- 1School of Biotechnology and Health Sciences, Wuyi University, Jiangmen, China
- 2State Key Laboratory of Quality Research in Chinese Medicine, Macau University of Science and Technology, Macau, China
Melatonin (MT) is a hormone with antioxidant activity secreted by the pineal gland in the human brain, which is highly efficient in scavenging free radicals and plays an important role in the neuro-immuno-endocrine system. Emerging evidence showed that MT supplementation was a potential therapeutic strategy for Parkinson’s disease (PD), which inhibits pathways associated with oxidative stress in PD. In this study, we reported a C7-selective olefination of melatonin under rhodium catalysis with the aid of PIII-directing groups and synthesized 10 new melatonin-C7-cinnamic acid derivatives (6a–6j). The antioxidant potential of the compounds was evaluated both by ABTS and ORAC methods. Among these newly synthesized melatonin derivatives, 6a showed significantly higher activity than MT at 10−5 M. In the transgenic Caenorhabditis elegans model of PD, 6a significantly reduces alpha-synuclein aggregation and dopaminergic neuronal damage in nematodes while reducing intracellular ROS levels and recovers behavioral dysfunction induced by dopaminergic neurodegeneration. Further study of the mechanism of action of this compound can provide new therapeutic ideas and treatment strategies for PD.
Introduction
Melatonin (MT), secreted by the midbrain pineal gland and some peripheral tissues, is a tryptophan metabolite that activates multiple intracellular signaling pathways (Pohanka et al., 2011) and has a variety of physiological effects, such as regulating circadian rhythms, scavenging free radicals, enhancing immunity, and inhibiting oxidation of biomolecules (Yu et al., 2000; Skene and Swaab., 2003; Wu et al., 2004). In addition, melatonin has protective effects against neurodegenerative diseases (Rudnitskaya et al., 2015).
Parkinson’s disease (PD) is a neurodegenerative disease with motor and non-motor symptoms characterized by the loss of dopaminergic neurons in the nigrostriatal and the formation of Lewy body proteins (Calabrese et al., 2018; Bouca-Machado et al., 2019). The main features of PD are resting tremor, rigidity, and motor retardation (Mann and Yates, 1982; Smeyne and Jackson-Lewis, 2005; Jankovic and Stacy., 2007; Hirsch et al., 2012). This may be due to the selective loss of dopaminergic (DA) neurons in the substantia nigra densa (SN), resulting in neurological dysfunction (Delenclos et al., 2016). Pathologically, PD is also characterized by the formation of alpha-synuclein (α-syn) aggregates. The pathological process of PD involves multiple pathways, including apoptosis, autophagy, oxidative stress, α-syn aggregation, and alterations in neurotransmitters (Olzmann et al., 2010; Martinez et al., 2017; Aditi and Verma, 2019; Brunetti et al., 2020).
There is emerging evidence supporting the impact of oxidative stress on PD, and therefore, drugs with antioxidant activity are expected to be a potential treatment for PD (Moosmann and Behl, 2002; Abou-Sleiman et al., 2006; Weber and Ernst, 2006; Sayre et al., 2008). Studies suggest that MT supplementation is a therapeutic approach for PD disorder. The use of MT inhibits a number of pathways associated with oxidative stress response, α-syn aggregation, and dopamine loss in PD (Chen et al., 2002; Zarranz et al., 2004; Berendse et al., 2010). MT may also improve some non-motor symptoms in PD patients.
The nematode Caenorhabditis elegans (C. elegans) is a powerful genetic model system for exploring PD and related molecular mechanisms (Nass and Chen, 2008; Anand et al., 2020). In this study, we used two well-established C. elegans models to assess the anti-Parkinsonian effects of melatonin derivatives and to explore their associated potential neuroprotective mechanisms.
Materials and Methods
Materials and Strains
1H NMR and 13C NMR spectra were both performed on a 500 MHz Bruker NMR spectrometer using TMS as an internal standard (chloroform-d as the solvent). Mass spectra were carried out using a Thermo Fisher LCQ Fleet LC-MS mass spectrometer. All reagents used were of analytical grade.
Strains: Bristol N2: wild-type N2 nematode; NL5901: pkIs2386 [unc-54p:α-synuclein:YFP + unc-119 (+)], a transgenic nematode model of PD disorder expressing yellow fluorescent protein-tagged human α-synuclein in muscle; BZ555: egIs1 [dat-1p:GFP], pharmacological model of PD disorder nematode, dat-1 encodes a plasma membrane dopamine transporter protein labelled with a green fluorescent mono-white that can be observed as bright green fluorescence at the junctions between dopamine neuronal soma cells, purchased from the CGC (Caenorhabditis Genetics Center); E. coli OP50: Escherichia coli OP50, uracil leakage mutant strain, used as normal food for feeding C. elegans.
Synthesis of 6a–6j
Our group designed a PIII-directing group (N-PtBu2), which directed C7-H functionalization of melatonin with olefins through rhodium catalysis. (Borah and Shi, 2018). We describe the development of the decarbonylative cross-couplings (Zhao and Yu, 2008; Lei et al., 2015) of carboxylic acids with melatonin at the C7 position by PIII-chelation-assisted RhI-catalyzed C-C bond activation (Qiu et al., 2019).
The decarbonylative reaction was further optimized with substituted cinnamic acid 4 as a partner to produce the melatonin C7-olefination products. We found that the reaction achieved optimal efficiency and selectivity with 2.5 equiv. of Boc2O in the presence of 5.0 mol% Rh(cod)2OTf as a catalyst. Product 5 was obtained after reaction at 120°C for 18 h under a nitrogen atmosphere. Furthermore, the directing group (PtBu2) could be easily removed by TBAF in THF, and N-free melatonin derivatives 6 were isolated at the yields of 47–67% (Han et al., 2019; Qiu et al., 2019) Scheme 1.
N-(2-(5-Methoxy-7-Styryl-1h-Indol-3-yl)ethyl)acetamide (6a)
Light yellow oil. 67% yield. 1H NMR (500 MHz, Chloroform-d) δ: 8.47 (s, 1H), 7.60∼7.49 (m, 2H), 7.44∼7.35 (m, 2H), 7.35∼7.27 (m, 2H), 7.18 (s, 1H), 7.09 (t, J = 2.0 Hz, 2H), 7.03 (d, J = 2.3 Hz, 1H), 5.62 (s, 1H), 3.92 (s, 3H), 3.62 (q, J = 6.5 Hz, 2H), 2.97 (t, J = 6.8 Hz, 2H), and 1.94 (s, 3H); 13C NMR (125 MHz, chloroform-d) δ: 170.2, 154.4, 137.2, 130.5, 129.8, 128.8, 128.5, 127.9, 126.5, 124.3, 122.9, 122.3, 113.2, 109.9, 100.7, 56.1, 39.8, 25.3, and 23.4; LC-MS (ESI) m/z: calcd. for C21H22N2O2 {[M + Na]+} 357.1573, found 357.1572.
The details of compounds 6b–6j can be found in the Supplementary Material S1.
ABTS Method
The ABTS assay was performed in accordance with a previously reported procedure (Schaich et al., 2015; Sommer et al., 2022). A volume of 5 ml of 7 mmol/L ABTS and 88 μL of 140 mmol/L potassium persulfate were mixed and left for 12 h at room temperature in the dark to form an ABTS+ free radical reserve solution. The reserve liquid was relatively stable at room temperature away from the light. Before use, it was diluted into the working liquid with ultra-pure water, and its absorbance was required to be 0.7 ± 0.02 at 30°C and a wavelength of 734 nm. The samples were prepared with DMSO into 5, 10, 20, and 40 μg/ml sample solutions for later use. During the determination, 100 μL of ABTS working solution was added to each well of the 96-well microtitration plate, and then 100 μL of sample solution at different concentrations was added to shake and mix. After 10 min, the absorbance at 734 nm was determined, and 100 μL of ABTS working solution mixed with 100 μL of DMSO was used to determine the blank absorbance A0. The absorbance of a 100 μL sample mixed with 100 μL of DMSO was defined as Ar. For each determination, experiments were performed in triplicate.
The radical scavenging rate of ABTS is calculated using the following formula: ABTS free radical scavenging rate (%) = [1 − (At − Ar)/A0] × 100%.
ORAC Method
The ORAC method was performed as described previously (Huang et al., 2002; Ou et al., 2002) with slight modification. The reaction was carried out in 75 mM phosphate buffer (pH 7.4), while the addition of antioxidant substances produced more stable fluorescent signal that could reflect the antioxidant capacity.
A volume of 50 µL of samples to be tested or different concentrations of Trolox working solution (0.10, 0.08, 0.06, 0.04, and 0.02 mmol/L) were added to a 96-well plate, followed by the addition of 100 µL of FL working solution (8.4 × 10–8 mol/L) and shaking for 30 s. The fluorescence value F0 was recorded immediately, and then the reaction was shaken for 3 min and incubated at 37 °C for 10 min. A volume of 50 µL of AAPH working fluid was added to induce the reaction. The excitation wavelength and emission wavelength were 485 and 535 nm, respectively, and the fluorescence value Fn was recorded every 2.5 min. The reaction was considered to be over when the fluorescence decrease slowed. The initial fluorescence value (F0) is the pore fluorescence intensity reading without AAPH. fn = fluorescence value (Fn)/initial fluorescence value (F0); AUC = 2 × (f0 + f1 +...+ fn) − f0 − fn; NetAUC (ORAC value) = AUCsample − AUCAAPH+. NetAUC was used to determine the capacity for absorbing free radicals. Here, AUC is the area under the fluorescence decay curve, and NetAUC is the protected area. In addition to the tested sample group, a blank group and an AAPH + group (for which 50 µL of 75 mM phosphate-buffered saline (PBS) solution was used as the sample substitution) were also tested. To create a standard curve, different concentrations of Trolox solution were used for the abscissa and NetAUC was used for the ordinate, and the absorption capacity of the sample was expressed as μmol TE/g DW. For each determination, experiments were performed in triplicate.
Measurement of Reactive Oxygen Species (ROS)
2′,7′-Dichlorofluorescein diacetate (H2DCFDA), a universal oxidative stress indicator, was used as a probe for the detection of intracellular ROS. H2DCFDA was oxidized by ROS to form the fluorescent dye dichlorofluorescein (DCF) (Jia et al., 2021). The fluorescence intensity was proportional to the ROS level in C. elegans.
Briefly, age-matched L3 larvae were treated with 10 μM MT or 6a for 24 h. Subsequently, 2000 nematodes per group were collected and washed three times with M9 buffer. The nematodes were suspended in 400 µL of PBS with 1% Tween-20 and homogenized to obtain worm lysate. The protein concentration of the lysate was determined using the BCA protein assay kit. A volume of 50 μL of nematode lysate was transferred into a 96-well black microplate and incubated with 50 μL of 50 μM H2DCFDA. Fluorescence intensity (485 nm excitation and 590 nm emission) was monitored using an enzyme plate reader (Wu et at., 2006; Liu et al., 2015; Zhao et al., 2018).
α-Synuclein Accumulation Assay
PD pathology usually showed an accumulation of the α-syn. We used strain NL5901 to test the effect of MT and 6a on α-syn aggregation. The synchronized nematodes were immersed in M9 buffer containing 10 µM melatonin and 6a for 48 h at 20°C. L1 worms were transferred to OP50/NGM plates at 20°C for 65 h (L3 stage), and then the nematodes were transferred to plates containing OP50/NGM/5-fluorodeoxyuridine (FUDR, 0.04 mg/ml) and incubated at 20°C for 3 days. The young adults were washed three times with M9 buffer, then anesthetized with 10% sodium azide, and fixed on slides. The worms were observed using an Olympus BX63 fluorescent microscope to monitor the YFP expression (α-syn aggregation). The fluorescence intensity of each nematode was quantified using ImageJ software (Jadiya et al., 2011; Govindan et al., 2018; Anjaneyulu et al., 2020).
6-OHDA-Induced Damage to Dopaminergic Neurons
Dopaminergic neuron degeneration was induced by 50 mM 6-OHDA in C. elegans as described previously (Tucci et al., 2011). Briefly, the synchronized nematodes were immersed in M9 buffer containing MT or 6a for 48 h at 20°C, and then L1 worms were transferred to OP50/NGM plates at 20°C for 65 h (L3 stage), after which they were exposed to 50 mM 6-OHDA for 1 h. After exposure, the worms were washed with M9 buffer and transferred to OP50/NGM plates containing 0.04 mg/ml FUDR for 3 days at 20 °C for various assays (Chalorak et al., 2021; Ma et al., 2021).
Behavior Assay
In general, N2 nematodes were treated with 50 mM 6-OHDA to induce degenerative lesions in dopamine neurons.
For thrashing assay, synchronized L4 stage nematodes were transferred to a 3-cm diameter Petri dish, 1 ml of M9 buffer was added, and after the nematodes were stabilized for 30 s, a Leica M205 FA microscope was used to continuously capture the worms for 10 s. The number of thrashing of the nematodes was counted (Lee et al., 2021).
For travel distance assay, nematodes of the synchronized L4 stage were transferred from food-containing dishes to non-food dishes and washed three times repeatedly in M9 buffer to remove the remaining food. The distance (mm) and speed (µm/sec) of the nematode’s movement in 20 s were calculated using the Leica M205 FA microscope and finally analyzed using GraphPad Prism software (Sawin et al., 2000).
Statistical Analysis
All the experiments were performed in triplicate. The significance of differences between control and treated groups was analyzed by one-way analysis of variance (ANOVA), followed by Bonferroni’s method. p-values < 0.05 were accepted as statistically significant. Graphs were constructed using GraphPad Prism version 8.00.
Results and Discussion
Antioxidant Activity of Melatonin Derivatives In Vitro
In the ABTS method, the antioxidant activity of the 10 derivatives of melatonin at the C7 position, 6a–6j, increased in a concentration-dependent manner, and the antioxidant activity of 6a–6j was higher than that of melatonin at the same concentration, while the ABTS radical scavenging rate of the 10 derivatives, 6a–6j, was higher than that of vitamin C (VC) and MT at low concentrations of 5 and 10 μg/ml (Figure 1). These results indicated that the derivatization of melatonin at the C7 position could improve its antioxidant activity.
In the ORAC method, at the same concentration (4 μg/ml), the antioxidant activity of derivatives 6b–6j at the C7 position of melatonin was lower than that of melatonin, whereas the antioxidant activity of 6a was higher than that of MT (Figure 2). This suggested that the derivatization of melatonin at the C7 position may increase its antioxidant activity.
In this study, the antioxidant activity of melatonin derivatives was assessed by two in vitro assays, the ABTS method and the ORAC method. Comparing the results of these two methods, we found that the antioxidant activity of 6a, a derivative of melatonin at position C7, was higher than that of MT and the positive control VC as well.
6a Decreased the ROS Level in C. elegans
To further confirm the antioxidant effect of 6a, we tested the intracellular ROS level in C. elegans. We first examined the effect of 6a on the ROS levels in wild-type N2 nematodes. As shown in Figure 3, compared with the untreated group, after treatment with 6a at different concentrations (2, 10, and 50 µM), the levels of ROS in the nematodes decreased, and 10 µM of 6a achieved a significant decrease compared with the level in the untreated group (p < 0.001). Additionally, the ROS levels clearly declined in the 6a group compared with those in the MT group (p < 0.05). These results are consistent with the in vitro antioxidant assay, further demonstrating the antioxidant capacity of 6a. The excellent antioxidant activity of 6a suggests its potential as a therapeutic agent for PD disorders.
To test this hypothesis, we first examined the effect of 6a on the ROS level in the transgenic nematode model of PD. As shown in Figure 4, compared with the untreated group, after treatment with different concentrations (2, 10, and 50 µM) of 6a, the levels of ROS in NL5901 worms reduced, and 10 µM of 6a achieved a significant decrease compared with the level in the untreated group (p < 0.001), along with a clear decline compared with that in the MT group (p < 0.05). These results are consistent with the findings in the ROS levels in N2 worms. The regulation of ROS levels in wild-type and NL590 animals by 6a confirmed its ability to regulate ROS in vivo, suggesting that it could reduce ROS-induced acute oxidative damage.
6a Reduced α-Synuclein Aggregation in the Transgenic Nematode NL5901
One of the typical features of PD patients is the formation of Lewy bodies in the brain. The accumulation of Lewy bodies exacerbates the neurodegenerative progression in PD. Aggregated α-syn, which is known as a major component of Lewy bodies, can be measured in the transgenic NL5901 strain of C. elegans. In this strain, human α-syn is expressed in muscle cells with a YFP reporter. The fluorescence intensity at the anterior end of the worms indicates the accumulation of α-syn. To further confirm the therapeutic effect of 6a on PD, we examined its effect on α-syn aggregation. The results showed that, in the group treated with 10 µM 6a, the YFP fluorescence intensity in NL5901 was reduced compared with that in the untreated control group (Figure 5A). Quantitative analysis of the fluorescence intensity using ImageJ software showed that, in worms treated with 10 µM 6a (73.92 ± 1.80), the fluorescence intensity was lowered by 25.65% (p < 0.001) compared with that in untreated worms (99.45 ± 2.03). In addition, the 6a-treated group had lower fluorescence intensity than the MT-treated group (81.04 ± 2.74, p < 0.05; Figure 5B). These results indicate that the protective effect of 6a occurs by reducing the aggregation of α-syn in the PD model.
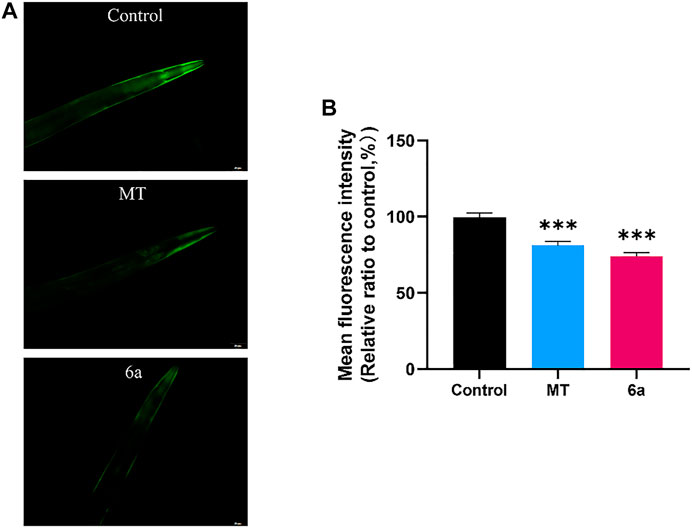
FIGURE 5. α-syn aggregation in NL5901 was decreased by treatment of MT and 6a. (A) Representative images of α-syn accumulation in different groups of head muscles. (B) Quantification of fluorescence intensity in NL5901 using ImageJ software (***p < 0.001).
6a Is Protective Against 6-OHDA-Induced Dopaminergic Neuronal Damage
C. elegans contains exactly eight dopaminergic neurons, including two ADEs, four CEPs, and two PDEs (Figure 6A). In the BZ555 strain, all the eight DA neurons were tagged with GFP. Upon exposure to 50 mM 6-OHDA, the BZ555 strain showed selective damage in DA neurons. To examine the efficacy of 6a, the viability of the DA neurons was assessed by measuring the loss of expression of the GFP reporter. We found that CEP, ADE, and PDE neurons showed partial GFP loss after 6-OHDA treatment (Figure 6B). When nematodes were pretreated with MT or 6a for 48 h at L1, the GFP expression loss was remarkably restored in CEP and ADE neurons (Figure 6B). We further measured the fluorescence intensity in DA neurons using ImageJ software. In nematodes treated with 50 mM 6-OHDA (47.41 ± 2.53), the mean fluorescence (GFP) intensity decreased by about 56% (p < 0.001) compared with that of untreated nematodes (84.71 ± 1.25), whereas dopaminergic neurons of nematodes exposed to 6-OHDA after treatment with 10 µM 6a recovered to 74.28 ± 1.87 (p < 0.001), and the mean fluorescence intensity was higher in the 6a-treated group than in the MT-treated group (72.94 ± 1.09, p < 0.05; Figure 6C), suggesting that 6a is protective against 6-OHDA-induced dopaminergic neuronal damage and that this protective effect is stronger than that of MT.
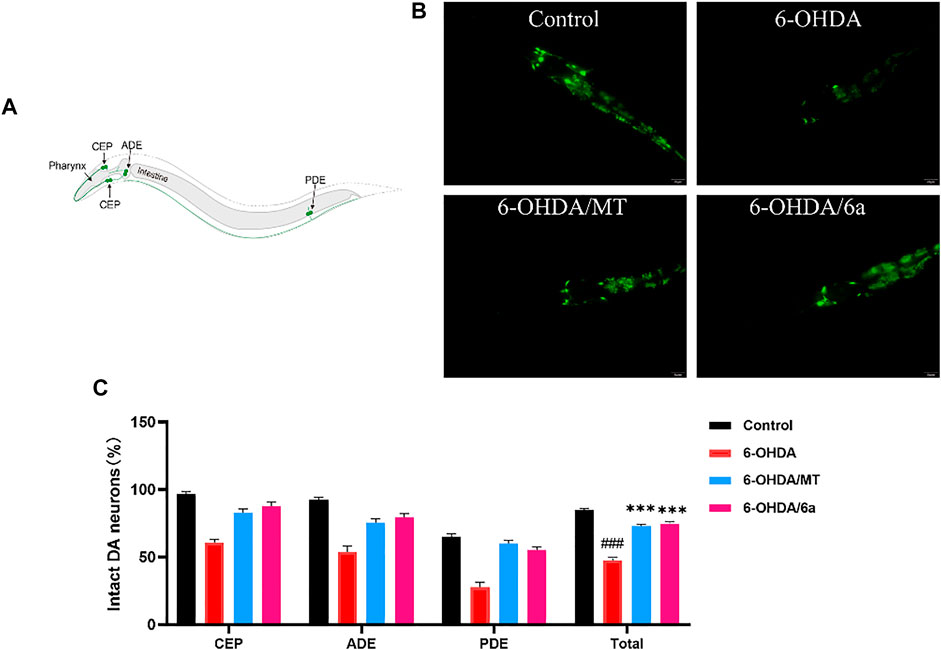
FIGURE 6. Effect of MT and 6a on restoration of 6-OHDA-induced DA neurodegeneration in the BZ555 strain. (A) Neuronal circuits in C. elegans. (B) GFP expression patterns of CEP and ADE in BZ555. (C) Quantification of the fluorescence intensity of all eight DA neurons of BZ555. (###p < 0.001, compared with the control; ***p < 0.001, compared with the 6-OHDA-treated group).
6a Recovers Behavioral Dysfunction Induced by DA Neurodegeneration
We next investigated whether 6a improves the functions of DA neurons. The locomotion of worms slows down when they encounter food, referred to as the basal slowing response, which is mediated by mechanosensory DA neurons. In this study, we found that N2 nematodes showed a 72.2% decrease in speed when on NGM plates with food and a 9.7% decrease in basal deceleration induced by 50 mM 6-OHDA (p < 0.001), in terms of food perception behavior. Meanwhile, there was significant recovery from this decline to 69.9% after treatment with 10 µM 6a (p < 0.001), and the 6a-treated group showed a better basal deceleration response than the melatonin-treated group (p < 0.05) (Figure 7C). Similarly, in wild-type N2 nematodes, supplementation with 6a also restored 6-OHDA-induced deficits in DA neuron-associated motor activity, such as increasing the distance traveled and thrashing behavior (Figures 7A, B). These results suggest that 6a recovers the behavioral dysfunction induced by DA neurodegeneration.
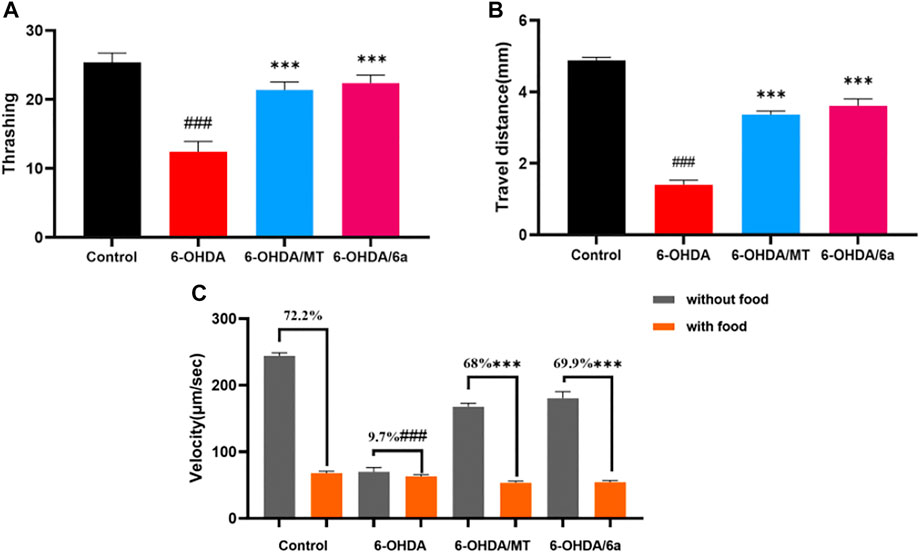
FIGURE 7. Effect of MT and 6a on DA neuronal function. (A) Thrashing behavior of N2. (B) Travel distances of N2. (C) Velocity of N2 in NGM plates with and without food and basal slowing response. (###p < 0.001, compared with the control; ***p < 0.001, compared with the 6-OHDA-treated group).
Conclusion
We successfully synthesized 10 melatonin derivatives 6a–6j, and their activities were tested using in vitro antioxidant assays, ABTS and ORAC. The results showed that the antioxidant activity of melatonin derivative 6a was higher than that of MT. Subsequently, we evaluated the effect of 6a on ROS levels in C. elegans. The results showed that 10 µM 6a significantly reduced the ROS levels in wild-type N2. We also used two PD models of C. elegans to investigate the therapeutic effect of 6a on PD. We found that 10 µM 6a significantly reduced the ROS levels and α-syn aggregation in NL5901. Treatment of BZ555 with 50 mM 6-OHDA reduced the fluorescence intensity of their dopaminergic neuronal cells, which was increased by supplementation with 10 µM 6a. Finally, we evaluated the capacity of 6a to improve behavioral deficits caused by DA neurodegeneration. The results showed that 10 µM 6a improved the nematode’s behavior in perceiving food and increased its basal rate compared with the findings in the control.
Our conclusions prove that melatonin derivative 6a significantly reduces α-syn aggregation and dopaminergic neuronal damage in PD by reducing oxidative stress-induced ROS levels and improves the behavioral impairment caused by DA neurodegeneration. Further study of the mechanism of action of this compound could provide new therapeutic ideas and treatment strategies for PD.
Data Availability Statement
The original contributions presented in the study are included in the article/Supplementary Material; further inquiries can be directed to the corresponding authors.
Author Contributions
NF, A-JM, and W-ZM designed the work and wrote the manuscript. LH, J-JD, J-JZ, M-TC, and LL carried out the experiments. All authors reviewed the manuscript and agreed to its publication.
Funding
This work was supported by the COVID-19 Epidemic Prevention and Control Project of Wuyi University (2020FKZX01), the Department of Education of Guangdong Province (2019KTSCX184, 2020KCXTD036, and 2021KQNCX101), the Hong Kong–Macao Joint Research and Development Fund of Wuyi University (2019WGALH12), the Science Foundation for Young Teachers (2019td02) of Wuyi University, the Jiangmen City Science and Technology Basic Research Project (2021030102630004945), and the Innovations in Graduate Education Program (YJS-SFJD-21-01).
Conflict of Interest
The authors declare that the research was conducted in the absence of any commercial or financial relationships that could be construed as a potential conflict of interest.
Publisher’s Note
All claims expressed in this article are solely those of the authors and do not necessarily represent those of their affiliated organizations, or those of the publisher, the editors and the reviewers. Any product that may be evaluated in this article, or claim that may be made by its manufacturer, is not guaranteed or endorsed by the publisher.
Acknowledgments
We thank Liwen Bianji (Edanz) (www.liwenbianji.cn) for editing the language of the draft of this article.
Supplementary Material
The Supplementary Material for this article can be found online at: https://www.frontiersin.org/articles/10.3389/fchem.2022.918116/full#supplementary-material
References
Abou-Sleiman, P. M., Muqit, M. M. K., and Wood, N. W. (2006). Expanding Insights of Mitochondrial Dysfunction in Parkinson's Disease. Nat. Rev. Neurosci. 7, 207–219. doi:10.1038/nrn1868
Anand, N., Holcom, A., Broussalian, M., Schmidt, M., Chinta, S. J., Lithgow, G. J., et al. (2020). Dysregulated Iron Metabolism in C. elegans catp-6/ATP13A2 Mutant Impairs Mitochondrial Function. Neurobiol. Dis. 139, 104786. doi:10.1016/j.nbd.2020.104786
Anjaneyulu, J., R, V., and Godbole, A. (2020). Differential Effect of Ayurvedic Nootropics on C. elegans Models of Parkinson's Disease. J. Ayurveda Integr. Med. 11, 440–447. doi:10.1016/j.jaim.2020.07.006
Berendse, H. W., Booij, J., Francot, C. M. J. E., Bergmans, P. L. M., Hijman, R., Stoof, J. C., et al. (2001). Subclinical Dopaminergic Dysfunction in Asymptomatic Parkinson's Disease Patients' Relatives with a Decreased Sense of Smell. Ann. Neurol. 50, 34–41. doi:10.1002/ana.1049
Borah, A. J., and Shi, Z. (2018). Rhodium-Catalyzed, Remote Terminal Hydroarylation of Activated Olefins through a Long-Range Deconjugative Isomerization. J. Am. Chem. Soc. 140, 6062–6066. doi:10.1021/jacs.8b03560
Bouça‐Machado, R., Duarte, G. S., Patriarca, M., Castro Caldas, A., Alarcão, J., Fernandes, R. M., et al. (2019). Measurement Instruments to Assess Functional Mobility in Parkinson's Disease: A Systematic Review. Mov. Disord. Clin. Pract. 7, 129–139. doi:10.1002/mdc3.12874
Brunetti, G., Di Rosa, G., Scuto, M., Leri, M., Stefani, M., Schmitz-Linneweber, C., et al. (2020). Healthspan Maintenance and Prevention of Parkinson's-like Phenotypes with Hydroxytyrosol and Oleuropein Aglycone in C. elegans. Ijms 21, 2588. doi:10.3390/ijms21072588
Calabrese, V., Santoro, A., Monti, D., Crupi, R., Di Paola, R., Latteri, S., et al. (2018). Aging and Parkinson's Disease: Inflammaging, Neuroinflammation and Biological Remodeling as Key Factors in Pathogenesis. Free Radic. Biol. Med. 115, 80–91. doi:10.1016/j.freeradbiomed.2017.10.379
Chalorak, P., Sanguanphun, T., Limboonreung, T., and Meemon, K. (2021). Neurorescue Effects of Frondoside A and Ginsenoside Rg3 in C. elegans Model of Parkinson's Disease. Molecules 26, 4843. doi:10.3390/molecules26164843
Chen, S. T., Chuang, J. I., Hong, M. H., and Li, E. I.-C. (2002). Melatonin Attenuates MPP+ -induced Neurodegeneration and Glutathione Impairment in the Nigrostriatal Dopaminergic Pathway. J. Pineal Res. 32, 262–269. doi:10.1034/j.1600-079X.2002.01871.x
Delenclos, M., Jones, D. R., Mclean, P. J., and Uitti, R. J. (2016). Biomarkers in Parkinson's Disease: Advances and Strategies. Park. Relat. Disord. 22, s106–s110. doi:10.1016/j.parkreldis.2015.09.048
Govindan, S., Amirthalingam, M., Duraisamy, K., Govindhan, T., Sundararaj, N., and Palanisamy, S. (2018). Phytochemicals-induced Hormesis Protects Caenorhabditis elegans against α-synuclein Protein Aggregation and Stress through Modulating HSF-1 and SKN-1/Nrf2 Signaling Pathways. Biomed. Pharmacother. 102, 812–822. doi:10.1016/j.biopha.2018.03.128
Han, X., Yuan, Y., and Shi, Z. (2019). Rhodium-Catalyzed Selective C-H Trideuteromethylation of Indole at C7 Position Using Acetic-D6 Anhydride. J. Org. Chem. 84, 12764–12772. doi:10.1021/acs.joc.9b01114
Hirsch, E. C., Jenner, P., and Przedborski, S. (2012). Pathogenesis of Parkinson's Disease. Mov. Disord. 28, 24–30. doi:10.1002/mds.25032
Huang, D., Ou, B., Hampsch-Woodill, M., Flanagan, J. A., and Prior, R. L. (2002). High-throughput Assay of Oxygen Radical Absorbance Capacity (ORAC) Using a Multichannel Liquid Handling System Coupled with a Microplate Fluorescence Reader in 96-well Format. J. Agric. Food Chem. 50, 4437–4444. doi:10.1021/jf0201529
Jadiya, P., Khan, A., Sammi, S. R., Kaur, S., Mir, S. S., and Nazir, A. (2011). Anti-Parkinsonian Effects of Bacopa Monnieri: Insights from Transgenic and Pharmacological Caenorhabditis elegans Models of Parkinson's Disease. Biochem. Biophysical Res. Commun. 413, 605–610. doi:10.1016/j.bbrc.2011.09.010
Jankovic, J., and Stacy, M. (2007). Medical Management of Levodopa-Associated Motor Complications in Patients with Parkinson?s Disease. Cns Drugs 21, 677–692. doi:10.2165/00023210-200721080-00005
Jia, W., Su, Q., Cheng, Q., Peng, Q., Qiao, A., Luo, X., et al. (2021). Neuroprotective Effects of Palmatine via the Enhancement of Antioxidant Defense and Small Heat Shock Protein Expression in Aβ-Transgenic Caenorhabditis elegans. Oxidative Med. Cell. Longev. 2021, 1–18. doi:10.1155/2021/9966223
Lee, S. H., Han, Y. T., and Cha, D. S. (2021). Neuroprotective Effect of Damaurone D in a C. elegans Model of Parkinson's Disease. Neurosci. Lett. 747, 135623. doi:10.1016/j.neulet.2021.135623
Lei, Z.-Q., Pan, F., Li, H., Li, Y., Zhang, X.-S., Chen, K., et al. (2015). Group Exchange between Ketones and Carboxylic Acids through Directing Group Assisted Rh-Catalyzed Reorganization of Carbon Skeletons. J. Am. Chem. Soc. 137, 5012–5020. doi:10.1021/ja512003d
Liu, J., Banskota, A., Critchley, A., Hafting, J., and Prithiviraj, B. (2015). Neuroprotective Effects of the Cultivated Chondrus Crispus in a C. elegans Model of Parkinson's Disease. Mar. Drugs 13, 2250–2266. doi:10.3390/md13042250
Ma, J., Wang, R., Chen, T., Jiang, S., and Xu, A. (2021). Protective Effects of Baicalin in a Caenorhabditis elegans Model of Parkinson's Disease. Toxicol. Res. 10, 409–417. doi:10.1093/toxres/tfaa107
Mann, D. M. A., and Yates, P. O. (1982). Pathogenesis of Parkinson's Disease. Archives Neurology 39, 545–549. doi:10.1001/archneur.1982.00510210015004
Martinez, B. A., Caldwell, K. A., and Caldwell, G. A. (2017). C . Elegans as a Model System to Accelerate Discovery for Parkinson Disease. Curr. Opin. Genet. Dev. 44, 102–109. doi:10.1016/j.gde.2017.02.011
Moosmann, B., and Behl, C. (2002). Antioxidants as Treatment for Neurodegenerative Disorders. Expert Opin. Investigational Drugs 11, 1407–1435. doi:10.1517/13543784.11.10.1407
Nass, R., and Chen, L. (2008). Caenorhabditis elegans Models of Human Neurodegenerative Diseases. Humana Press 6615, 91–101. 978-1-58829-933-8. doi:10.1007/978-1-59745-285-4_12
Olzmann, J. A., Bordelon, J. R., Muly, E. C., Rees, H. D., Levey, A. I., Li, L., et al. (2007). Selective Enrichment of DJ-1 Protein in Primate Striatal Neuronal Processes: Implications for Parkinson's Disease. J. Comp. Neurol. 500, 585–599. doi:10.1002/cne.21191
Ou, B., Huang, D., Hampsch-Woodill, M., Flanagan, J. A., and Deemer, E. K. (2002). Analysis of Antioxidant Activities of Common Vegetables Employing Oxygen Radical Absorbance Capacity (ORAC) and Ferric Reducing Antioxidant Power (FRAP) Assays: a Comparative Study. J. Agric. Food Chem. 50, 3122–3128. doi:10.1021/jf0116606
Pohanka, M. (2011). Alzheimer's Disease and Related Neurodegenerative Disorders: Implication and Counteracting of Melatonin. Jab 9, 185–196. doi:10.2478/v10136-011-0003-6
Qiu, X., Wang, P., Wang, D., Wang, M., Yuan, Y., and Shi, Z. (2019). PIII -Chelation-Assisted Indole C7-Arylation, Olefination, Methylation, and Acylation with Carboxylic Acids/Anhydrides by Rhodium Catalysis. Angew. Chem. Int. Ed. 58, 1504–1508. doi:10.1002/anie.201813182
Rudnitskaya, E. A., Maksimova, K. Y., Muraleva, N. A., Logvinov, S. V., Yanshole, L. V., Kolosova, N. G., et al. (2015). Beneficial Effects of Melatonin in a Rat Model of Sporadic Alzheimer's Disease. Biogerontology 16, 303–316. doi:10.1007/s10522-014-9547-7
Sawin, E. R., Ranganathan, R., and Horvitz, H. R. (2000). C. elegans Locomotory Rate Is Modulated by the Environment through a Dopaminergic Pathway and by Experience through a Serotonergic Pathway. Neuron 26, 619–631. doi:10.1016/S0896-6273(00)81199-X
Sayre, L. M., Perry, G., and Smith, M. A. (2008). Oxidative Stress and Neurotoxicity. Chem. Res. Toxicol. 21, 172–188. doi:10.1021/tx700210j
Schaich, K. M., Tian, X., and Xie, J. (2015). Hurdles and Pitfalls in Measuring Antioxidant Efficacy: A Critical Evaluation of ABTS, DPPH, and ORAC Assays. J. Funct. Foods 14, 111–125. doi:10.1016/j.jff.2015.01.043
Skene, D., and Swaab, D. F. (2003). Melatonin Rhythmicity: Effect of Age and Alzheimer's Disease. Exp. Gerontol. 38, 199–206. doi:10.1016/S0531-5565(02)00198-5
Smeyne, R. J., and Jackson-Lewis, V. (2005). The MPTP Model of Parkinson's Disease. Mol. Brain Res. 134, 57–66. doi:10.1016/j.molbrainres.2004.09.017
Sommer, J., Kunzmann, A., Stuthmann, L. E., and Springer, K. (2022). The Antioxidative Potential of Sea Grapes (Caulerpa Lentillifera, Chlorophyta) Can Be Triggered by Light to Reach Comparable Values of Pomegranate and Other Highly Nutritious Fruits. Plant Physiol. Rep. 27, 186–191. doi:10.1007/s40502-021-00637-6
Tucci, M. L., Harrington, A. J., Caldwell, G. A., and Caldwell, K. A. (2011). Modeling Dopamine Neuron Degeneration in Caenorhabditis elegans. Humana Press 793, 129–148. doi:10.1007/978-1-61779-328-8_9
Verma, A., and Ravindranath, V. (2019). CaV1.3 L-type Calcium Channels Increase the Vulnerability of Substantia Nigra Dopaminergic Neurons in MPTP Mouse Model of Parkinson's Disease. Front. Aging Neurosci. 11, 382. doi:10.3389/fnagi.2019.00382
Weber, C. A., and Ernst, M. E. (2006). Antioxidants, Supplements, and Parkinson's Disease. Ann. Pharmacother. 40, 935–938. doi:10.1345/aph.1G551
Wu, Y.-H., Feenstra, M. G. P., Zhou, J.-N., Liu, R.-Y., Toranõ, J. S., Van Kan, H. J. M., et al. (2003). Molecular Changes Underlying Reduced Pineal Melatonin Levels in Alzheimer Disease: Alterations in Preclinical and Clinical Stages. J. Clin. Endocrinol. Metabolism 88, 5898–5906. doi:10.1210/jc.2003-030833
Wu, Y., Wu, Z., Butko, P., Christen, Y., Lambert, M. P., Klein, W. L., et al. (2006). Amyloid- -Induced Pathological Behaviors Are Suppressed by Ginkgo Biloba Extract EGb 761 and Ginkgolides in Transgenic Caenorhabditis elegans. J. Neurosci. 26, 13102–13113. doi:10.1523/JNEUROSCI.3448-06.2006
Yu, C.-X., Zhu, C.-B., Xu, S.-F., Cao, X.-D., and Wu, G.-C. (2000). Selective MT2 Melatonin Receptor Antagonist Blocks Melatonin-Induced Antinociception in Rats. Neurosci. Lett. 282, 161–164. doi:10.1016/S0304-3940(00)00883-1
Zarranz, J. J., Alegre, J., Gómez-Esteban, J. C., Lezcano, E., Ros, R., Ampuero, I., et al. (2004). The New Mutation, E46K, of α-synuclein Causes Parkinson and Lewy Body Dementia. Ann. Neurol. 55, 164–173. doi:10.1002/ana.10795
Zhao, S., Cheng, Q., Peng, Q., Yu, X., Yin, X., Liang, M., et al. (2018). Antioxidant Peptides Derived from the Hydrolyzate of Purple Sea Urchin (Strongylocentrotus Nudus) Gonad Alleviate Oxidative Stress in Caenorhabditis elegans. J. Funct. Foods 48, 594–604. doi:10.1016/j.jff.2018.07.060
Keywords: derivative of melatonin, synthesis, antioxidant activity, C. elegans, Parkinson’s disease
Citation: He L, Du J-J, Zhou J-J, Chen M-T, Luo L, Li B-Q, Zhang X-Z, Ma W-Z, Ma A-J and Feng N (2022) Synthesis of Melatonin Derivatives and the Neuroprotective Effects on Parkinson’s Disease Models of Caenorhabditis elegans. Front. Chem. 10:918116. doi: 10.3389/fchem.2022.918116
Received: 12 April 2022; Accepted: 28 April 2022;
Published: 08 June 2022.
Edited by:
Xi Zheng, The State University of New Jersey, United StatesCopyright © 2022 He, Du, Zhou, Chen, Luo, Li, Zhang, Ma, Ma and Feng. This is an open-access article distributed under the terms of the Creative Commons Attribution License (CC BY). The use, distribution or reproduction in other forums is permitted, provided the original author(s) and the copyright owner(s) are credited and that the original publication in this journal is cited, in accordance with accepted academic practice. No use, distribution or reproduction is permitted which does not comply with these terms.
*Correspondence: Ai-Jun Ma, d3l1Y2hlbW1hakAxMjYuY29t; Na Feng, d3l1Y2hlbWZuQDEyNi5jb20=