- 1Materials Science and Engineering Program, University of Colorado Boulder, Boulder, CO, United States
- 2Paul M. Rady Mechanical Engineering Department, University of Colorado Boulder, Boulder, CO, United States
- 3ATLAS Institute, University of Colorado Boulder, Boulder, CO, United States
Despite hundreds of studies involving slide-ring gels derived from cyclodextrin (CD)-based polyrotaxanes (PRs), their covalent cross-linking kinetics are not well characterized. We employ chemorheology as a tool to measure the gelation kinetics of a model slide-ring organogel derived from α-cyclodextrin/poly (ethylene glycol) PRs cross-linked with hexamethylenediisocyanate (HMDI) in DMSO. The viscoelastic properties of the gels were monitored in situ by small-amplitude oscillatory shear (SAOS) rheology, enabling us to estimate the activation barrier and rate law for cross-linking while mapping experimental parameters to kinetics and mechanical properties. Gelation time, gel point, and final gel elasticity depend on cross-linker concentration, but polyrotaxane concentration only affects gelation time and elasticity (not gel point), while temperature only affects gelation time and gel point (not final elasticity). These measurements facilitate the rational design of slide-ring networks by simple parameter selection (temperature, cross-linker concentration, PR concentration, reaction time).
Introduction
Main-chain polyrotaxanes are mechanically bonded macromolecules with sliding bead-on-string structures which, when appropriately cross-linked, give rise to slide-ring networks Okumura and Ito (2001); Mayumi et al. (2015) having remarkable stress-dissipative properties. Mayumi and Ito (2010) The most highly researched slide-ring networks are derived from the poly (ethylene glycol) (PEG)/α-cyclodextrin (α-CD) polyrotaxanes first introduced by Harada, Harada et al. (1992) due in large part to their favorable cost, biocompatibility, and accessibility. Araki and Ito (2007) These networks can exhibit high softness, Kato et al. (2013) extensibility, Bin Imran et al. (2014); Kato et al. (2015b); Jiang et al. (2018) and fracture toughness, Liu et al. (2017, 2019) leading to varied applications in technologies such as cell-growth substrates Hyodo et al. (2019); Rajendran et al. (2019), dental materials Matsunaga et al. (2020), piezoelectric sensorsSeo et al. (2021), scratch-resistant coatings, Noda et al. (2014); Seo et al. (2019) and battery electrode binders. Choi et al. (2017); Cho et al. (2019); Yoo et al. (2019) Despite the growing reach of slide-ring gels, Hart et al. (2021) reports on their kinetics are limited mainly to gel degradation Kang et al. (2021) or physical gels formed by hydrogen-bond-driven α-CD micro-crystallization. Travelet et al. (2009, 2010a,b).
In the course of our own slide-ring experiments, Dikshit and Bruns (2021) we sought to characterize the kinetics of chemically cross-linked polyrotaxane gels in order to calibrate our reaction times. Here we chemically cross-link α-CD PRs and use SAOS rheological time sweeps to characterize the gelation process in situ by observing the temporal evolution of the viscoelastic moduli. We show how the kinetics and mechanics of a model slide-ring gel depend on temperature, cross-linker concentration, PR concentration, and reaction time. This information will facilitate the selection and fine-tuning of desired network structure and properties.
Results and Discussion
We chose to measure the chemical gelation kinetics by chemorheology Halley and Mackay (1996) to serve our interests in gel mechanics. The PR physical gelation studies Travelet et al. (2009, 2010a,b) have validated a rheological approach to measuring gelation kinetics in PR systems. Among a variety of hydroxyl-reactive cross-linkers, Karino et al. (2006); Samitsu et al. (2006); Shinohara et al. (2006); Ito (2007) HMDI has been reacted with CD-based PRs to give slide-ring gels, Kato et al. (2013) adhesives, Dikshit and Bruns (2021) elastomers, Minato et al. (2017) nanocomposites, Iida et al. (2021) polymer electrodes, Lin et al. (2018) and piezoelectric sensors. Seo et al. (2021) In our model system, we employ HMDI in DMSO with 0.1% v/v dibutyltin dilaurate (DBTDL) to cross-link (Scheme 1) the α-CD rings of an adamantamine-capped Araki et al. (2005) polyrotaxane (PR) based on a PEG backbone of molecular weight (MW) 35,000 Da and an inclusion ratio (proportion of threaded diethylene glycol units in the chain) of ∼30%, corresponding to ∼120 α-CD rings per chain based on NMR signal integration. The number-average molecular weight of 152 kDa obtained using NMR analysis (Supplementary Figure S1) is comparable to that estimated by gel permeation chromatography (138 kDa, Supplementary Figure S2). Specimen labels of the form PPCCTT differentiate the samples based on PR concentration (PP), HMDI concentration (CC) and temperature (TT). By monitoring the isothermal evolution of viscoelastic moduli in SAOS, we describe how these parameters impact gelation kinetics and mechanical properties. Other gelation parameters, such as sample volume (0.5 ml) and reaction time (18 h), were held constant across all experiments.
Figure 1A shows an example of the evolution of storage moduli (G′) and loss moduli (G″) in specimen P10C5T60 ([PR] = 10% w/v [HMDI] = 5% v/v, T = 60°C) over 18 h. The inset magnifies the data at the gel point, where G’ and G” intersect at crossover time tcross. We performed the analogous sweeps while varying PR concentration from 1 to 10% w/v (Supplementary Figure S3, [HMDI] = 5% v/v, T = 60°C), HMDI concentration from 0.5 to 10% v/v (Supplementary Figure S4, [PR] = 10% w/v, T = 25°C), and temperature from 25 to 60°C (Supplementary Figure S5, [PR] = 10% v/v, [HMDI] = 5% w/v). A thin layer of heavy mineral oil is maintained around the sample throughout the in situ gelation to prevent drying. Without the ring of mineral oil, an 18-h time sweep of sample P10C0T25 (no cross-linker) exhibits an increase in viscoelastic moduli and gel point due to physical gelation associated with sample drying; in the presence of mineral oil the same solution does not dry and the moduli remain unchanged over the 18-h interval (Supplementary Figure S6). Isothermal time sweeps have been used to capture time-resolved viscoelasticity data in other cross-linking reactions, Chambon and Winter (1987); Adrus and Ulbricht (2013); Vo et al. (2020) where the evolution of G′ and G″ can be linked to specific processes in the sample. G′ and G″ are equal at the gel point, above which the material attains enough elastically effective cross-links to exhibit solid-like behavior. Vlassopoulos et al. (1998) The Arrhenius plot (Figure 1B) of ln (tcross) vs inverse temperature (1/T) was used to estimate Yang et al. (2015) a gelation activation energy of 96 kJ/mol. Since this activation energy exceeds that of small-molecule isocyanate-hydroxyl coupling, Cheikh et al. (2019) we infer that autoretardation due to cross-linker immobilization on the PR and nearest neighbor effects Alfrey and Lloyd (1963) are involved. This finding also supports the presumed mechanism of gelation driven by urethane formation between HMDI and α-CD, since the physical gelation process driven by hydrogen bonding in α-CD PRs has negative activation energy. Travelet et al. (2009) Further information can be extracted Sun Han Chang et al. (2019) from the time sweeps by fitting the data to the modified Hill Eq (1):
where θ is gelation halftime,
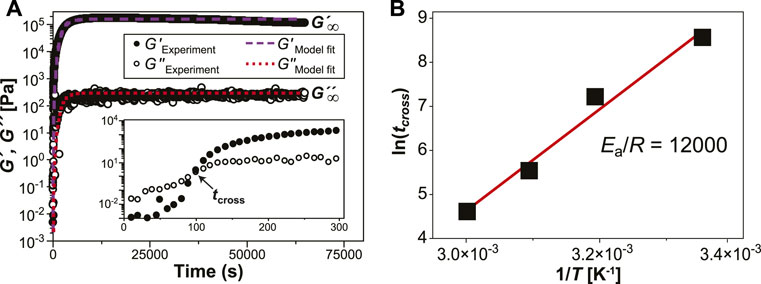
FIGURE 1. (A) Chemorheological time sweep of sample P10C5T60 over 68,400 s (18 h) show the plateau moduli (G′,G″) when gelation is complete. Inset magnifies the gel point at crossover time (tcross) in the first 300 s. (B) Activation energy of gelation. An Arrhenius plot of tcross against 1/T gives an activation energy of 96 kJ/mol for the cross-linking reaction.
The fitted data show that gelation can be accelerated by increasing PR concentration (Figure 2A), HMDI concentration (Figure 2B), or temperature (Figure 2C). The rate

FIGURE 2. Tracking gelation as a function of reaction conditions. Gelation halftime (θ) and rate of production of elastically effective crosslinks (
The plateau modulus,
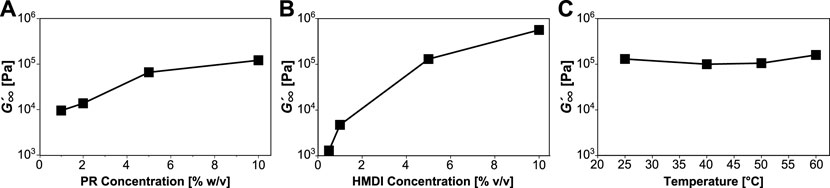
FIGURE 3. Post-gelation mechanics of slide-ring gels. Plateau modulus
We leveraged our time-resolved viscoelasticity data to demonstrate a novel way to tune slide-ring gel softness based on reaction time. We hypothesized that quenching the cross-linking reaction prior to completion would result in softer final gels with moduli that are predictably lower, proportional to the in situ viscoelastic moduli at quench time. Samples of P10C5T25 in glass scintillation vials were quenched at time points of 10, 14, and 18 h by the addition of excess methanol, followed by vacuum drying overnight at 70°C. The quenched gels were swollen in DMSO for 24 h before carrying out frequency sweeps (0.1–100 rad/s, strain 1%, Supplementary Figure S11). The red data points representing these gels, softened due to swelling, Subramani et al. (2020) are superimposed on the in situ sweep of P10C5T25 in Figure 4, showing uniformly lower values. The storage moduli of the swollen quenched gels increase from 5.3 kPa (10 h) to 14.4 kPa (18 h), while the corresponding loss moduli increase from 61 to 491 Pa, respectively. The ratio of G′18h:G′10h is 2.7 in situ and 2.5 after quenching and swelling. Likewise, G′14h:G′10h is 1.8 in situ and 1.9 after quenching and swelling. The nearly matching ratios confirm that the chemorheological sweeps can be used to predict and select desired modulus values (below the maximum) by quenching at an appropriate time in the evolution of the cross-linking reaction. The swelling ratio of the quenched gel decreases from 16 at 10 h to eight at 18 h (Supplementary Table S1), confirming the higher cross-link density of the latter sample. This quenching procedure provides an additional handle to fine-tune gel stiffness beyond HMDI concentration, which is a more course-tuning factor. Since it is performed at room temperature, it also reduces equipment and labor requirements.
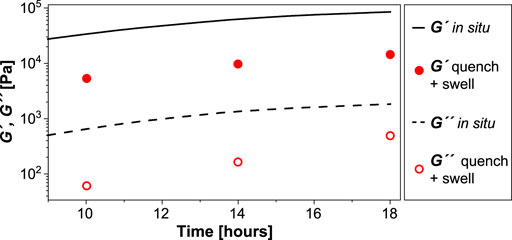
FIGURE 4. Controlling gelation and polyrotaxane mechanics. Storage and loss moduli values (circular symbols) of gels terminated at 10 h, 14 h, and 18 h are compared with the time sweep data of P10C5T25. The swelling ratios (by weight) of the organogel are mentioned at the specific time points in the gelation process.
Conclusion
In summary, chemorheological characterization of polyrotaxane gelation kinetics provides insight on the evolution of gel elasticity and its dependence on polymer and cross-linker concentration, as well as temperature. Our kinetics data confirm that the mechanism of polyrotaxane gelation is driven by the formation of chemical cross-links and may also help researchers calibrate their gelation conditions to achieve desired gel structure and properties. The reaction is, unsurprisingly, most sensitive to cross-linker concentration [HMDI], which influences the gelation time, gel point, and final elasticity. PR concentration impacts the reaction rate more strongly, but the final gel elasticity is less affected, while the gel point is almost unchanged at all PR concentrations. The reaction temperature does not affect the final gel mechanics, but the total reaction time is reduced from 18 h at room temperature to less than 1 h at 60°C, information which is of practical consequence for preparing these gels. We also showed that the gelation may be arrested mid-reaction by quenching, and the elasticity of the resulting swollen gels are proportional to those made in situ. In addition to the experimental parameters probed here, other work has shown that factors such as PR molecular weight, Ohmori et al. (2016) inclusion ratio, Kato et al. (2015b); Jiang et al. (2018) ring size, Kato et al. (2015a) functional groups, Bin Imran et al. (2014); Liu et al. (2021) and type of cross linker Kato et al. (2019); Liu et al. (2021) also play major roles in the mechanical properties of slide-ring gels. Our chemorheological data add to this body of knowledge and enable judicious selection of reactant concentrations, temperature, and reaction time to control the viscoelastic properties of slide-ring gels over a wide range of modulus values between 1 kPa and 1 MPa.
Data Availability Statement
The original contributions presented in the study are included in the article/Supplementary Material. Further inquiries can be directed to the corresponding author.
Author Contributions
KD and CB planned experiments, performed analysis, and wrote the manuscript. KD performed all experiments.
Funding
This work was supported by funding from the College of Engineering and Applied Science at the University of Colorado Boulder and the National Science Foundation (Award No. 2023179).
Conflict of Interest
The authors declare that the research was conducted in the absence of any commercial or financial relationships that could be construed as a potential conflict of interest.
Publisher’s Note
All claims expressed in this article are solely those of the authors and do not necessarily represent those of their affiliated organizations, or those of the publisher, the editors and the reviewers. Any product that may be evaluated in this article, or claim that may be made by its manufacturer, is not guaranteed or endorsed by the publisher.
Supplementary Material
The Supplementary Material for this article can be found online at: https://www.frontiersin.org/articles/10.3389/fchem.2022.923775/full#supplementary-material
References
Adibnia, V., and Hill, R. J. (2016). Universal Aspects of Hydrogel Gelation Kinetics, Percolation and Viscoelasticity from Pa-Hydrogel Rheology. J. Rheol. 60, 541–548. doi:10.1122/1.4948428
Adrus, N., and Ulbricht, M. (2013). Rheological Studies on Pnipaam Hydrogel Synthesis via In Situ Polymerization and on Resulting Viscoelastic Properties. React. Funct. Polym. 73, 141–148. doi:10.1016/j.reactfunctpolym.2012.08.015
Alfrey, T., and Lloyd, W. G. (1963). Kinetics of High‐Polymer Reactions: Effects of Neighboring Groups. J. Chem. Phys. 38, 318–321. doi:10.1063/1.1733659
Araki, J., and Ito, K. (2007). Recent Advances in the Preparation of Cyclodextrin-Based Polyrotaxanes and Their Applications to Soft Materials. Soft Matter 3, 1456–1473. doi:10.1039/b705688e
Araki, J., Zhao, C., and Ito, K. (2005). Efficient Production of Polyrotaxanes from α-Cyclodextrin and Poly(ethylene Glycol). Macromolecules 38, 7524–7527. doi:10.1021/ma050290+
Benedini, S., Zheng, Y., Nitti, A., Mazza, M. M. A., Dondi, D., Raymo, F. x. i. M., et al. (2021). Large Polarization of Push–Pull “Cruciforms” via Coordination with Lanthanide Ions. New J. Chem. 46, 221–227. doi:10.1039/D1NJ04358G
Bin Imran, A., Esaki, K., Gotoh, H., Seki, T., Ito, K., Sakai, Y., et al. (2014). Extremely Stretchable Thermosensitive Hydrogels by Introducing Slide-Ring Polyrotaxane Cross-Linkers and Ionic Groups into the Polymer Network. Nat. Commun. 5, 5124. doi:10.1038/ncomms6124
Chambon, F., and Winter, H. H. (1987). Linear Viscoelasticity at the Gel Point of a Crosslinking Pdms with Imbalanced Stoichiometry. J. Rheol. 31, 683–697. doi:10.1122/1.549955
Cheikh, W., Rózsa, Z. B., Camacho López, C. O., Mizsey, P., Viskolcz, B., Szőri, M., et al. (2019). Urethane Formation with an Excess of Isocyanate or Alcohol: Experimental and ab initio Study. Polymers 11, 1543. doi:10.3390/polym11101543
Cho, Y., Kim, J., Elabd, A., Choi, S., Park, K., Kwon, T.-w., et al. (2019). A Pyrene–Poly (Acrylic Acid)–Polyrotaxane Supramolecular Binder Network for High-Performance Silicon Negative Electrodes. Adv. Mater. 31, 1905048. doi:10.1002/adma.201905048
Choi, S., Kwon, T.-w., Coskun, A., and Choi, J. W. (2017). Highly Elastic Binders Integrating Polyrotaxanes for Silicon Microparticle Anodes in Lithium Ion Batteries. Science 357, 279–283. doi:10.1126/science.aal4373
Dikshit, K., and Bruns, C. J. (2021). Post-Synthesis Modification of Slide-Ring Gels for Thermal and Mechanical Reconfiguration. Soft Matter 17, 5248–5257. doi:10.1039/d0sm02260h
Fleury, G., Schlatter, G., Brochon, C., Travelet, C., Lapp, A., Lindner, P., et al. (2007). Topological Polymer Networks with Sliding Cross-Link Points: the “Sliding Gels”. Relationship between Their Molecular Structure and the Viscoelastic as Well as the Swelling Properties. Macromolecules 40, 535–543. doi:10.1021/ma0605043
Giraldo, J., Vivas, N. M., Vila, E., and Badia, A. (2002). Assessing the (A) Symmetry of Concentration-Effect Curves: Empirical versus Mechanistic Models. Pharmacol. Ther. 95, 21–45. doi:10.1016/s0163-7258(02)00223-1
Gorteau, V., Bollot, G., Mareda, J., Pasini, D., Tran, D.-H., Lazar, A. N., et al. (2005). Synthetic Multifunctional Pores that Open and Close in Response to Chemical Stimulation. Bioorg. Med. Chem. 13, 5171–5180. doi:10.1016/j.bmc.2005.05.022
Halley, P. J., and Mackay, M. E. (1996). Chemorheology of Thermosets—An Overview. Polym. Eng. Sci. 36, 593–609. doi:10.1002/pen.10447
Harada, A., Li, J., and Kamachi, M. (1992). The Molecular Necklace: A Rotaxane Containing Many Threaded α-Cyclodextrins. Nature 356, 325–327. doi:10.1038/356325a0
Hart, L. F., Hertzog, J. E., Rauscher, P. M., Rawe, B. W., Tranquilli, M. M., and Rowan, S. J. (2021). Material Properties and Applications of Mechanically Interlocked Polymers. Nat. Rev. Mater. 6, 508–530. doi:10.1038/s41578-021-00278-z
Hyodo, K., Arisaka, Y., Yamaguchi, S., Yoda, T., and Yui, N. (2019). Stimulation of Microvascular Networks on Sulfonated Polyrotaxane Surfaces with Immobilized Vascular Endothelial Growth Factor. Macromol. Biosci. 19, 1800346. doi:10.1002/mabi.201800346
Iida, M., Goto, T., Mayumi, K., Maeda, R., Hatakeyama, K., Ito, T., et al. (2021). Fabrication of Polyrotaxane and Graphene Nanoplate Composites with High Thermal Conductivities. Polym. Compos. 42, 5556–5563. doi:10.1002/pc.26246
Ito, K. (2007). Novel Cross-Linking Concept of Polymer Network: Synthesis, Structure, and Properties of Slide-Ring Gels with Freely Movable Junctions. Polymer 39, 489–499. doi:10.1295/polymj.pj2006239
Jiang, L., Liu, C., Mayumi, K., Kato, K., Yokoyama, H., and Ito, K. (2018). Highly Stretchable and Instantly Recoverable Slide-Ring Gels Consisting of Enzymatically Synthesized Polyrotaxane with Low Host Coverage. Chem. Mater. 30, 5013–5019. doi:10.1021/acs.chemmater.8b01208
Junior, E. A. P., Davila, J. L., and d’Avila, M. A. (2019). Rheological Studies on Nanocrystalline Cellulose/alginate Suspensions. J. Mol. Liq. 277, 418–423. doi:10.1016/j.molliq.2018.12.091
Kang, T. W., Tamura, A., Arisaka, Y., and Yui, N. (2021). Visible Light-Degradable Supramolecular Gels Comprising Cross-Linked Polyrotaxanes Capped by Trithiocarbonate Groups. Polym. Chem. 12, 3794–3805. doi:10.1039/d1py00569c
Karino, T., Shibayama, M., and Ito, K. (2006). Slide-ring Gel: Topological Gel with Freely Movable Cross-Links. Phys. B Condens. Matter 385, 692–696. doi:10.1016/j.physb.2006.05.293
Kato, K., Yasuda, T., and Ito, K. (2013). Viscoelastic Properties of Slide-Ring Gels Reflecting Sliding Dynamics of Partial Chains and Entropy of Ring Components. Macromolecules 46, 310–316. doi:10.1021/ma3021135
Kato, K., Karube, K., Nakamura, N., and Ito, K. (2015a). The Effect of Ring Size on the Mechanical Relaxation Dynamics of Polyrotaxane Gels. Polym. Chem. 6, 2241–2248. doi:10.1039/c4py01644k
Kato, K., Okabe, Y., Okazumi, Y., and Ito, K. (2015b). A Significant Impact of Host-Guest Stoichiometry on the Extensibility of Polyrotaxane Gels. Chem. Commun. 51, 16180–16183. doi:10.1039/c5cc07122d
Kato, K., Ikeda, Y., and Ito, K. (2019). Direct Determination of Cross-Link Density and its Correlation with the Elastic Modulus of a Gel with Slidable Cross-Links. ACS Macro Lett. 8, 700–704. doi:10.1021/acsmacrolett.9b00238
Kodavaty, J., Venkat, G., Deshpande, A. P., and Gummadi, S. N. (2020). Molecular Association and Gelling Characteristics of Curdlan. Curr. Sci. 118, 1436–1442. doi:10.18520/cs/v118/i9/1436-1442
Lin, Y.-C., Ito, K., and Yokoyama, H. (2018). Solid Polymer Electrolyte Based on Crosslinked Polyrotaxane. Polymer 136, 121–127. doi:10.1016/j.polymer.2017.12.046
Liu, C., Kadono, H., Mayumi, K., Kato, K., Yokoyama, H., and Ito, K. (2017). Unusual Fracture Behavior of Slide-Ring Gels with Movable Cross-Links. ACS Macro Lett. 6, 1409–1413. doi:10.1021/acsmacrolett.7b00729
Liu, C., Kadono, H., Yokoyama, H., Mayumi, K., and Ito, K. (2019). Crack Propagation Resistance of Slide-Ring Gels. Polymer 181, 121782. doi:10.1016/j.polymer.2019.121782
Liu, C., Morimoto, N., Jiang, L., Kawahara, S., Noritomi, T., Yokoyama, H., et al. (2021). Tough Hydrogels with Rapid Self-Reinforcement. Science 372, 1078–1081. doi:10.1126/science.aaz6694
Matsunaga, S., Tamura, A., Fushimi, M., Santa, H., Arisaka, Y., Nikaido, T., et al. (2020). Light-embrittled Dental Resin Cements Containing Photodegradable Polyrotaxane Cross-Linkers for Attenuating Debonding Strength. ACS Appl. Polym. Mater. 2, 5756–5766. doi:10.1021/acsapm.0c01024
Mayumi, K., and Ito, K. (2010). Structure and Dynamics of Polyrotaxane and Slide-Ring Materials. Polymer 51, 959–967. doi:10.1016/j.polymer.2009.12.019
Mayumi, K., Ito, K., and Kato, K. (2015). Polyrotaxane and Slide-Ring Materials. Cambridge: Royal Society of Chemistry.
Minato, K., Mayumi, K., Maeda, R., Kato, K., Yokoyama, H., and Ito, K. (2017). Mechanical Properties of Supramolecular Elastomers Prepared from Polymer-Grafted Polyrotaxane. Polymer 128, 386–391. doi:10.1016/j.polymer.2017.02.090
Noda, Y., Hayashi, Y., and Ito, K. (2014). From Topological Gels to Slide-Ring Materials. J. Appl. Polym. Sci. 131, 40509. doi:10.1002/app.40509
Ohmori, K., Bin Imran, A., Seki, T., Liu, C., Mayumi, K., Ito, K., et al. (2016). Molecular Weight Dependency of Polyrotaxane-Cross-Linked Polymer Gel Extensibility. Chem. Commun. 52, 13757–13759. doi:10.1039/c6cc07641f
Okumura, Y., and Ito, K. (2001). The Polyrotaxane Gel: A Topological Gel by Figure-Of-Eight Cross-Links. Adv. Mater. 13, 485–487. doi:10.1002/1521-4095(200104)13:7<485:aid-adma485>3.0.co;2-t
Rajendran, A. K., Arisaka, Y., Iseki, S., and Yui, N. (2019). Sulfonated Polyrotaxane Surfaces with Basic Fibroblast Growth Factor Alter the Osteogenic Potential of Human Mesenchymal Stem Cells in Short-Term Culture. ACS Biomater. Sci. Eng. 5, 5652–5659. doi:10.1021/acsbiomaterials.8b01343
Samitsu, S., Araki, J., Kataoka, T., and Ito, K. (2006). New Solvent for Polyrotaxane. II. Dissolution Behavior of Polyrotaxane in Ionic Liquids and Preparation of Ionic Liquid-Containing Slide-Ring Gels. J. Polym. Sci. B Polym. Phys. 44, 1985–1994. doi:10.1002/polb.20849
Seo, J., Moon, S. W., Kang, H., Choi, B.-H., and Seo, J. H. (2019). Foldable and Extremely Scratch-Resistant Hard Coating Materials from Molecular Necklace-like Cross-Linkers. ACS Appl. Mater. Interf. 11, 1–12. doi:10.1021/acsami.9b05738
Seo, J., Hur, J., Kim, M.-S., Lee, T.-G., Seo, S. J., Han, S. H., et al. (2021). All-organic Piezoelectric Elastomer Formed through the Optimal Cross-Linking of Semi-crystalline Polyrotaxanes. Chem. Eng. J. 426, 130792. doi:10.1016/j.cej.2021.130792
Shinohara, Y., Kayashima, K., Okumura, Y., Zhao, C., Ito, K., and Amemiya, Y. (2006). Small-angle X-Ray Scattering Study of the Pulley Effect of Slide-Ring Gels. Macromolecules 39, 7386–7391. doi:10.1021/ma061037s
Subramani, R., Izquierdo-Alvarez, A., Bhattacharya, P., Meerts, M., Moldenaers, P., Ramon, H., et al. (2020). The Influence of Swelling on Elastic Properties of Polyacrylamide Hydrogels. Front. Mater. 7, 212. doi:10.3389/fmats.2020.00212
Sun Han Chang, R., Lee, J. C.-W., Pedron, S., Harley, B. A., and Rogers, S. A. (2019). Rheological Analysis of the Gelation Kinetics of an Enzyme Cross-Linked Peg Hydrogel. Biomacromolecules 20, 2198–2206. doi:10.1021/acs.biomac.9b00116
Travelet, C., Schlatter, G., Hebraud, P., Brochon, C., Lapp, A., and Hadziioannou, G. (2009). Formation and Self-Organization Kinetics of α-cd/peo-Based Pseudo-Polyrotaxanes in Water. a Specific Behavior at 30 c. Langmuir 25, 8723–8734. doi:10.1021/la900070v
Travelet, C., Hebraud, P., Perry, C., Brochon, C., Hadziioannou, G., Lapp, A., et al. (2010a). Temperature-Dependent Structure of α-cd/peo-based Polyrotaxanes in Concentrated Solution in Dmso: Kinetics and Multiblock Copolymer Behavior. Macromolecules 43, 1915–1921. doi:10.1021/ma902686p
Travelet, C., Schlatter, G., Hébraud, P., Brochon, C., Anokhin, D. V., Ivanov, D. A., et al. (2010b). Physical Gels Based on Polyrotaxanes: Kinetics of the Gelation, and Relative Contributions of α-cyclodextrin and Poly (Ethylene Oxide) to the Gel Cohesion. Macromol. Symp. 291, 202–211. doi:10.1002/masy.201050524
Vlassopoulos, D., Chira, I., Loppinet, B., and McGrail, P. T. (1998). Gelation Kinetics in Elastomer/Thermoset Polymer Blends. Rheol. acta 37, 614–623. doi:10.1007/s003970050148
Vo, N. T., Huang, L., Lemos, H., Mellor, A., and Novakovic, K. (2020). Poly (Ethylene Glycol)-Interpenetrated Genipin-Crosslinked Chitosan Hydrogels: Structure, Ph Responsiveness, Gelation Kinetics, and Rheology. J. Appl. Polym. Sci. 137, 49259. doi:10.1002/app.49259
Yang, M., Wang, D., Sun, N., Chen, C., and Zhao, X. (2015). Rheological Behaviour and Cure Kinetic Studies of a Trifunctional Phenylethynyl-Terminated Imide Oligomer. High. Perform. Polym. 27, 449–457. doi:10.1177/0954008314555521
Keywords: chemorheology, rheology, cyclodextrins, organogels, slide-ring gels, polyrotaxane, viscoelasticity
Citation: Dikshit K and Bruns CJ (2022) Chemorheological Monitoring of Cross-Linking in Slide-ring Gels Derived From α-cyclodextrin Polyrotaxanes. Front. Chem. 10:923775. doi: 10.3389/fchem.2022.923775
Received: 19 April 2022; Accepted: 20 June 2022;
Published: 14 July 2022.
Edited by:
Leyong Wang, Nanjing University, ChinaReviewed by:
Dario Pasini, University of Pavia, ItalyJesús del Barrio, University of Zaragoza, Spain
Copyright © 2022 Dikshit and Bruns . This is an open-access article distributed under the terms of the Creative Commons Attribution License (CC BY). The use, distribution or reproduction in other forums is permitted, provided the original author(s) and the copyright owner(s) are credited and that the original publication in this journal is cited, in accordance with accepted academic practice. No use, distribution or reproduction is permitted which does not comply with these terms.
*Correspondence: Carson J. Bruns , Y2Fyc29uLmJydW5zQGNvbG9yYWRvLmVkdQ==