decomposition". Science 352 (6286), 667. doi:10.1126/science.aaf1399
Song, H., Deng, H., Li, C., Feng, N., He, P., and Zhou, H. (2017). Advances in Lithium-Containing Anodes of Aprotic Li-O2Batteries: Challenges and Strategies for Improvements. Small Methods 1 (8), 1700135. doi:10.1002/smtd.201700135
Sun, B., Guo, L., Ju, Y., Munroe, P., Wang, E., Peng, Z., et al. (2016). Unraveling the Catalytic Activities of Ruthenium Nanocrystals in High Performance Aprotic Li-O2 Batteries. Nano Energy 28, 486–494. doi:10.1016/j.nanoen.2016.08.057
Tang, L., Li, J., Zhang, Y., Gao, Z., Chen, J., and Liu, T. (2022). Unraveling the Reaction Interfaces and Intermediates of Ru-Catalyzed LiOH Decomposition in DMSO-Based Li-O2 Batteries. J. Phys. Chem. Lett. 13 (2), 471–478. doi:10.1021/acs.jpclett.1c03470
Temprano, I., Liu, T., Petrucco, E., Ellison, J. H. J., Kim, G., Jónsson, E., et al. (2020). Toward Reversible and Moisture-Tolerant Aprotic Lithium-Air Batteries. Joule 4 (11), 2501–2520. doi:10.1016/j.joule.2020.09.021
Torres, A. E., and Balbuena, P. B. (2018). Exploring the LiOH Formation Reaction Mechanism in Lithium-Air Batteries. Chem. Mat. 30 (3), 708–717. doi:10.1021/acs.chemmater.7b04018
Tulodziecki, M., Leverick, G. M., Amanchukwu, C. V., Katayama, Y., Kwabi, D. G., Barde, F., et al. (2017). The Role of Iodide in the Formation of Lithium Hydroxide in Lithium-Oxygen Batteries. Energy Environ. Sci. 10 (8), 1828–1842. doi:10.1039/C7EE00954B
Viswanathan, V., Pande, V., Abraham, K. M., Luntz, A. C., McCloskey, B. D., and Addison, D. (2016). Comment on "Cycling Li-O 2 Batteries via LiOH Formation and Decomposition". Science 352 (6286), 667. doi:10.1126/science.aad8689
Wang, B., Fielding, A. J., and Dryfe, R. A. W. (2019). Electron Paramagnetic Resonance Investigation of the Structure of Graphene Oxide: pH-Dependence of the Spectroscopic Response. ACS Appl. Nano Mat. 2 (1), 19–27. doi:10.1021/acsanm.8b01329
Wang, C., Zhang, Z., Liu, W., Zhang, Q., Wang, X. G., Xie, Z., et al. (2020). Enzyme‐Inspired Room‐Temperature Lithium-Oxygen Chemistry via Reversible Cleavage and Formation of Dioxygen Bonds. Angew. Chem. Int. Ed. 59 (41), 17856–17863. doi:10.1002/anie.202009792
Wang, H.-H., Lee, Y. J., Assary, R. S., Zhang, C., Luo, X., Redfern, P. C., et al. (2017). Lithium Superoxide Hydrolysis and Relevance to Li-O2 Batteries. J. Phys. Chem. C 121 (18), 9657–9661. doi:10.1021/acs.jpcc.6b12950
Wu, S., Tang, J., Li, F., Liu, X., Yamauchi, Y., Ishida, M., et al. (2016). A Synergistic System for Lithium-Oxygen Batteries in Humid Atmosphere Integrating a Composite Cathode and a Hydrophobic Ionic Liquid-Based Electrolyte. Adv. Funct. Mat. 26 (19), 3291–3298. doi:10.1002/adfm.201505420
Wu, S., Yi, J., Zhu, K., Bai, S., Liu, Y., Qiao, Y., et al. (2017). A Super-hydrophobic Quasi-Solid Electrolyte for Li-O2Battery with Improved Safety and Cycle Life in Humid Atmosphere. Adv. Energy Mat. 7 (4), 1601759. doi:10.1002/aenm.201601759
Xia, C., Kwok, C. Y., and Nazar, L. F. (2018). A High-Energy-Density Lithium-Oxygen Battery Based on a Reversible Four-Electron Conversion to Lithium Oxide. Science 361 (6404), 777–781. doi:10.1126/science.aas9343
Yang, H., Wang, Q., Zhang, R., Trimm, B. D., and Whittingham, M. S. (2016). The Electrochemical Behaviour of TTF in Li-O2 Batteries Using a TEGDME-Based Electrolyte. Chem. Commun. 52 (48), 7580–7583. doi:10.1039/c6cc01120a
Yuan, M., Wang, R., Fu, W., Lin, L., Sun, Z., Long, X., et al. (2019). Ultrathin Two-Dimensional Metal-Organic Framework Nanosheets with the Inherent Open Active Sites as Electrocatalysts in Aprotic Li-O2 Batteries. ACS Appl. Mat. Interfaces 11 (12), 11403–11413. doi:10.1021/acsami.8b21808
Zhang, X., Dong, P., Lee, J.-I., Gray, J. T., Cha, Y.-H., Ha, S., et al. (2019). Enhanced Cycling Performance of Rechargeable Li-O2 Batteries via LiOH Formation and Decomposition Using High-Performance MOF-74@CNTs Hybrid Catalysts. Energy Storage Mater. 17, 167–177. doi:10.1016/j.ensm.2018.11.014
Zhang, X., Guo, L., Gan, L., Zhang, Y., Wang, J., Johnson, L. R., et al. (2017). LiO2: Cryosynthesis and Chemical/Electrochemical Reactivities. J. Phys. Chem. Lett. 8 (10), 2334–2338. doi:10.1021/acs.jpclett.7b00680
Keywords: lithium–oxygen batteries, LiOH chemistry, reversibility, high energy batteries, reaction mechanisms, redox mediator, lithium metal anode
Citation: Zhang X, Dong P and Song M-K (2022) Advances in Lithium–Oxygen Batteries Based on Lithium Hydroxide Formation and Decomposition. Front. Chem. 10:923936. doi: 10.3389/fchem.2022.923936
Received: 19 April 2022; Accepted: 23 May 2022;
Published: 01 July 2022.
Edited by:
Seung Woo Lee, Georgia Institute of Technology, United StatesReviewed by:
Chen Liao, Argonne National Laboratory (DOE), United StatesCopyright © 2022 Zhang, Dong and Song. This is an open-access article distributed under the terms of the Creative Commons Attribution License (CC BY). The use, distribution or reproduction in other forums is permitted, provided the original author(s) and the copyright owner(s) are credited and that the original publication in this journal is cited, in accordance with accepted academic practice. No use, distribution or reproduction is permitted which does not comply with these terms.
*Correspondence: Min-Kyu Song, bWlua3l1LnNvbmdAd3N1LmVkdQ==