- 1State Key Laboratory of Silicate Materials for Architectures, Wuhan University of Technology, Wuhan, China
- 2State Key Laboratory of Advanced Technology for Materials Synthesis and Processing, Wuhan University of Technology, Wuhan, China
- 3School of Chemistry, Chemical Engineering and Life Science, Wuhan University of Technology, Wuhan, China
- 4Department of Histology and Embryology, Tongji Medical College, Huazhong University of Science and Technology, Wuhan, China
Low or excessively high concentration of S-vacancy (CS-vacancy) is disadvantageous for the hydrogen evolution reaction (HER) activity of MoS2-based materials. Additionally, alkaline water electrolysis is most likely to be utilized in the industry. Consequently, it is of great importance for fine-tuning CS-vacancy to significantly improve alkaline hydrogen evolution. Herein, we have developed a one-step Ru doping coupled to compositing with CoS2 strategy to precisely regulate CS-vacancy of MoS2-based materials for highly efficient HER. In our strategy, Ru doping favors the heterogeneous nucleation and growth of CoS2, which leads to a high crystallinity of Ru-doped CoS2 (Ru-CoS2) and rich heterogeneous interfaces between Ru-CoS2 and Ru-doped MoS2-x (Ru-MoS2-x). This facilitates the electron transfer from Ru-CoS2 to Ru-MoS2-x, thereby increasing CS-vacancy of MoS2-based materials. Additionally, the electron injection effect increases gradually with an increase in the mass of Co precursor (mCo), which implies more S2- leaching from MoS2 at higher mCo. Subsequently, CS-vacancy of the as-synthesized samples is precisely regulated by the synergistic engineering of Ru doping and compositing with CoS2. At CS-vacancy = 17.1%, a balance between the intrinsic activity and the number of exposed Mo atoms (EMAs) to boost highly active EMAs should be realized. Therefore, the typical samples demonstrate excellent alkaline HER activity, such as a low overpotential of 170 mV at 100 mA cm−2 and a TOF of 4.29 s−1 at -0.2 V. Our results show promise for important applications in the fields of electrocatalysis or energy conversion.
Introduction
The rapid development of the economy has made the fast consumption of fossil energy, resulting in the energy crisis and severe environmental pollution (Xie et al., 2013b; Xu et al., 2017; Jiang et al., 2022). Hydrogen energy, as one of the clean and renewable energies, has received extensive attention around the world (Huang et al., 2019; Venkateshwaran and Senthil Kumar, 2019; Zhu et al., 2019; Wang F. et al., 2020). Electrocatalytic water splitting (Lin et al., 2017; Lin et al., 2019; Djara et al., 2020; Liu L. et al., 2022) is regarded as an eco-friendly technology for hydrogen (H2) production. So far, platinum-based materials are still the best electrocatalysts for acidic water electrolysis (Chen et al., 2020). Nevertheless, the high cost and low abundance seriously restrict the wide applications of such precious metals (Li et al., 2011; Qi et al., 2019; Jing et al., 2020). On the other hand, alkaline water electrolysis is most likely to be utilized in the industry, owing to the unrestricted reactant availability, desirable safety, and satisfactory output (Yin et al., 2015). However, the sluggish dynamics of hydrogen evolution reaction (HER) in alkaline environments results in excessive energy consumption (Yin et al., 2015). Therefore, it is of great significance for the development of highly efficient and low-cost electrocatalysts to overcome energy barriers for accelerating kinetics and to decrease overpotential during the hydrogen evolution reaction (HER) process.
It is well known that molybdenum disulfide (MoS2) can be designed as an alternative to Pt due to the excellent H* adsorption–desorption properties, special layered structures, good stability, and low cost (Hinnemann et al., 2005; Deng et al., 2017; Wang Y. et al., 2019; Gao et al., 2020). However, the pristine 2H-MoS2 tends to aggregate under van der Waals forces, resulting in poor edge active sites (Kibsgaard et al., 2012; Wang et al., 2017; Wang et al., 2021b). More importantly, there remain a lot of active sites from the vast basal planes of such MoS2 to be developed (Kibsgaard et al., 2012; Wang et al., 2017; Wang et al., 2021b). To address these drawbacks, defect-rich, double-gyroid, and amorphous structures have been introduced into such MoS2 nanosheets to increase the unsaturated sulfur atoms as active sites for HER (Kibsgaard et al., 2012; Xie et al., 2013a; Liu et al., 2018). Doping transition metals (TMs) into MoS2 is another important method for the advancement of electrocatalysis. This is because doping TMs not only increases the number of unsaturated sulfur atoms but also regulates the adsorption free energy of hydrogen atoms (ΔGH) of active sites to favor HER (Wang et al., 2015; Wang D. et al., 2019; Wang et al., 2021b). Among these TMs, ruthenium (Ru) belongs to the Pt group, but its price is as low as about 5% of Pt (Zhang J. et al., 2019). A quintessential example demonstrates that ΔGH at the Ru-doped in-plane sulfur sites decreases to approximately 0.19 eV (Zhang X. et al., 2019). This indicates that doping Ru can efficiently modulate the electronic features of the adjacent sulfur atoms, thereby leading to the optimal H atom binding energy (Yan et al., 2018). On the other hand, after doping Ru into 2H-MoS2 (Li J. et al., 2021) or 2H-WS2 (Li J. et al., 2022), these Ru sites can significantly accelerate the water dissociation in alkaline environments. For example, the energy barrier of the H−OH cleavage (E) is as high as 2.42 eV before doping Ru. Remarkably, E of such Ru sites decreases to 2.02 eV, which is advantageous for water dissociation to OH and H intermediates.
Modulating S-vacancy into MoS2-based materials has been developed as an efficient strategy (Tsai et al., 2017; Park et al., 2018; Li Y. et al., 2021) to activate inert basal planes because the exposed Mo atoms can be tailored into newborn active sites (Wang X. et al., 2020). Following this opinion, much effort has so far been devoted to introducing S-vacancy into the basal planes of mono-layered or multi-layered 2H-MoS2 nanosheets (Li et al., 2016b; Tsai et al., 2017; Park et al., 2018; Li et al., 2019; Wang X. et al., 2020). Among these strategies, chemical vapor deposition coupled to plasma has been developed to modulate S-vacancy into MoS2 (Li et al., 2016b). In this case, S atoms escape from the MoS2 lattice more easily than Mo atoms due to the lower formation energy of S-vacancy compared to that of the Mo interstitial (Li et al., 2016b). Subsequently, the controllable electrochemical preparation of S-vacancy for multi-layered MoS2 has been proposed by simply adjusting desulfurization parameters, such as desulfurization potential and time (Tsai et al., 2017). More recently, the single Ru atom doping technology promotes the phase transition of 2H-MoS2 and the formation of S-vacancy, which greatly enhances its HER activity (Zhang J. et al., 2019). Nevertheless, a low or excessively high concentration of S-vacancy (CS-vacancy) is disadvantageous for the hydrogen evolution reaction (HER) activity of MoS2-based materials. Consequently, it is of great importance for the development of a novel approach to fine-tuning CS-vacancy to significantly improve alkaline hydrogen evolution. In addition, the aforementioned electrocatalysts based on S-vacancy only consist of a single component (that is, MoS2) rather than hybrid catalysts. As one of the traditional semiconductor materials, MoS2 suffers from another drawback of unsatisfactory charge-transfer resistance (RCT), leading to low HER activity (Li Y. et al., 2021; Wang et al., 2021b; Wang et al., 2021c). Hence, compositing with the metallic phase will favor fast electrode kinetics, realizing synergistically regulating CS-vacancy and RCT of MoS2-based electrocatalysts for highly efficient HER.
Herein, we develop a one-step Ru doping coupled to compositing with the CoS2 strategy to synergistically regulate CS-vacancy of MoS2-based materials for highly efficient alkaline HER. Ru doping is advantageous for the formation of S-vacancy in the basal planes of MoS2. On the other hand, Ru doping favors heterogeneous nucleation and growth of CoS2, which leads to rich heterogeneous interfaces between Ru-doped CoS2 (Ru-CoS2) and Ru-doped MoS2-x (Ru-MoS2-x). This facilitates the electron transfer from Ru-CoS2 to Ru-MoS2-x, thereby increasing CS-vacancy of MoS2-based materials. At fixed Ru dopant, the electron injection effect increases gradually with an increase in the mass of Co precursor, which means more S2- escaping from Ru-MoS2 nanosheets. Therefore, synergistically regulating CS-vacancy of the as-synthesized samples, from 2.1 to 27.5%, is realized by a new one-step Ru doping coupled to compositing with the CoS2 strategy. On regulating CS-vacancy to 17.1%, a balance between the intrinsic activity and the number of exposed Mo atoms (EMAs) to boost highly active EMAs should be realized. As a consequence, the typical samples demonstrate the optimal alkaline HER activity among all samples, such as a low overpotential of 170 mV at 100 mA cm−2, a large specific alkaline HER current density of 77.6 μA cm−2, and a turnover frequency of 4.29 s−1 at -0.2 V as well as excellent long-term stability. Our results pave a novel approach to unlocking the potential of inert basal planes in MoS2-based materials for highly efficient HER and promise important applications in the field of electrocatalytic hydrogen evolution.
Experimental Section
Fabrication of the Typical Samples (Ru-MoS2-X-CoS2/CC)
The typical samples were synthesized by a one-pot hydrothermal strategy using the following precursors such as Na2MoO4.2H2O, CH4N2S, and Co(NO3)2·6H2O. In the typical experiments, 160 mg Co(NO3)2·6H2O, 160 mg Na2MoO4.2H2O, and 600 mg CH4N2S were dissolved in 46.0 ml deionized water under magnetic stirring. Subsequently, 4.0 ml RuCl3 solution (5 mmol L−1) was introduced into the above cobalt salt solution under magnetic stirring for 0.5 h. Carbon cloth (CC, 4 cm2) was pretreated according to the related literature (Yu et al., 2015). Then, the above solution and the pretreated CC were transferred into a 100.0 ml Teflon-lined stainless-steel autoclave and heated to 200°C for 20 h. After the hydrothermal reaction, the mixture was cooled to room temperature. The typical samples were harvested after being washed with water thoroughly and vacuum-dried at 60 °C for 12.0 h and abbreviated as Ru-MoS2-x-CoS2/CC.
Fabrications of Other Ru-MoS2-X-CoS2/CC Samples
Other Ru-MoS2-x-CoS2/CC samples were synthesized at various volumes of RuCl3 solution (5 mmol L−1) of 1.0, 7.0, 10.0, and 30.0 ml under otherwise similar conditions as the typical experiments. These Ru-MoS2-x-CoS2/CC samples are denoted as Ru-MoS2-x-CoS2/CC-1.0, Ru-MoS2-x-CoS2/CC-7.0, Ru-MoS2-x-CoS2/CC-10.0, and Ru-MoS2-x-CoS2/CC-30.0.
In addition, Ru-MoS2-x-CoS2/CC-80, Ru-MoS2-x-CoS2/CC-240, Ru-MoS2-x-CoS2/CC-280, and Ru-MoS2-x-CoS2/CC-320 were synthesized at various masses of Co(NO3)2·6H2O of 80, 240, 280, and 320 mg under otherwise similar conditions of the typical experiments, respectively.
Figure 1 displays a schematic representation of the fabrication of all Ru-MoS2-x-CoS2/CC samples.
Fabrications of Ru-MoS2/CC, MoS2/CC, MoS2-CoS2/CC, and Ru-CoS2/CC
Ru-doped MoS2 nanosheets assembled on CC are abbreviated as Ru-MoS2/CC. Fabrication of Ru-MoS2/CC is almost the same as the typical samples except for the absence of Co(NO3)2.
MoS2 nanosheets assembled on CC are abbreviated as MoS2/CC. Fabrication of MoS2/CC is almost the same as Ru-MoS2/CC except for the absence of RuCl3 solution.
MoS2 nanosheets coated with CoS2 assembled on CC are abbreviated as MoS2-CoS2/CC. Fabrication of MoS2-CoS2/CC is almost the same as the typical samples except for the absence of RuCl3 solution.
Ru-doped CoS2 assembled on CC are abbreviated as Ru-CoS2/CC. Fabrication of Ru-CoS2/CC is almost similar to the typical samples except for the absence of Na2MoO4.2H2O.
Other experimental details about materials, characterization, and performance measurements are supplied in Supplementary Material S1.
Results and Discussion
Characterization of the Typical Samples
Scanning electron microscopic (SEM) images display the morphology of the typical samples in Supplementary Figures SJ–L. From these figures, it can be observed that lots of nanosheets are grown on the smooth surface of CC (Supplementary Figure S2). Powder X-ray diffraction (XRD) pattern of Ru-MoS2-x-CoS2/CC (Figure 2A) shows the diffraction peaks located at 2θ = 14.1°, 32.7°, and 58.8°, matching well with the (002), (100), and (110) planes of 2H-MoS2 (PDF#75-1539) (Nguyen et al., 2021), respectively. Other sharp diffraction peaks of Ru-MoS2-x-CoS2/CC, such as 32.4° and 55.1°, are attributed to the (200) and 311) planes of CoS2 (PDF#70-2865) (Yao et al., 2019), respectively, suggesting the presence of highly crystallized CoS2 besides 2H-MoS2. From Figure 2A, we also observe the diffraction peak at 26.2° originating from CC. The Raman spectrum of Ru-MoS2-x-CoS2/CC (Supplementary Figure S3) displays the typical E12g and A1g vibration models of the Mo-S bonds, further verifying the phase structure of 2H-MoS2 in Ru-MoS2-x-CoS2/CC. As shown in Figure 2B, the Ru 3p high-resolution X-ray photoelectron spectroscopy (XPS) spectrum of Ru-MoS2-x-CoS2/CC is divided into two characteristic peaks at the binding energies of 462.4 and 484.7 eV, corresponding to Ru 3p3/2 and Ru 3p1/2 (Ge et al., 2022). Nevertheless, no peak of Ru is detected in MoS2 nanosheets coated with CoS2 assembled on CC (MoS2-CoS2/CC) in Figure 2B. Furthermore, Figures 2C, D exhibit Co 2p and Mo 3 day XPS spectra of Ru-MoS2-x-CoS2/CC, respectively. It is seen that Co 2p and Mo 3 day peaks positively shift by about 0.27 and 0.15 eV compared to those of MoS2-CoS2/CC (Hao et al., 2017), respectively. Additionally, the characteristic peaks about Ru2S3 or RuCl3 are not observed in the XRD pattern of Ru-MoS2-x-CoS2/CC (Figure 2A). Therefore, these data confirm the successful doping of Ru into the following two phases of Ru-MoS2-x-CoS2/CC, 2H-MoS2, and CoS2.
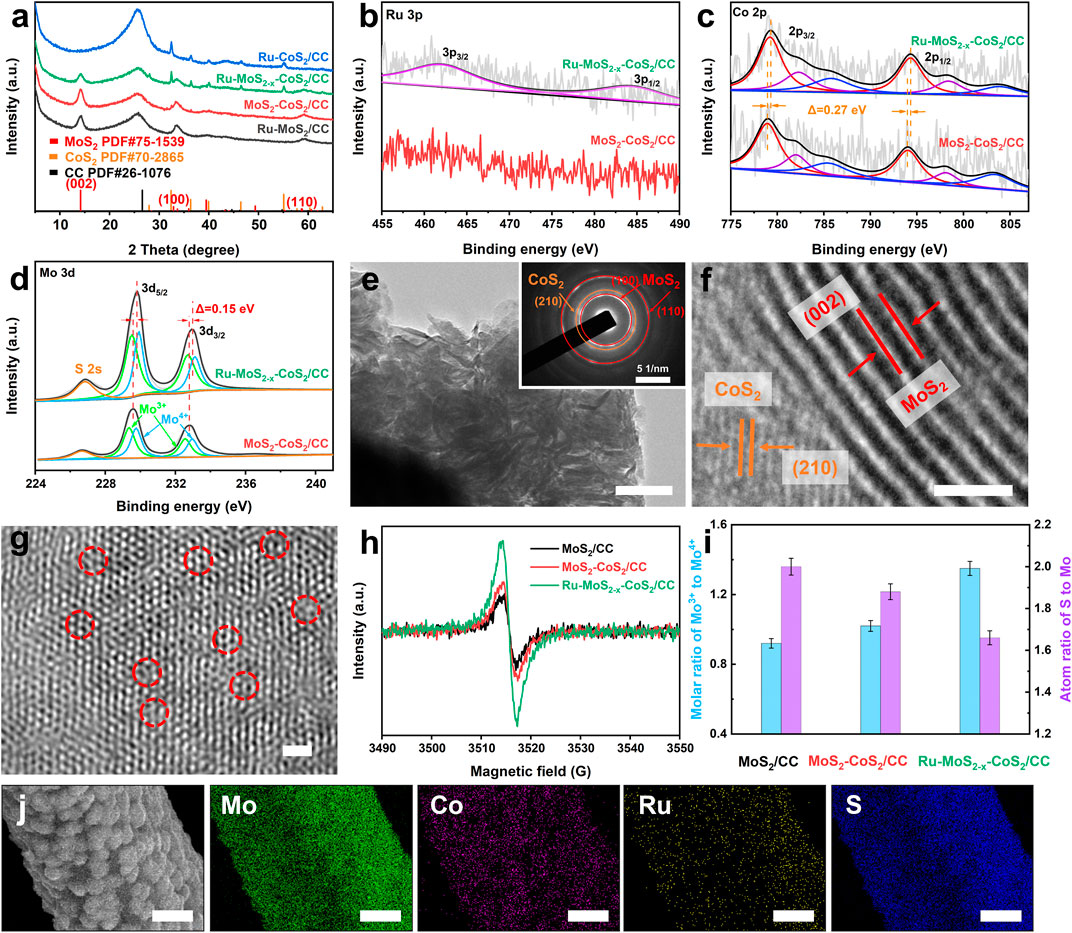
FIGURE 2. (A) XRD patterns of Ru-MoS2/CC, MoS2-CoS2/CC, Ru-CoS2/CC, and the typical samples; (B) Ru 3p, (C) Co 2p, and (D) Mo3d high-resolution XPS spectra of the typical samples and MoS2-CoS2/CC; (E) TEM image and (F,G) HR-TEM images of the typical samples; (H) EPR spectra, and (I) molar ratio of Mo3+ to Mo4+, and the atom ratio of S to Mo of MoS2/CC, MoS2-CoS2/CC, and the typical samples. (J) EDX mapping profiles of Mo, Co, Ru, and S over the typical samples. The inset of panel (E) is the SAED pattern of the typical samples. Scale bars of panels (E), (F), (G), and (J) are 100 nm, 2 nm, 2 nm, and 2 μm, respectively.
Nanosheets of Ru-MoS2-x-CoS2/CC are also clearly observed from transmission electron microscopic (TEM) image (Figure 2E), which is consistent with the results of SEM images. According to the high-resolution TEM (HR-TEM) image (Figure 2F), the lattice spacings of 0.625 and 0.245 nm that are seen in Ru-MoS2-x-CoS2/CC correspond to the (002) plane of MoS2 and the (210) plane of CoS2 (He et al., 2020; Liu X. et al., 2022), respectively. These also conform to the results of XRD. As we know, MoS2 is regarded as one of the traditional semiconductor materials, and CoS2 is a metallic phase due to its high Fermi level. Therefore, there are lots of Schottky heterojunctions among Ru-MoS2-x-CoS2/CC. In addition, the inset of Figure 2E (selected area electron diffraction, SAED) pattern indicates that Ru-doped MoS2 (Ru-MoS2) and Ru-doped CoS2 (Ru-CoS2) of Ru-MoS2-x-CoS2/CC are polycrystalline (Huang et al., 2015). We can clearly observe that many defects exist in such Ru-MoS2 from Figure 2G due to lattice distortion by Ru doping (Zhang J. et al., 2019) and the electron injection effect (Gan et al., 2018; Zhang J. et al., 2019). Energy-dispersive X-ray (EDX) spectroscopy mapping (Figure 2J) profiles further confirm the uniform distribution of Mo, Co, Ru, and S elements throughout Ru-MoS2-x-CoS2/CC. Considering that Ru-MoS2 assembled on CC (Ru-MoS2/CC) and Ru-CoS2 assembled on CC (Ru-CoS2/CC) tend to form nanosheets (Supplementary Figures S1B, C) and nanoparticles (Supplementary Figures SH, I), respectively, and that Ru-CoS2 nucleates and grows prior to Ru-MoS2 (Figures 4A–F), we can reasonably deduce as follows: during the hydrothermal process, Ru-CoS2 nanoparticles are firstly assembled on CC; then, Ru-CoS2/CC are densely coated by Ru-MoS2 nanosheets to construct the typical samples, Ru-MoS2-x-CoS2/CC.
According to XPS data in Figure 2D and Supplementary Figure S4, we characterize molar ratios of Mo3+ to Mo4+ of MoS2/CC, MoS2-CoS2/CC, and Ru-MoS2-x-CoS2/CC in Figure 2I. The presence of Mo3+ can induce S-vacancy of the basal planes in Ru-MoS2 (Ma et al., 2020) of Ru-MoS2-x-CoS2/CC. From Figure 2I, Ru-MoS2-x-CoS2/CC exhibits a higher value of about the molar ratio of Mo3+ to Mo4+ than MoS2/CC or MoS2-CoS2/CC, reaching 1.35. To confirm such defective structures, electron paramagnetic resonance (EPR) (Liu et al., 2017; Gong et al., 2020; Wang J. et al., 2021) is further employed to estimate the S-vacancy of all samples. As expected, Ru-MoS2-x-CoS2/CC demonstrates the highest EPR signal at g = ∼ 2.002 among all samples (Figure 2H). Moreover, the EPR signal of MoS2-CoS2/CC is higher than that of MoS2/CC. These data straightforwardly indicate that the formation of S-vacancy might be closely related to both Ru doping into MoS2 and compositing with CoS2.
Doping Ru Coupled to Compositing With CoS2 to Regulate Microstructures of the As-synthesized Samples
First, a series of Ru-MoS2/CC samples were synthesized at the various volumes of RuCl3 solution (V) of 1.0, 4.0, 7.0, 10.0, and 30.0 ml under otherwise similar conditions of the typical experiments except for the absence of Co(NO3)2. These Ru-MoS2/CC samples are denoted as Ru-MoS2/CC-1.0, Ru-MoS2/CC-7.0, Ru-MoS2/CC-10.0, and Ru-MoS2/CC-30.0. Similar Ru 3p XPS spectra to the typical samples are observed in Supplementary Figure S5A, which is responsible for successfully doping Ru into all Ru-MoS2/CC samples. In addition, the atom ratios of Ru to Mo (A) of all Ru-MoS2/CC samples are characterized by XPS in Supplementary Figure S5B. From this figure, A increases with increasing V, indicating that more Ru will be doped into the Ru-MoS2/CC samples at higher V. In this work, the atomic ratio of S to Mo (S: Mo) of MoS2/CC is firstly measured (Supplementary Table S1) and is normalized to 2.00. Then, it is employed as a reference to confirm the normalized S: Mo of MoS2-x in all Ru-MoS2/CC samples in terms of XPS data (Xu et al., 2016; Wang J. et al., 2021). Here, the measured S: Mo for all Ru-MoS2/CC samples are the atom ratios of S minus double Ru to Mo, which is abbreviated as [(S–2Ru)/Mo]. Therefore, the normalized S: Mo of MoS2-x in all samples are listed in Supplementary Table S2. From Figure 3E, CS-vacancy of Ru-MoS2/CC samples increases with the increase in Ru dopants. For example, CS-vacancy of Ru-MoS2/CC-30.0 increases to 10.5% at V = 30.0 ml. That is to say, doping Ru can regulate CS-vacancy of Ru-MoS2/CC samples varying from 2.1 to 10.5%.
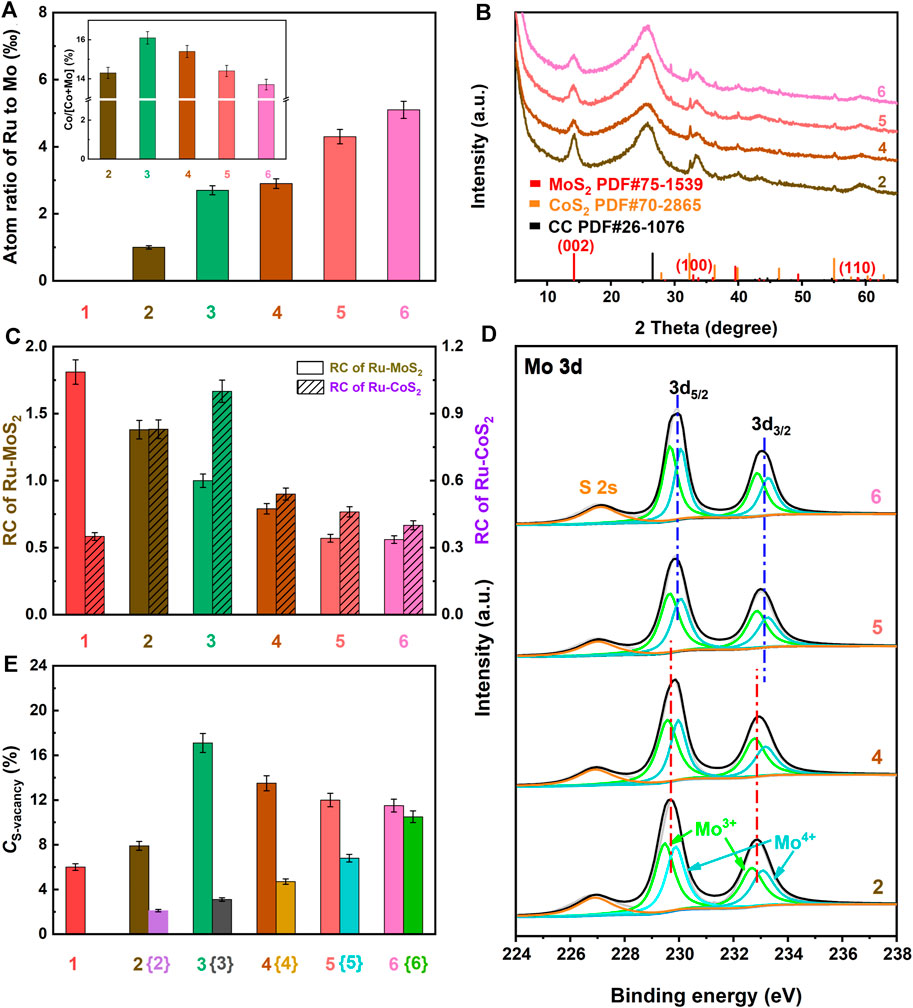
FIGURE 3. (A) Atom ratio of Ru to Mo based on ICP-OES, (B) XRD patterns, (C) RC of Ru-MoS2-x and Ru-CoS2, (D) Mo3d high-resolution XPS spectra, and (E) CS-vacancy of the related samples. Meanwhile, 1, 2, 3, 4, 5 and 6 are denoted as MoS2-CoS2/CC, Ru-MoS2-x-CoS2/CC-1.0, the typical samples, Ru-MoS2-x-CoS2/CC-7.0, Ru-MoS2-x-CoS2/CC-10.0, and Ru-MoS2-x-CoS2/CC-30.0, respectively. Fabrications of {2}, {3}, {4}, {5}, and {6} are the same as those of Ru-MoS2-x-CoS2/CC-1.0, the typical samples, Ru-MoS2-x-CoS2/CC-7.0, Ru-MoS2-x-CoS2/CC-10.0, and Ru-MoS2-x-CoS2/CC-30.0 except for the absence of Co(NO3)2, to be denoted as Ru-MoS2/CC-1.0, Ru-MoS2/CC, Ru-MoS2/CC-7.0, Ru-MoS2/CC-10.0, and Ru-MoS2/CC-30.0, respectively. The inset of panel (A) is the atom ratio of Co to (Co + Mo) of the related samples based on ICP-OES.
Density functional theory (DFT) calculation reveals that the Ru-S bonding energy (about 0.92 eV) decreases by 0.87 eV compared to the Mo-S bonding energy (about 1.79 eV) (Luo et al., 2020), which is advantageous for the formation of S-vacancy in the basal planes of MoS2. In this work, Ru atoms substituting for Mo atoms in MoS2 are abbreviated as Ru(Mo). According to the previously reported literature (Zhang J. et al., 2019; Zhang X. et al., 2019), Ru doping results in an increase in CS-vacancy due to the strong attractive interaction between Ru(Mo) and the adjacent S-vacancy. Furthermore, S-vacancy is exclusively stable around Ru(Mo) (Kang, 2021).
To investigate the synergistic effect of doping Ru coupled to compositing with CoS2 on the microstructure, other Ru-MoS2-x-CoS2/CC samples are further synthesized at various V of 1.0, 7.0, 10.0, and 30.0 ml under otherwise similar conditions of the typical experiments to be denoted as Ru-MoS2-x-CoS2/CC-1.0, Ru-MoS2-x-CoS2/CC-7.0, Ru-MoS2-x-CoS2/CC-10.0, and Ru-MoS2-x-CoS2/CC-30.0, respectively. According to Supplementary Figure S6, these Ru-MoS2-x-CoS2/CC samples almost display the same Ru 3p XPS spectra as the typical samples, indicating a successful doping of Ru into them as well. The values of A of all samples are characterized by inductively coupled plasma-optical emission spectroscopy (ICP-OES). In terms of Figure 3A, the values of A of these samples increase with the increase in V. Moreover, other Ru-MoS2-x-CoS2/CC samples also exhibit the same phase structure as the typical samples, since the XRD pattern of each sample is similar to that of the typical samples (Figure 3B). Figure 3C further presents the relative crystallinity (RC) of Ru-MoS2-x and Ru-CoS2 for all Ru-MoS2-x-CoS2/CC samples based on the corresponding XRD data such as the (002) plane for molybdenum disulfide and (200) (210), (211) (220), and 311) planes for cobalt disulfide. From this figure, the RC of Ru-MoS2-x decreases with the increase in V, which implies that more defective structures or S-vacancy would be modulated into the basal planes of Ru-MoS2-x at higher V. However, the influence of V on RC of Ru-CoS2 is not coincident with the trend of the former. Initially, the RC of Ru-CoS2 gradually increases with increasing V. At V = 4.0 ml, the RC of Ru-CoS2 of the typical samples exhibits the highest value among all samples. However, further increasing V leads to a decrease in the RC of Ru-CoS2.
In addition, Mo3+ can be observed in Mo 3 day XPS spectra of all Ru-MoS2-x-CoS2/CC samples (Figure 3D), implying the formation of S-vacancy of the basal planes in Ru-MoS2-x. Taking into account that MoS2-CoS2/CC and other Ru-MoS2-x-CoS2/CC samples (Supplementary Figures S1E, F; Supplementary Figure S7) are almost similar to the morphology of the typical samples (Supplementary Figures S1K, L), such core–shell structure, Ru-MoS2-x nanosheets densely coated Ru-CoS2 composites, facilitates to correctly reflect the S: Mo of Ru-MoS2-x in all samples by XPS analysis. As mentioned above, the S: Mo of MoS2/CC is also employed as a reference to confirm the normalized S: Mo of Ru-MoS2-x in all Ru-MoS2-x-CoS2/CC samples (Li et al., 2016a; Xu et al., 2016) based on XPS data (Supplementary Figure S8). Here, the measured S: Mo for all Ru-MoS2-x-CoS2/CC samples is the atom ratio of S minus double (Co + Ru) to Mo, which is abbreviated as [(S–2Co–2Ru)/Mo]. Subsequently, we further calculate the related CS-vacancy, as shown in Figure 3E; Supplementary Table S1. From Figure 3E, CS-vacancy of Ru-MoS2-x-CoS2/CC increases to 7.9% compared to that of MoS2-CoS2/CC (about 6.0%). Out of expectation, CS-vacancy of the typical samples rather than Ru-MoS2-x-CoS2/CC-30.0 reaches the highest value among all samples, about 17.1% from Figure 3E. At the same time, Ru-MoS2-x-CoS2/CC-30.0 exhibits a lower EPR signal than the typical samples (Supplementary Figure S9), further confirming fewer S-vacancy for Ru-MoS2-x-CoS2/CC-30.0 in comparison with the typical samples. In other words, at a fixed mass of Co precursor, CS-vacancy of Ru-MoS2-x can be precisely regulated from 7.9 to 17.1% by synergistic Ru doping and compositing with CoS2 engineering. As a consequence, we can infer that compositing with CoS2 should be another key factor to regulate CS-vacancy of Ru-MoS2-x nanosheets in Ru-MoS2-x-CoS2/CC samples. This is because Ru doping also affects the microstructure of CoS2, which may have a significant influence on CS-vacancy of Ru-MoS2-x nanosheets in Ru-MoS2-x-CoS2/CC samples in turn.
Following this viewpoint, we deeply investigate the influence of doping Ru on the microstructure of cobalt disulfide. As mentioned above, CoS2 nucleates and grows prior to MoS2. To eliminate the effect of MoS2 as much as possible during the characterization of microstructures of CoS2, the related samples such as [MoS2-CoS2/CC], [1] [Ru-MoS2-x-CoS2/CC], [2], [3], and [4] are synthesized at t = 6 h (Figures 4A–F) rather than 20 h. Fabrications of [MoS2-CoS2/CC], [1] [Ru-MoS2-x-CoS2/CC], [2], [3], and [4] are the same as those of MoS2-CoS2/CC, Ru-MoS2-x-CoS2/CC-1.0, the typical samples, Ru-MoS2-x-CoS2/CC-7.0, Ru-MoS2-x-CoS2/CC-10.0, and Ru-MoS2-x-CoS2/CC-30.0 except for t, respectively. Their SEM images and XRD patterns are displayed in Figures 4A–G, respectively. Besides the diffraction peak at 26.2° of CC, other peaks are indexed to CoS2 without the signal of MoS2 for all samples (Figure 4G), further confirming the heterogeneous nucleation and growth of CoS2 prior to MoS2. As shown in Figure 4A, a small number of spherical CoS2 nanoparticles are grown on CC for [MoS2-CoS2/CC]. Moreover, the similar phenomena are observed for Ru-doped samples from Figures 4B–F. Interestingly, with an increase in V, the size and number of Ru-CoS2 nanoparticles gradually increase (Figure 4B). At V = 4.0 ml, both the size and number of Ru-CoS2 nanoparticles for [Ru-MoS2-x-CoS2/CC] reach up to the maximum value (Figure 4C). However, further increasing V leads to a decrease in both the size and number of Ru-CoS2 for other [Ru-MoS2-x-CoS2/CC] (Figures 4D–F). Next, we characterize the RC of Ru-CoS2 for all samples synthesized at 6 h according to XRD data such as (200) (210), (211) (220), and (311) planes of cobalt disulfide, as shown in Figure 4H. Impressively, their trend in the variation is almost consistent with that of the RC of Ru-CoS2 of Ru-MoS2-x-CoS2/CC samples synthesized at 20 h (Figure 3C). This confirms that doping Ru can improve the crystallinity of CoS2 under certain conditions. To find out the reason for this issue, we investigate the Fourier-transforming infrared (FT-IR) spectrum of MoS2-CoS2/CC in Figure 4I. The peak located at 1020 cm−1 straightforwardly indicates the formation of CoS2 (Chakraborty et al., 2006) at V = 0.0 ml. At V increasing to 1.0 ml, the peak of Ru-MoS2-x-CoS2/CC-1.0 located at 1024 cm−1 shows a blue-shift of about 4 cm−1 compared to that of MoS2-CoS2/CC. At V = 4.0 ml, the related peak blue-shifts to about 1036 cm−1, as shown in Figure 4I. This indicates that doping Ru into CoS2 can decrease the Co−S bond length and enhance the bonding energy (Feng et al., 2018), which favors the formation of Ru-CoS2. However, a decrease in both the size and number of CoS2 with further increasing V (Figures 4D–F) can be explained as follows: introducing excessive Ru into the lattice of CoS2 may induce an increase in the oxidation state of neighboring Co ions to maintain the charge neutrality. The similar result has been observed for other transition metal compounds, such as NiO (Smyth, 2000; Ge et al., 2022). This implies that excessive dopant will hinder the formation of CoS2 in turn.
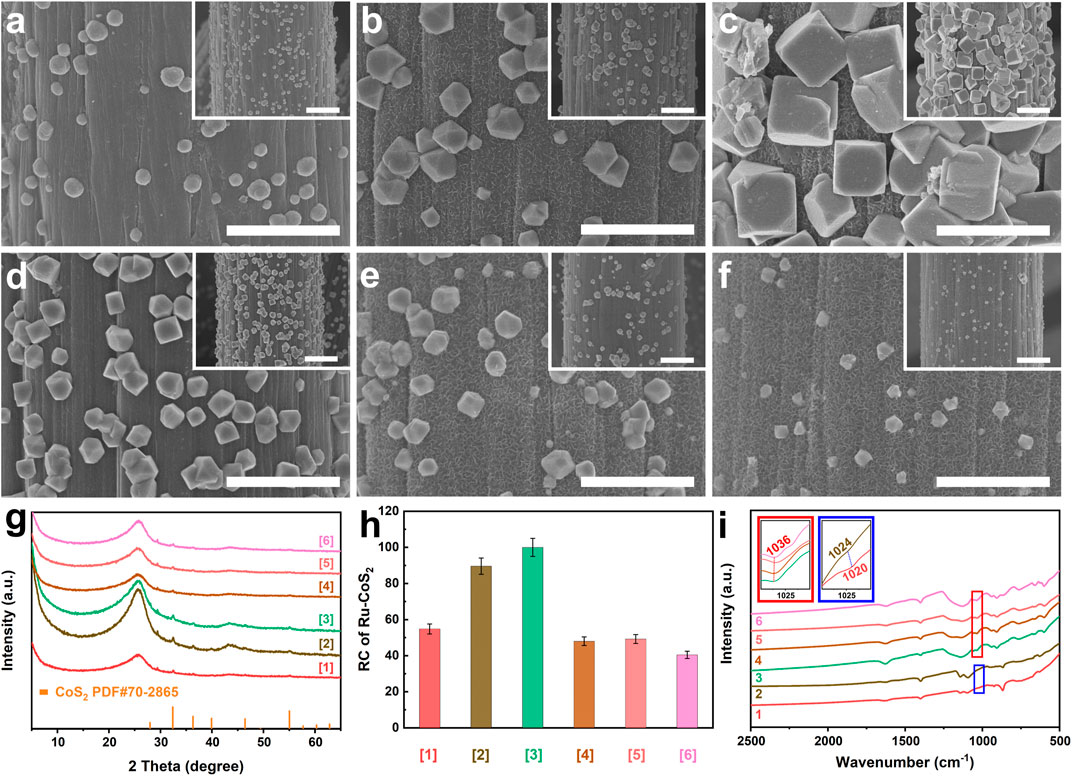
FIGURE 4. SEM images of (A) [MoS2-CoS2/CC] ([1]), (B) [Ru-MoS2-x-CoS2/CC-1.0] ([2]), (C) [the typical samples] ([3]), (D) [Ru-MoS2-x-CoS2/CC-7.0] ([4]), (E) [Ru-MoS2-x-CoS2/CC-10.0] ([5]), and (F) [Ru-MoS2-x-CoS2/CC-30.0] ([6]); (G) XRD patterns and (H) RC of Ru-CoS2 of the corresponding samples; and (I) FT-IR spectra of the related samples. Meanwhile, 1, 2, 3, 4, 5, and 6 are MoS2-CoS2/CC, Ru-MoS2-x-CoS2/CC-1.0, the typical samples, Ru-MoS2-x-CoS2/CC-7.0, Ru-MoS2-x-CoS2/CC-10.0, and Ru-MoS2-x-CoS2/CC-30.0, respectively. Additionally, [1], [2], [3], [4], [5], and [6] are the corresponding samples synthesized at t = 6 h rather than 20 h. The insets of panels (A–F) are the low-magnified SEM images of the corresponding samples. The insets in red and blue solid lines of panel (I) are the high-magnified images of panel (I) indicated in red and blue solid frames, respectively. Scale bars of panels (A–F) and their insets are 2 and 5 μm.
Possible Formation Mechanism of S-Vacancy in Ru-MoS2-X-CoS2/CC Samples
Based on these results and discussion, a possible formation mechanism of S-vacancy of the basal planes in Ru-MoS2-x of Ru-MoS2-x-CoS2/CC samples can be proposed as follows: on the one side, S-vacancy is modulated into the basal planes of MoS2 due to the strong attractive interaction between Ru(Mo) and the adjacent S-vacancy. Furthermore, S-vacancy is exclusively stable around Ru(Mo). On the other hand, by compositing with CoS2, Schottky heterojunctions provide a feasible opportunity for the electron transfer from Ru-CoS2 to Ru-MoS2-x (Li et al., 2018) due to a high Fermi level of CoS2. This effect can promote S2- escaping from Ru-MoS2-x nanosheets to remain charge neutrality (Liu et al., 2017; Gan et al., 2018), indicating the formation of the positively charged defects, S-vacancy. Subsequently, we further characterize the atom ratios of Co to (Co + Mo) of the related samples by ICP-OES in the inset of Figure 3A. From this inset, the atom ratio of Co to (Co + Mo) of the typical samples is as high as about 16.1% at V increasing to 4.0 ml, thereby resulting in more Ru-CoS2, and rich heterogeneous interfaces between Ru-CoS2 and Ru-MoS2-x as well. More heterogeneous interfaces favor the electron injection from Ru-CoS2 to Ru-MoS2-x, which leads to an increase in CS-vacancy (Figure 3E). However, further increasing V results in a decrease in atom ratios of Co to (Co + Mo) (inset of Figure 3A) and poor heterojunctions. This could indicate a weaker electron injection effect of Ru-CoS2 and lower CS-vacancy at excessively high V. The schematic illustration of the possible formation mechanism of S-vacancy of the basal planes in Ru-MoS2-x of Ru-MoS2-x-CoS2/CC samples is presented in Figure 5.
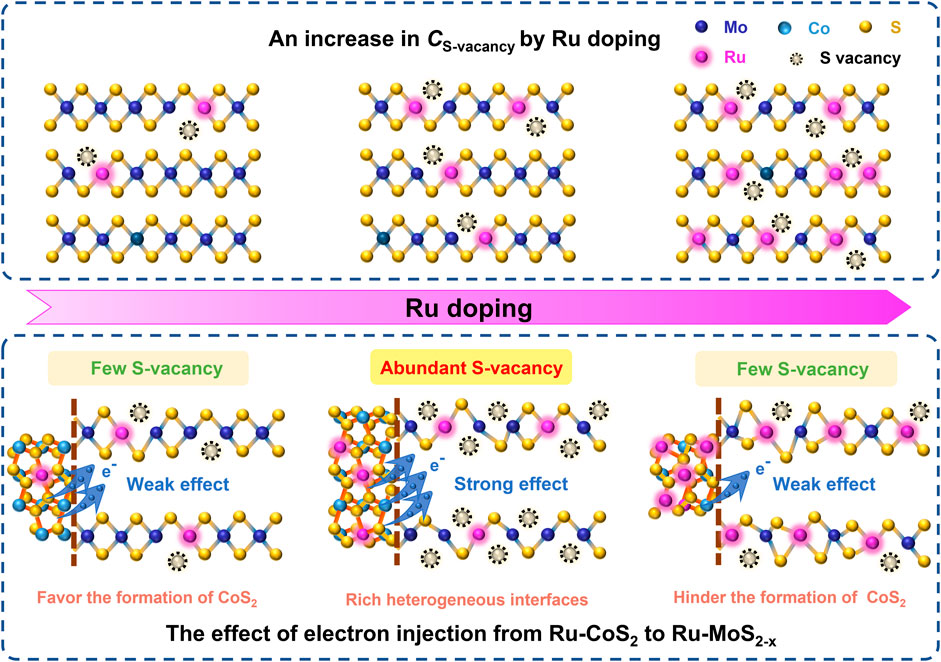
FIGURE 5. Schematic illustration of the possible formation mechanism of the S-vacancy of the basal planes in Ru-MoS2-x of Ru-MoS2-x-CoS2/CC samples.
To confirm this viewpoint, we systematically investigate the effect of compositing with CoS2 on CS-vacancy of the as-synthesized samples at fixed V or Ru dopants in Figure 6. Figure 6A exhibits XRD patterns of Ru-MoS2-x-CoS2/CC-80, Ru-MoS2-x-CoS2/CC -240, Ru-MoS2-x-CoS2/CC-280, and Ru-MoS2-x-CoS2/CC-320. With an increase in the mass of Co precursor (mCo), the intensity of the diffraction peak of the (002) plane at 14.1° for Ru-MoS2-x greatly decreases, while intensities of peaks of the (200) and 311) planes for Ru-CoS2 gradually increase (Figure 6A), indicating a decrease in the RC of Ru-MoS2-x and an increase in the RC of Ru-CoS2 (Supplementary Figure S10). At mCo increasing to 240 mg, Ru-CoS2 can be densely coated by Ru-MoS2-x nanosheets (Supplementary Figure S11B). Nevertheless, further increasing mCo to 280 or 320 mg leads to incompact Ru-MoS2-x shells (Supplementary Figures S11C, D) due to the formation of more Ru-CoS2 or a decrease in the content of Ru-MoS2-x. These data are in good agreement with the results of Figure 6D, which displays atom ratios of Co to (Co + Mo) of the as-synthesized samples by XPS analysis. These atom ratios also increase with increasing mCo. For example, the atom ratio of Co to (Co + Mo) of R Ru-MoS2-x-CoS2/CC-320 increases up to 10.1%. To further confirm the effect of electron injection from Ru-CoS2 to Ru-MoS2-x, we characterize Mo3d high-resolution XPS spectra of Ru-MoS2-x-CoS2/CC-80, Ru-MoS2-x-CoS2/CC-240, Ru-MoS2-x-CoS2/CC-280, and Ru-MoS2-x-CoS2/CC-320 in Figure 6B. Mo 3 days in XPS data move to low binding energy (Huang et al., 2019), implying an increase in the electron density of Ru-MoS2-x of the as-synthesized samples. For example, Mo 3 day peaks of the typical samples negatively shift by about 0.06 eV compared to those of Ru-MoS2-x-CoS2/CC-80 in terms of Figure 2D and Figure 6B. After careful investigations, similar results are observed in Mo3d XPS spectra of other Ru-MoS2-x-CoS2/CC samples (Figure 6B), yielding the related data about negative shifts of 0.12 eV for Ru-MoS2-x-CoS2/CC-240, 0.45 eV for Ru-MoS2-x-CoS2/CC-280, and 0.50 eV for Ru-MoS2-x-CoS2/CC-320. It is seen that such an electron injection effect increases gradually with the increase in mCo, which means more S2- escaping from MoS2-x nanosheets (Liu et al., 2017; Gan et al., 2018) at higher mCo. Therefore, the atom ratios of S to Mo for all Ru-MoS2-x-CoS2/CC samples decrease (Supplementary Table S3), and CS-vacancy increases (Figure 6E) with increasing mCo. For instance, Ru-MoS2-x-CoS2/CC-320 demonstrate the highest CS-vacancy (about 27.5%) among all samples.
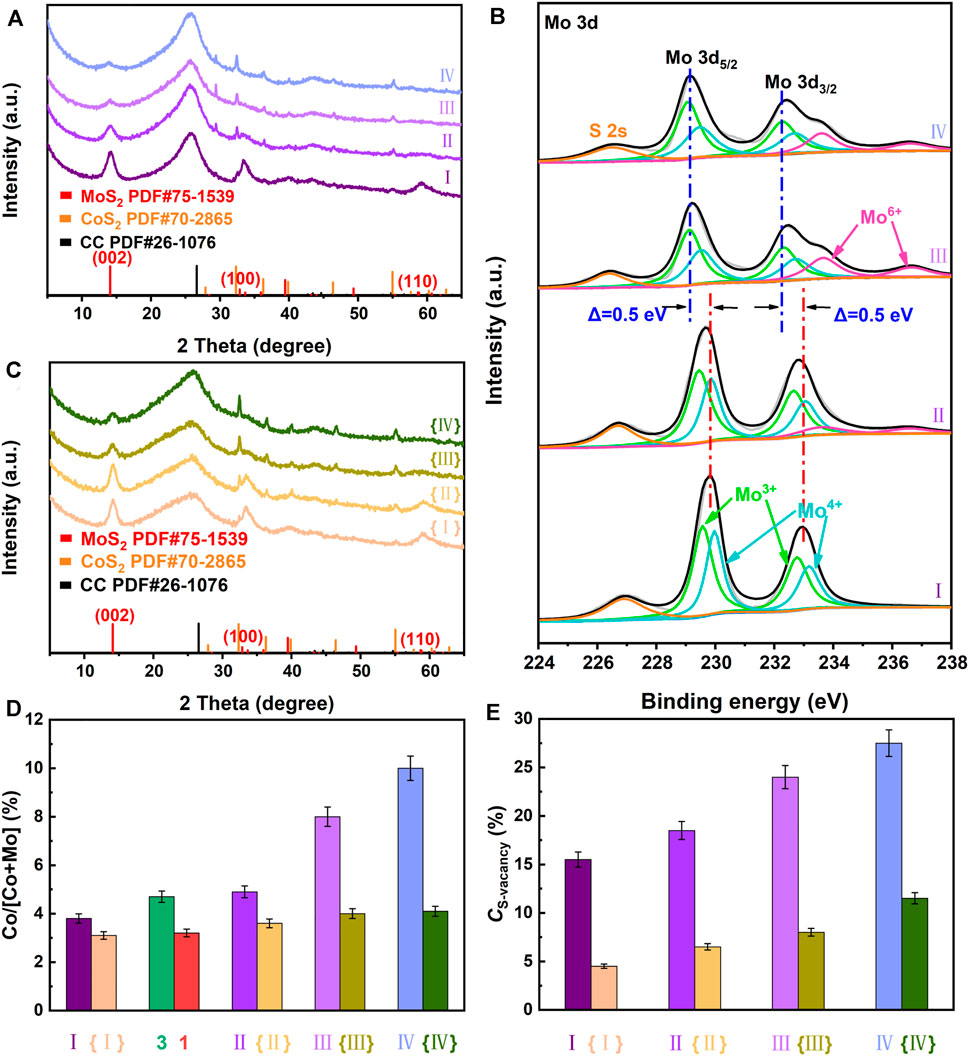
FIGURE 6. (A) XRD patterns and (B) Mo3d high-resolution XPS spectra of Ru-MoS2-x-CoS2/CC-80 (Ⅰ), Ru-MoS2-x-CoS2/CC-240 (Ⅱ), Ru-MoS2-x-CoS2/CC-280 (Ⅲ), and Ru-MoS2-x-CoS2/CC-320 (Ⅳ); (C) XRD patterns of MoS2-CoS2/CC-80 ({Ⅰ}), MoS2-CoS2/CC-240 ({Ⅱ}), MoS2-CoS2/CC-280 ({Ⅲ}), and MoS2-CoS2/CC-320 ({Ⅳ}); (D) atom ratios of Co to (Co + Mo) by XPS data, (E) CS-vacancy of the related samples. Meanwhile, fabrications of MoS2-CoS2/CC-80, MoS2-CoS2/CC-240, MoS2-CoS2/CC-280, and MoS2-CoS2/CC-320 are almost the same as those of Ru-MoS2-x-CoS2/CC-80, Ru-MoS2-x-CoS2/CC-240, Ru-MoS2-x-CoS2/CC-280, and Ru-MoS2-x-CoS2/CC-320 except for the absence of RuCl3 solution.
Additionally, Figure 6 also demonstrates the influence of compositing with CoS2 on CS-vacancy of the as-synthesized samples without Ru dopants. Figure 6C shows XRD patterns of MoS2-CoS2/CC-80, MoS2-CoS2/CC-240, MoS2-CoS2/CC-280, and MoS2-CoS2/CC-320. The influences of mCo on intensities of the related peaks and RC of MoS2 and CoS2 are shown in Figure 6C and Supplementary Figure S12, suggesting a decline in the crystallinity of MoS2 and an increase in crystallinity of CoS2 as well. The trend in the variation of atom ratios of Co to (Co + Mo) of the as-synthesized samples without Ru dopants is the same as that of Ru-doped samples in Figure 6D, which implies the formation of more CoS2 and richer heterojunctions at higher mCo. Similarly, the more heterojunctions are, the stronger electron injection effect from CoS2 to MoS2-x is obtained. For instance, Mo 3 day peaks of MoS2-CoS2/CC-240 display a negative shift of 0.15 eV in comparison with those of MoS2-CoS2/CC-80 from Supplementary Figure S13. At mCo increasing to 320 mg, Mo 3 day peaks of MoS2-CoS2/CC-320 present a negative shift of about 0.36 eV compared to those of MoS2-CoS2/CC-80 (Supplementary Figure S13). Therefore, the atom ratios of S to Mo for these samples without Ru dopants also decrease with increasing mCo (Supplementary Table S3), leading to an increase in CS-vacancy (Figure 6E) as well. Therefore, CS-vacancy of the basal planes of Ru-MoS2-x can be efficiently regulated by simply changing mCo based on the effect of electron injection from Ru-CoS2 to Ru-MoS2-x.
Together, either doping Ru or compositing with CoS2 only regulates CS-vacancy of the as-synthesized samples within a narrow range, such as between 2.1 and 10.5% for doping Ru (Figure 3E) and 4.5 and 11.5% for compositing with CoS2 (Figure 6E). Interestingly, synergistically regulating CS-vacancy of the as-synthesized samples, from 2.1 to 27.5% (Figure 6E), is realized by a new one-step doping-assisted compositing strategy.
HER Activities of Ru-MoS2-X-CoS2/CC Samples Synthesized at Various V
To find out the relationship between the microstructure and HER activity, we carefully investigate a series of electrochemical performances of Ru-MoS2-x-CoS2/CC samples synthesized at various V under the fixed mCo (160 mg) in Figures 7, 8. According to Figures 7A–D, the typical samples exhibit the highest HER activity among these samples, such as an overpotential of about 170 mV at a current density of 100 mA cm−2 and a Tafel plot of 71 mV dec−1 in alkaline environments (1 M KOH), implying that low or excessive Ru dopant is disadvantageous for the HER activity of Ru-MoS2-x-CoS2/CC samples.
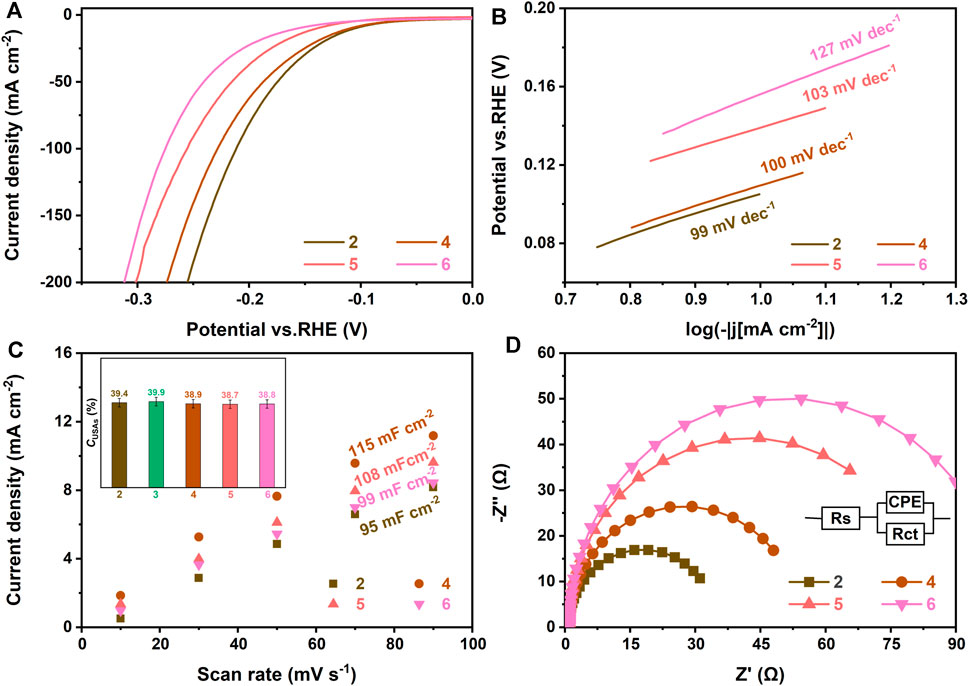
FIGURE 7. (A) Polarization curves, (B) Tafel plots, (C) Cdl at different scan rates, and (D) Nyquist plots of Ru-MoS2-x-CoS2/CC-1.0 (2), Ru-MoS2-x-CoS2/CC-7.0 (4), Ru-MoS2-x-CoS2/CC-10.0 (5), and Ru-MoS2-x-CoS2/CC-30.0 (6). The inset of panel (C) is CUSAs of the related samples. The electrolyte is N2-saturated 1 M KOH.
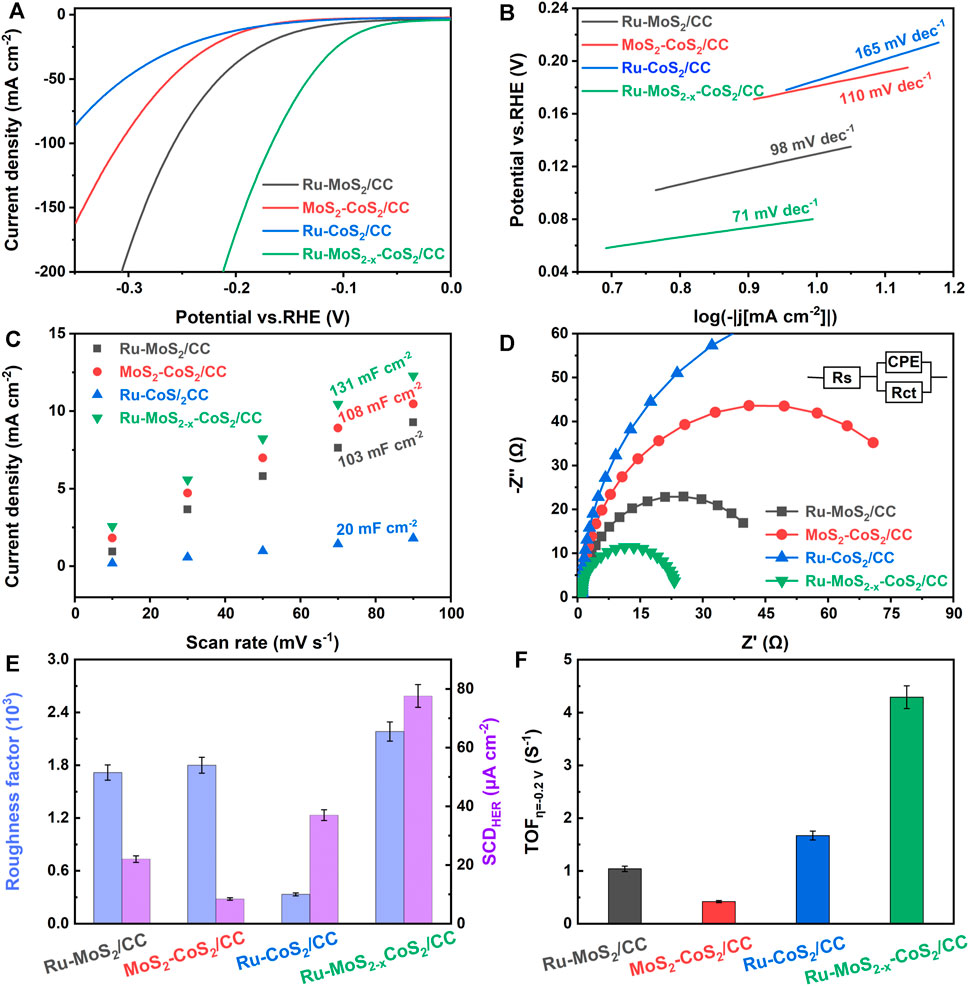
FIGURE 8. (A) Polarization curves, (B) Tafel plots, (C) Cdl at different scan rates, (D) Nyquist plots, (E) Rf and SCDHER, and (F) TOF at 0.2 V of Ru-MoS2/CC, MoS2-CoS2/CC, Ru-CoS2/CC, and the typical samles. The electrolyte is N2-saturated 1 M KOH.
Figure 7C displays double-layer capacitance (Cdl) to evaluate electrochemical active surface area (ECSA), which is calculated by cyclic voltammetry (CV) curves in Supplementary S14, 15. From Figure 7C and Figure 8C, the typical samples also demonstrate the highest Cdl among all Ru-MoS2-x-CoS2/CC samples, reaching up to ca. 131 mF cm−2. For 2H-MoS2, its active sites are mainly from unsaturated sulfur atoms (Kibsgaard et al., 2012; Xie et al., 2013a; Liu et al., 2018). This is because the adsorption free energy of hydrogen atom (ΔGH) of unsaturated sulfur atoms approaches zero (about -0.06 eV), which indicates their excellent H* adsorption–desorption property (Hinnemann et al., 2005). More recently, modulating sulfur vacancy into MoS2-based materials has been developed as an efficient strategy (Tsai et al., 2017; Park et al., 2018; Li Y. et al., 2021) to activate inert basal planes because EMAs can be tailored into newborn active sites (Wang X. et al., 2020). Therefore, the active sites of these Ru-MoS2-x-CoS2/CC samples should include unsaturated sulfur atoms and EMAs.
To confirm the difference in active sites for such Ru-MoS2-x-CoS2/CC samples, the inset of Figure 7C further shows the concentration of unsaturated sulfur atoms (USAs) of all samples based on XPS data from Supplementary Figure S16. From this inset, there is no significant difference in the concentration of USAs (CUSAs) for these samples. This may be closely related to preferentially exposed sulfur-edge atoms of Ru-MoS2-x nanosheet array vertically assembled on CC. At the same time, this inset implies that USAs are not the essential factor for the most abundant active sites of the typical samples. From Figure 3E, CS-vacancy for Ru-MoS2-x-CoS2/CC-1.0, the typical samples, Ru-MoS2-x-CoS2/CC-7.0, Ru-MoS2-x-CoS2/CC-10.0, and Ru-MoS2-x-CoS2/CC-30.0 are 7.9, 17.1, 14.0, 12.5, and 12.0%, respectively. It is seen that CS-vacancy of the typical samples is higher than that of other Ru-MoS2-x-CoS2/CC samples. Consequently, we can reasonably conclude that the difference in active sites of RCM/CC should be dependent on CS-vacancy. The formation of one S-vacancy means the occurrence of three EMAs at CS-vacancy < 18% (Li et al., 2016a) because S-vacancy uniformly distributes on the basal planes of 2H-MoS2-x. Obviously, under this situation, the higher CS-vacancy is obtained, the more EMAs or active sites are achieved. At mCo = 160 mg, Ru doping firstly favors heterogeneous nucleation and growth of CoS2 (Figures 4C, I) besides introducing a certain quantity S-vacancy into the basal planes. For instance, at V increasing to 4.0 ml, the typical samples demonstrate higher CS-vacancy (Figure 3E) than Ru-MoS2-x-CoS2/CC-1.0, maybe due to more heterogeneous interfaces between Ru-CoS2 and Ru-MoS2-x. Nevertheless, further increasing V hinders the formation of Ru-CoS2 (Figures 4F, I) and leads to a decline in CS-vacancy. Lower CS-vacancy could originate from fewer Schottky heterojunctions and a weaker electron injection effect of Ru-CoS2 at excessively high V.
As another key factor for HER, the RCT of all Ru-MoS2-x-CoS2/CC samples is investigated in Figure 7D and Figure 8D. These figures exhibit their Nyquist plots. Each semicircle represents the RCT of the cathode reaction. The charge transfer of Ru-MoS2-x-CoS2/CC-1.0 or Ru-MoS2-x-CoS2/CC-7.0 is inferior to that of the typical samples. Moreover, further increasing V results in considerably unsatisfactory RCT from Figure 7D. In our viewpoint, rich active sites will promote the occurrence of HER reaction in the cathode, implying efficient charge transfer for the typical samples. On the other hand, compositing with the metallic phase for fast electrode kinetics (He et al., 2020; Li et al., 2020; Zhou G. et al., 2021), such as CoS2 (Li et al., 2020), carbon nanotube (Huang et al., 2017), and reduced graphene oxide (Wang Y. et al., 2020), is one of the important approaches to improving electrocatalytic HER activity. From Figure 7D and Figure 8D, Ru-MoS2-x-CoS2/CC-1.0 demonstrate better charge transfer compared to Ru-MoS2/CC. Furthermore, the influence of V on RCT is coincident with the trend in the variation of RC of Ru-CoS2 (Figure 3C) and atom ratios of Co to (Co + Mo) (inset of Figure 3A) of all Ru-MoS2-x-CoS2/CC samples. Therefore, the higher crystallinity of cobalt disulfide or the more CoS2 is obtained, the lower RCT of the as-synthesized samples is achieved.
HER Activities of Ru-MoS2-X-CoS2/CC Samples Synthesized at Various mCo
We further investigate the HER activity of Ru-MoS2-x-CoS2/CC samples synthesized at various mCo under the fixed V (4.0 ml) in Supplementary Figure S17. From Supplementary Figures S17A, B, HER activities of Ru-MoS2-x-CoS2/CC-80 are an overpotential of about 228 mV at a current density of 100 mA cm−2 and a Tafel plot of 102 mV dec−1. At mCo increasing to 160 mg, the typical samples demonstrate higher HER activity than other samples (Figure 8A; Supplementary Figure S17A). Further increasing mCo leads to unsatisfactory overpotential and sluggish electrode kinetics, yielding the related data of 264 and 123 mV dec−1 for Ru-MoS2-x-CoS2/CC-240, 294 and 130 mV dec−1 for Ru-MoS2-x-CoS2/CC-280, and 301 and 140 mV dec−1 for Ru-MoS2-x-CoS2/CC-320 at the same current density.
To illustrate the reason why excessive mCo is disadvantageous for HER activity, Cdl and CUSAs of all samples are tested in Supplementary FIgure S17C and Supplementary Figure S18, respectively. The corresponding CV curves related to Supplementary FIgure S17C are shown in Supplementary Figure S19. From Supplementary FIgure S17C, Cdl gradually increases with an increase in mCo. For example, Ru-MoS2-x-CoS2/CC-80 display low Cdl (about 118 mF cm−2) at mCo = 80 mg. At mCo increasing to 320 mg, the Cdl of Ru-MoS2-x-CoS2/CC-320 is as high as 199 mF cm−2. We rationally hypothesize that this is closely related to CS-vacancy, due to the fact that high CS-vacancy favors abundant EMAs. As we know, the basal planes in 2H-MoS2 are inert, owing to ΔGH reaching 2.1 eV (Zhou W. et al., 2021). Impressively, inert basal planes can be efficiently activated by a low concentration of S-vacancy (ca 4%) modulating into them because the corresponding ΔGH closes to zero (Wang X. et al., 2020). It is not difficult to understand that such EMAs should be highly active, but the number is considerably poor.
According to the related literature (Wang X. et al., 2020), ΔGH negatively shifts with an increase in CS-vacancy, implying that hydrogen atoms do not easily desorb at excessive CS-vacancy. For instance, at excessive mCo, CS-vacancy of Ru-MoS2-x-CoS2/CC-320 reaches up to 27.5%. As expected, EMAs are abundant in this case, but the catalytic sites are of low activity, owing to the undesirable ΔGH (Wang X. et al., 2020). Subsequently, a balance between the intrinsic activity and the number of EMAs to boost highly active EMAs could be realized by precisely regulating CS-vacancy to 17.1% (Wang X. et al., 2020). Consequently, the typical samples demonstrate the optimal HER activity among all samples. Furthermore, CUSAs of all samples except for Ru-MoS2-x-CoS2/CC-320 is almost the same as that of the typical samples. CUSAs of Ru-MoS2-x-CoS2/CC-320 (about 33.9%) is lower than that of other samples, which is responsible for the incompact Ru-MoS2-x shells (Supplementary Figure S11D), and the significant decrease in the content of molybdenum disulfide (Figure 6D). This indicates that sulfur-edge atoms of Ru-MoS2-x nanosheets are not easy to be preferentially exposed for such samples synthesized at excessive mCo.
Last but not least, Supplementary Figure S17Dand Figure 8D further exhibit RCT of all samples. Higher mCo leads to higher RCT of the related samples (Supplementary Figure S17D). For example, at mCo reaching up to 320 mg, Ru-MoS2-x-CoS2/CC-320 display the highest RCT among all Ru-MoS2-x-CoS2/CC samples. It can be ascribed to insufficient highly active EMAs regardless of the higher RC of Ru-CoS2 (Figure 6A; Supplementary Figure S10) for these samples synthesized at higher mCo. Insufficient active sites could not promote redox half-reaction, thereby leading to inefficient charge transfer.
Comparison of HER Activities of the Typical Samples and Other Similar Electrocatalysts
HER activities of Ru-MoS2/CC, MoS2-CoS2/CC, Ru-CoS2/CC, and the typical samples are shown in Figure 8A. Ru-CoS2/CC display the lowest HER activity among these samples, suggesting that the activity of the typical samples is mainly from Ru-MoS2-x rather than Ru-CoS2. In addition, Ru-MoS2/CC exhibit faster electrode kinetics than MoS2-CoS2/CC according to Figure 8B. In our viewpoint, the Ru-doped sites can significantly accelerate the sluggish water dissociation in alkaline HER (Li J. et al., 2021).
From Figure 8C, Cdl of Ru-MoS2/CC is only 103 mF cm−2 and lower than that of the typical samples. Furthermore, CUSAs of Ru-MoS2/CC (about 39.3%) is almost the same as that of the typical samples from Supplementary Figure S18. Therefore, the difference in active sites between Ru-MoS2/CC and the typical samples should be closely related to CS-vacancy. Higher CS-vacancy indicates more highly active EMAs. As shown in Figure 3E, CS-vacancy of Ru-MoS2/CC is only about 3.1% by doping Ru, implying insufficient active sites. In our strategy, doping Ru coupled to compositing with CoS2 synergistically regulates CS-vacancy of the as-synthesized samples from 2.1 to 27.5%. For instance, CS-vacancy of the typical samples reaches up to 17.1% (Figure 3E), which implies that rich highly active sites are successfully introduced into the typical samples. In terms of Figure 8D, RCT of the typical samples is lower than that of Ru-MoS2/CC. Besides rich active sites promoting redox half-reaction, high crystallized Ru-CoS2 of the typical samples is another important factor to realize the efficient charge transfer during electrocatalysis. Next, the specific activity and turnover frequency (TOF) are further conducted to investigate the intrinsic activity of all samples (Figures 8E, F). Cdl of an ideal plane electrode is considered as 60 μF cm−2 (Levine and Smith, 1971); the roughness factor (Rf) can be calculated by the formula: Rf = (Cdl 60-1)×10+3. The specific alkaline HER current density (SCDHER) is determined by the formula: SCDHER = j Rf−1 (Jovic et al., 2015; Jovic et al., 2016), where j is the current density at an overpotential of -0.2 V. From Figure 8E, the typical samples have not only the largest Rf (2.18 × 10+3) but also the highest specific activity (about 77.6 μA cm−2) among all samples. In addition, the TOF for these samples is obtained by the formula TOF = jS (2nF) −1. Here, S is the geometrical surface area in cm2 and F is the Faraday constant. The value of n is the number of active sites (mol), which is confirmed according to the previously reported literature (Tian et al., 2014). The typical samples also exhibit the highest intrinsic activity among all samples, about 4.29 s−1 in Figure 8F. Moreover, the comparison between the typical samples and other similar electrocatalysts is provided in Table 1. The typical samples exhibit higher HER activity than other similar electrocatalysts listed in Table 1, which is ascribed to two factors: abundant active sites and accelerated electrode kinetics during the HER process.
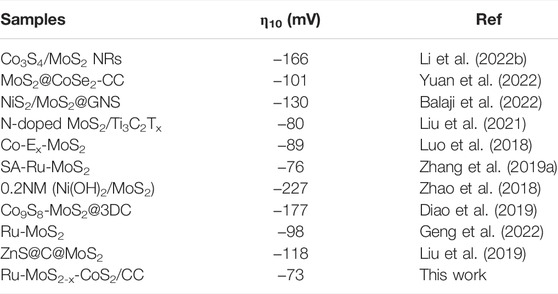
TABLE 1. Comparison of the HER electrocatalytic activity of Ru-MoS2-x-CoS2/CC with some MoS2-based HER electrocatalysts recently reported.
The Long-Term Durability of the Typical Samples
In addition, LSV curves of the typical samples before and after 1000 cycles are recorded to evaluate their durability, and just a 10 mV negative shift at a current density of -100 mA cm−2 is seen in Supplementary Figure S20A, B; the chronoamperometric response (i ∼ t) of the typical samples displays negligible attenuation of the current density for 10 h. Moreover, no significant change is observed in the morphology and phase structure after 1000 cycles (Supplementary Figures S21, 22). All results suggest that the typical samples possess remarkable long-term durability for HER in alkaline media.
Conclusion
Herein, we develop a one-step Ru doping coupled to compositing with the CoS2 strategy for the fabrication of Ru-MoS2-x-CoS2/CC. In our strategy, Ru doping is advantageous for the formation of S-vacancy in the basal planes of MoS2. More importantly, Ru doping affects microstructures of CoS2, which has a significant influence on CS-vacancy of Ru-MoS2-x nanosheets in Ru-MoS2-x-CoS2/CC samples in turn. At the fixed mCo (160 mg), Ru doping favors the heterogeneous nucleation and growth of CoS2 at V increasing to 4.0 ml, which leads to a high crystallinity of Ru-CoS2 and rich heterogeneous interfaces between Ru-CoS2 and Ru-MoS2-x. This facilitates the electron transfer from Ru-CoS2 to Ru-MoS2-x, indicating an increase in CS-vacancy, thereby increasing CS-vacancy of the MoS2-based materials. However, further increasing V results in a low crystallinity of Ru-CoS2 and poor heterojunctions, implying a weaker electron injection effect of Ru-CoS2 and low CS-vacancy. At fixed V (4.0 ml of RuCl3 solution), the electron injection effect increases gradually with the increase in mCo, which means more S2- escaping from Ru-MoS2 nanosheets at higher mCo. Therefore, synergistically regulating CS-vacancy of the as-synthesized samples, from 2.1 to 27.5%, is realized by a new one-step Ru doping coupled to compositing with the CoS2 strategy. High CS-vacancy indicates abundant EMAs. Impressively, inert basal planes can be efficiently activated by modulating low CS-vacancy into Ru-MoS2-x-CoS2/CC samples. However, the number is considerably poor. Additionally, hydrogen atoms do not easily desorb at excessive CS-vacancy. This is because EMAs are abundant in this case, but the catalytic sites are of low activity, owing to the undesirable ΔGH. By precisely regulating CS-vacancy to 17.1%, a balance between the intrinsic activity and the number of EMAs to boost highly active EMAs should be realized. Consequently, the typical samples demonstrate the optimal alkaline HER activity among all samples, such as a low overpotential of 170 mV at 100 mA cm−2, a large SCDHER of 77.6 μA cm−2, and a TOF of 4.29 s−1 at - 0.2 V as well as excellent long-term stability. The results pave a new approach to activating inert basal planes in MoS2 for efficient hydrogen evolution and promise important applications in the fields of electrocatalysis or energy conversion.
Data Availability Statement
The original contributions presented in the study are included in the article/Supplementary Material; further inquiries can be directed to the corresponding authors.
Author Contributions
X-YL has made substantial contributions to the design of this work and has drafted the work. S-JZ has made substantial contributions to the acquisition, analysis, and interpretation of data for the work. YW has revised the work critically for the important intellectual content and has approved the final version to be published. TL, X-YY, and C-FY have made some contributions to the analysis and interpretation of data for the work. YL has given some important suggestions for this revised article. BS has given some suggestions on preparing this article. L-HC has provided some important ideas for the design of this work and has approved the final version to be published.
Funding
This work was also financially supported by the National Natural Science Foundation of China (No. U20A20122).
Conflict of Interest
The authors declare that the research was conducted in the absence of any commercial or financial relationships that could be construed as a potential conflict of interest.
Publisher’s Note
All claims expressed in this article are solely those of the authors and do not necessarily represent those of their affiliated organizations, or those of the publisher, the editors, and the reviewers. Any product that may be evaluated in this article, or claim that may be made by its manufacturer, is not guaranteed or endorsed by the publisher.
Acknowledgments
L-HC acknowledges the Hubei Provincial Department of Education for the “Chutian Scholar” program. Y-LW acknowledges the Fundamental Research Funds for the Central Universities (WUT: 2020-IB-028). The authors deeply thank the 111 Projects (Grant No. B20002) and the International Science and Technology Cooperation Program of China (2021YFE0115800) for supporting this work.
Supplementary Material
The Supplementary Material for this article can be found online at: https://www.frontiersin.org/articles/10.3389/fchem.2022.915468/full#supplementary-material
References
Balaji, D., Madhavan, J., Vinesh, V., Neppolian, B., AlSalhi, M. S., and Prasad, S. (2022). Graphene Supported Flower-like NiS2/MoS2 Mixed Phase Nano-Composites as a Low Cost Electrode Material for Hydrogen Evolution Reaction in Alkaline Media. Mater. Chem. Phys. 280, 125839. doi:10.1016/j.matchemphys.2022.125839
Chakraborty, I., Malik, P. K., and Moulik, S. P. (2006). Preparation and Characterisation of CoS2 Nanomaterial in Aqueous Cationic Surfactant Medium of Cetyltrimethylammonium Bromide (CTAB). J. Nanopart. Res. 8 (6), 889–897. doi:10.1007/s11051-005-9032-y
Chen, T.-T., Wang, R., Li, L.-K., Li, Z.-J., and Zang, S.-Q. (2020). MOF-derived Co9S8/MoS2 Embedded in Tri-doped Carbon Hybrids for Efficient Electrocatalytic Hydrogen Evolution. J. Energy Chem. 44, 90–96. doi:10.1016/j.jechem.2019.09.018
Deng, J., Li, H., Wang, S., Ding, D., Chen, M., Liu, C., et al. (2017). Multiscale Structural and Electronic Control of Molybdenum Disulfide Foam for Highly Efficient Hydrogen Production. Nat. Commun. 8 (1), 14430. doi:10.1038/ncomms14430
Diao, L., Zhang, B., Sun, Q., Wang, N., Zhao, N., Shi, C., et al. (2019). An In-Plane Co9S8@MoS2 Heterostructure for the Hydrogen Evolution Reaction in Alkaline Media. Nanoscale 11 (44), 21479–21486. doi:10.1039/C9NR06609H
Djara, R., Holade, Y., Merzouki, A., Lacour, M.-A., Masquelez, N., Flaud, V., et al. (2020). Nanostructured Carbon-Nitrogen-Sulfur-Nickel Networks Derived from Polyaniline as Bifunctional Catalysts for Water Splitting. Front. Chem. 8, 22. doi:10.3389/fchem.2020.00385
Feng, J.-X., Wu, J.-Q., Tong, Y.-X., and Li, G.-R. (2018). Efficient Hydrogen Evolution on Cu Nanodots-Decorated Ni3S2 Nanotubes by Optimizing Atomic Hydrogen Adsorption and Desorption. J. Am. Chem. Soc. 140 (2), 610–617. doi:10.1021/jacs.7b08521
Gan, X., Lee, L. Y. S., Wong, K.-y., Lo, T. W., Ho, K. H., Lei, D. Y., et al. (2018). 2H/1T Phase Transition of Multilayer MoS2 by Electrochemical Incorporation of S Vacancies. ACS Appl. Energy Mat. 1 (9), 4754–4765. doi:10.1021/acsaem.8b00875
Gao, B., Du, X., Li, Y., Ding, S., Xiao, C., and Song, Z. (2020). Deep Phase Transition of MoS2 for Excellent Hydrogen Evolution Reaction by a Facile C-Doping Strategy. ACS Appl. Mat. Interfaces 12 (1), 877–885. doi:10.1021/acsami.9b18940
Ge, R., Wang, Y., Li, Z., Xu, M., Xu, S. M., Zhou, H., et al. (2022). Selective Electrooxidation of Biomass‐Derived Alcohols to Aldehydes in a Neutral Medium: Promoted Water Dissociation over a Nickel‐Oxide‐Supported Ruthenium Single‐Atom Catalyst. Angew. Chem. Int. Ed. 61. doi:10.1002/anie.202200211
Geng, S., Tian, F., Li, M., Liu, Y., Sheng, J., Yang, W., et al. (2022). Activating Interfacial S Sites of MoS2 Boosts Hydrogen Evolution Electrocatalysis. Nano Res. 15 (3), 1809–1816. doi:10.1007/s12274-021-3755-7
Gong, F., Ye, S., Liu, M., Zhang, J., Gong, L., Zeng, G., et al. (2020). Boosting Electrochemical Oxygen Evolution over Yolk-Shell Structured O-MoS2 Nanoreactors with Sulfur Vacancy and Decorated Pt Nanoparticles. Nano Energy 78, 105284. doi:10.1016/j.nanoen.2020.105284
Hao, J., Yang, W., Peng, Z., Zhang, C., Huang, Z., and Shi, W. (2017). A Nitrogen Doping Method for CoS2 Electrocatalysts with Enhanced Water Oxidation Performance. ACS Catal. 7 (6), 4214–4220. doi:10.1021/acscatal.7b00792
He, S., Du, H., Wang, K., Liu, Q., Sun, J., Liu, Y., et al. (2020). Low-temperature Molten Salt Synthesis of MoS2@CoS2 Heterostructures for Efficient Hydrogen Evolution Reaction. Chem. Commun. 56 (41), 5548–5551. doi:10.1039/D0CC01726D
Hinnemann, B., Moses, P. G., Bonde, J., Jørgensen, K. P., Nielsen, J. H., Horch, S., et al. (2005). Biomimetic Hydrogen Evolution: MoS2 Nanoparticles as Catalyst for Hydrogen Evolution. J. Am. Chem. Soc. 127 (15), 5308–5309. doi:10.1021/ja0504690
Huang, H., Huang, W., Yang, Z., Huang, J., Lin, J., Liu, W., et al. (2017). Strongly Coupled MoS2 Nanoflake-Carbon Nanotube Nanocomposite as an Excellent Electrocatalyst for Hydrogen Evolution Reaction. J. Mat. Chem. A 5 (4), 1558–1566. doi:10.1039/C6TA09612C
Huang, J., Hou, D., Zhou, Y., Zhou, W., Li, G., Tang, Z., et al. (2015). MoS2 Nanosheet-Coated CoS2 Nanowire Arrays on Carbon Cloth as Three-Dimensional Electrodes for Efficient Electrocatalytic Hydrogen Evolution. J. Mat. Chem. A 3 (45), 22886–22891. doi:10.1039/C5TA07234D
Huang, N., Ding, Y., Yan, S., Yang, L., Sun, P., Huang, C., et al. (2019). Ultrathin MoS2 Nanosheets Vertically Grown on CoS2 Acicular Nanorod Arrays: A Synergistic Three-Dimensional Shell/Core Heterostructure for High-Efficiency Hydrogen Evolution at Full pH. ACS Appl. Energy Mat. 2 (9), 6751–6760. doi:10.1021/acsaem.9b01219
Jiang, J., Cong, H., Huang, X., Sun, R., Li, Y., Xu, W., et al. (2022). Three-dimensional ZnCo/MoS2-Co3S4/NF Heterostructure Supported on Nickel Foam as Highly Efficient Catalyst for Hydrogen Evolution Reaction. Int. J. Hydrogen Energy 47 (5), 2947–2957. doi:10.1016/j.ijhydene.2021.10.217
Jing, Y., Lei, Q., Hu, G., He, J., Lei, X., Wang, F., et al. (2020). PVP/ZIF-8-derived Zn, Ni Co-loaded N-Doped Porous Carbon as a Catalyst for an Efficient Hydrogen Evolution Reaction. Front. Chem. 8, 6. doi:10.3389/fchem.2020.00723
Jović, B. M., Jović, V. D., Lačnjevac, U. Č., Gajić-Krstajić, L., and Krstajić, N. V. (2015). Ni-(Ebonex-supported Ir) Composite Coatings as Electrocatalysts for Alkaline Water Electrolysis. Part I: Hydrogen Evolution. Int. J. Hydrogen Energy 40 (33), 10480–10490. doi:10.1016/j.ijhydene.2015.06.127
Jović, B. M., Jović, V. D., Lačnjevac, U. Č., Stevanović, S. I., Kovač, J., Radović, M., et al. (2016). Ru Layers Electrodeposited onto Highly Stable Ti2AlC Substrates as Cathodes for Hydrogen Evolution in Sulfuric Acid Solutions. J. Electroanal. Chem. 766, 78–86. doi:10.1016/j.jelechem.2016.01.038
Kang, Y. (2021). Interplay between Transition-Metal Dopants and Sulfur Vacancies in MoS2 Electrocatalyst. Surf. Sci. 704, 121759. doi:10.1016/j.susc.2020.121759
Kibsgaard, J., Chen, Z., Reinecke, B. N., and Jaramillo, T. F. (2012). Engineering the Surface Structure of MoS2 to Preferentially Expose Active Edge Sites for Electrocatalysis. Nat. Mater 11 (11), 963–969. doi:10.1038/nmat3439
Levine, S., and Smith, A. L. (1971). Theory of the Differential Capacity of the Oxide/aqueous Electrolyte Interface. Discuss. Faraday Soc. 52 (0), 290–301. doi:10.1039/DF9715200290
Li, C., Liu, Y., Zhuo, Z., Ju, H., Li, D., Guo, Y., et al. (2018). Local Charge Distribution Engineered by Schottky Heterojunctions toward Urea Electrolysis. Adv. Energy Mat. 8 (27), 1801775. doi:10.1002/aenm.201801775
Li, H., Tsai, C., Koh, A. L., Cai, L., Contryman, A. W., Fragapane, A. H., et al. (2016a). Activating and Optimizing MoS2 Basal Planes for Hydrogen Evolution through the Formation of Strained Sulphur Vacancies. Nat. Mater 15 (1), 48–53. doi:10.1038/nmat4465
Li, H., Tsai, C., Koh, A. L., Cai, L., Contryman, A. W., Fragapane, A. H., et al. (2016b). Erratum: Corrigendum: Activating and Optimizing MoS2 Basal Planes for Hydrogen Evolution through the Formation of Strained Sulphur Vacancies. Nat. Mater 15 (3), 364. doi:10.1038/nmat4564
Li, J., Li, Y., Wang, J., Zhang, C., Ma, H., Zhu, C., et al. (2022a). Elucidating the Critical Role of Ruthenium Single Atom Sites in Water Dissociation and Dehydrogenation Behaviors for Robust Hydrazine Oxidation‐Boosted Alkaline Hydrogen Evolution. Adv. Funct. Mater. 32, 2109439. doi:10.1002/adfm.202109439
Li, J., Zhang, C., Ma, H., Wang, T., Guo, Z., Yang, Y., et al. (2021a). Modulating Interfacial Charge Distribution of Single Atoms Confined in Molybdenum Phosphosulfide Heterostructures for High Efficiency Hydrogen Evolution. Chem. Eng. J. 414, 128834. doi:10.1016/j.cej.2021.128834
Li, L., Qin, Z., Ries, L., Hong, S., Michel, T., Yang, J., et al. (2019). Role of Sulfur Vacancies and Undercoordinated Mo Regions in MoS2 Nanosheets toward the Evolution of Hydrogen. ACS Nano 13 (6), 6824–6834. doi:10.1021/acsnano.9b01583
Li, S., Sirisomboonchai, S., An, X., Ma, X., Li, P., Ling, L., et al. (2020). Engineering Interfacial Structures to Accelerate Hydrogen Evolution Efficiency of MoS2 over a Wide pH Range. Nanoscale 12 (12), 6810–6820. doi:10.1039/D0NR00008F
Li, Y., Wang, H., Xie, L., Liang, Y., Hong, G., and Dai, H. (2011). MoS2 Nanoparticles Grown on Graphene: An Advanced Catalyst for the Hydrogen Evolution Reaction. J. Am. Chem. Soc. 133 (19), 7296–7299. doi:10.1021/ja201269b
Li, Y., Zuo, S., Li, Q.-H., Wu, X., Zhang, J., Zhang, H., et al. (2021b). Vertically Aligned MoS2 with In-Plane Selectively Cleaved Mo-S Bond for Hydrogen Production. Nano Lett. 21 (4), 1848–1855. doi:10.1021/acs.nanolett.0c04978
Li, Z., Xu, W., Yu, X., Yang, S., Zhou, Y., Zhou, K., et al. (2022b). Synergistic Effect between 1D Co3S4/MoS2 Heterostructures to Boost the Performance for Alkaline Overall Water Splitting. Inorg. Chem. Front. 9, 2139–2149. doi:10.1039/D1QI01646F
Lin, H., Li, H., Li, Y., Liu, J., Wang, X., and Wang, L. (2017). Hierarchical CoS/MoS2 and Co3S4/MoS2/Ni2P Nanotubes for Efficient Electrocatalytic Hydrogen Evolution in Alkaline Media. J. Mat. Chem. A 5 (48), 25410–25419. doi:10.1039/C7TA08760H
Lin, J., Wang, P., Wang, H., Li, C., Si, X., Qi, J., et al. (2019). Defect‐Rich Heterogeneous MoS 2/NiS 2 Nanosheets Electrocatalysts for Efficient Overall Water Splitting. Adv. Sci. 6 (14), 1900246. doi:10.1002/advs.201900246
Liu, D., Lv, Z., Dang, J., Ma, W., Jian, K., Wang, M., et al. (2021). Nitrogen-Doped MoS2/Ti3C2TX Heterostructures as Ultra-efficient Alkaline HER Electrocatalysts. Inorg. Chem. 60 (13), 9932–9940. doi:10.1021/acs.inorgchem.1c01193
Liu, G., Robertson, A. W., Li, M. M.-J., Kuo, W. C. H., Darby, M. T., Muhieddine, M. H., et al. (2017). MoS2 Monolayer Catalyst Doped with Isolated Co Atoms for the Hydrodeoxygenation Reaction. Nat. Chem. 9 (8), 810–816. doi:10.1038/nchem.2740
Liu, L., Wang, Y., Zhao, Y., Wang, Y., Zhang, Z., Wu, T., et al. (2022a). Ultrahigh Pt‐Mass‐Activity Hydrogen Evolution Catalyst Electrodeposited from Bulk Pt. Adv. Funct. Mater. 32 (20), 2112207. doi:10.1002/adfm.202112207
Liu, S., Li, S., Sekar, K., Li, R., Zhu, Y., Xing, R., et al. (2019). Hierarchical ZnS@C@MoS2 Core-Shell Nanostructures as Efficient Hydrogen Evolution Electrocatalyst for Alkaline Water Electrolysis. Int. J. Hydrogen Energy 44 (47), 25310–25318. doi:10.1016/j.ijhydene.2019.08.048
Liu, W., Wang, X., Yu, H., and Yu, J. (2018). Direct Photoinduced Synthesis of Amorphous CoMoSx Cocatalyst and Its Improved Photocatalytic H2-Evolution Activity of CdS. ACS Sustain. Chem. Eng. 6 (9), 12436–12445. doi:10.1021/acssuschemeng.8b02971
Liu, X., Yin, Z., Cui, M., Gao, L., Liu, A., Su, W.-N., et al. (2022b). Double Shelled Hollow CoS2@MoS2@NiS2 Polyhedron as Advanced Trifunctional Electrocatalyst for Zinc-Air Battery and Self-Powered Overall Water Splitting. J. Colloid Interface Sci. 610, 653–662. doi:10.1016/j.jcis.2021.11.115
Luo, Y., Li, X., Cai, X., Zou, X., Kang, F., Cheng, H.-M., et al. (2018). Two-Dimensional MoS2 Confined Co(OH)2 Electrocatalysts for Hydrogen Evolution in Alkaline Electrolytes. ACS Nano 12 (5), 4565–4573. doi:10.1021/acsnano.8b00942
Luo, Z., Zhang, H., Yang, Y., Wang, X., Li, Y., Jin, Z., et al. (2020). Reactant Friendly Hydrogen Evolution Interface Based on Di-anionic MoS2 Surface. Nat. Commun. 11 (1), 1116. doi:10.1038/s41467-020-14980-z
Ma, Y., Hai, G., Atinafu, D. G., Dong, W., Li, R., Hou, C., et al. (2020). Carbon Inserted Defect-Rich MoS2−X nanosheets@CdSnanospheres for Efficient Photocatalytic Hydrogen Evolution under Visible Light Irradiation. J. Colloid Interface Sci. 569, 89–100. doi:10.1016/j.jcis.2020.02.071
Nguyen, D. C., Luyen Doan, T. L., Prabhakaran, S., Tran, D. T., Kim, D. H., Lee, J. H., et al. (2021). Hierarchical Co and Nb Dual-Doped MoS2 Nanosheets Shelled Micro-TiO2 Hollow Spheres as Effective Multifunctional Electrocatalysts for HER, OER, and ORR. Nano Energy 82, 105750. doi:10.1016/j.nanoen.2021.105750
Park, S., Park, J., Abroshan, H., Zhang, L., Kim, J. K., Zhang, J., et al. (2018). Enhancing Catalytic Activity of MoS2 Basal Plane S-Vacancy by Co Cluster Addition. ACS Energy Lett. 3 (11), 2685–2693. doi:10.1021/acsenergylett.8b01567
Qi, K., Cui, X., Gu, L., Yu, S., Fan, X., Luo, M., et al. (2019). Single-atom Cobalt Array Bound to Distorted 1T MoS2 with Ensemble Effect for Hydrogen Evolution Catalysis. Nat. Commun. 10 (1), 5231. doi:10.1038/s41467-019-12997-7
Smyth, D. M. (2000). The Effects of Dopants on the Properties of Metal Oxides. Solid State Ion. 129 (1), 5–12. doi:10.1016/S0167-2738(99)00312-4
Tian, J., Liu, Q., Asiri, A. M., and Sun, X. (2014). Self-Supported Nanoporous Cobalt Phosphide Nanowire Arrays: An Efficient 3D Hydrogen-Evolving Cathode over the Wide Range of pH 0-14. J. Am. Chem. Soc. 136 (21), 7587–7590. doi:10.1021/ja503372r
Tsai, C., Li, H., Park, S., Park, J., Han, H. S., Nørskov, J. K., et al. (2017). Electrochemical Generation of Sulfur Vacancies in the Basal Plane of MoS2 for Hydrogen Evolution. Nat. Commun. 8 (1), 15113. doi:10.1038/ncomms15113
Venkateshwaran, S., and Senthil Kumar, S. M. (2019). Template-Driven Phase Selective Formation of Metallic 1T-MoS2 Nanoflowers for Hydrogen Evolution Reaction. ACS Sustain. Chem. Eng. 7 (2), 2008–2017. doi:10.1021/acssuschemeng.8b04335
Wang, D., Li, Q., Han, C., Xing, Z., and Yang, X. (2019a). Single-atom Ruthenium Based Catalyst for Enhanced Hydrogen Evolution. Appl. Catal. B Environ. 249, 91–97. doi:10.1016/j.apcatb.2019.02.059
Wang, F., Yu, H., Feng, T., Zhao, D., Piao, J., and Lei, J. (2020a). Surface Roughed and Pt-Rich Bimetallic Electrocatalysts for Hydrogen Evolution Reaction. Front. Chem. 8, 9. doi:10.3389/fchem.2020.00422
Wang, H., Tsai, C., Kong, D., Chan, K., Abild-Pedersen, F., Nørskov, J. K., et al. (2015). Transition-metal Doped Edge Sites in Vertically Aligned MoS2 Catalysts for Enhanced Hydrogen Evolution. Nano Res. 8 (2), 566–575. doi:10.1007/s12274-014-0677-7
Wang, J., Bo, T., Shao, B., Zhang, Y., Jia, L., Tan, X., et al. (2021a). Effect of S Vacancy in Cu3SnS4 on High Selectivity and Activity of Photocatalytic CO2 Reduction. Appl. Catal. B Environ. 297, 120498. doi:10.1016/j.apcatb.2021.120498
Wang, R., Sun, P., Wang, H., and Wang, X. (2017). Pulsed Laser Deposition of Amorphous Molybdenum Disulfide Films for Efficient Hydrogen Evolution Reaction. Electrochimica Acta 258, 876–882. doi:10.1016/j.electacta.2017.11.138
Wang, X., Zhang, Y., Si, H., Zhang, Q., Wu, J., Gao, L., et al. (2020b). Single-Atom Vacancy Defect to Trigger High-Efficiency Hydrogen Evolution of MoS2. J. Am. Chem. Soc. 142 (9), 4298–4308. doi:10.1021/jacs.9b12113
Wang, Y.-l., Huang, Q., Sun, G.-q., Li, X.-y., Chen, L.-h., Su, B.-l., et al. (2021b). Synergistic Zinc Doping and Defect Engineering toward MoS2 Nanosheet Arrays for Highly Efficient Electrocatalytic Hydrogen Evolution. Dalton Trans. 50 (17), 5770–5775. doi:10.1039/D0DT04207B
Wang, Y.-l., Sun, G.-q., Chen, L.-h., Du, Z.-k., Li, X.-y., Ye, C.-f., et al. (2021c). Engineering Dual Defective Graphenes to Synergistically Improve Electrocatalytic Hydrogen Evolution. Appl. Surf. Sci. 566, 150712. doi:10.1016/j.apsusc.2021.150712
Wang, Y., Lu, F., Su, K., Zhang, N., Zhang, Y., Wang, M., et al. (2020c). Engineering Mo-O-C Interface in MoS2@rGO via Charge Transfer Boosts Hydrogen Evolution. Chem. Eng. J. 399, 126018. doi:10.1016/j.cej.2020.126018
Wang, Y., Qi, K., Yu, S., Jia, G., Cheng, Z., Zheng, L., et al. (2019b). Revealing the Intrinsic Peroxidase-Like Catalytic Mechanism of Heterogeneous Single-Atom Co-MoS2. Nano-Micro Lett. 11 (1), 102. doi:10.1007/s40820-019-0324-7
Xie, J., Zhang, H., Li, S., Wang, R., Sun, X., Zhou, M., et al. (2013a). Defect-Rich MoS2Ultrathin Nanosheets with Additional Active Edge Sites for Enhanced Electrocatalytic Hydrogen Evolution. Adv. Mat. 25 (40), 5807–5813. doi:10.1002/adma.201302685
Xie, J., Zhang, J., Li, S., Grote, F., Zhang, X., Zhang, H., et al. (2013b). Controllable Disorder Engineering in Oxygen-Incorporated MoS2 Ultrathin Nanosheets for Efficient Hydrogen Evolution. J. Am. Chem. Soc. 135 (47), 17881–17888. doi:10.1021/ja408329q
Xu, X., Shi, W., Li, P., Ye, S., Ye, C., Ye, H., et al. (2017). Facile Fabrication of Three-Dimensional Graphene and Metal-Organic Framework Composites and Their Derivatives for Flexible All-Solid-State Supercapacitors. Chem. Mat. 29 (14), 6058–6065. doi:10.1021/acs.chemmater.7b01947
Xu, Y., Wang, L., Liu, X., Zhang, S., Liu, C., Yan, D., et al. (2016). Monolayer MoS2with S Vacancies from Interlayer Spacing Expanded Counterparts for Highly Efficient Electrochemical Hydrogen Production. J. Mat. Chem. A 4 (42), 16524–16530. doi:10.1039/C6TA06534A
Yan, Y., Huang, J., Wang, X., Gao, T., Zhang, Y., Yao, T., et al. (2018). Ruthenium Incorporated Cobalt Phosphide Nanocubes Derived from a Prussian Blue Analog for Enhanced Hydrogen Evolution. Front. Chem. 6, 8. doi:10.3389/fchem.2018.00521
Yao, N., Li, P., Zhou, Z., Meng, R., Cheng, G., and Luo, W. (2019). Nitrogen Engineering on 3D Dandelion‐Flower‐Like CoS 2 for High‐Performance Overall Water Splitting. Small 15 (31), 1901993. doi:10.1002/smll.201901993
Yin, H., Zhao, S., Zhao, K., Muqsit, A., Tang, H., Chang, L., et al. (2015). Ultrathin Platinum Nanowires Grown on Single-Layered Nickel Hydroxide with High Hydrogen Evolution Activity. Nat. Commun. 6 (1), 6430. doi:10.1038/ncomms7430
Yu, H., Yu, X., Chen, Y., Zhang, S., Gao, P., and Li, C. (2015). A Strategy to Synergistically Increase the Number of Active Edge Sites and the Conductivity of MoS2 Nanosheets for Hydrogen Evolution. Nanoscale 7 (19), 8731–8738. doi:10.1039/C5NR00670H
Yuan, L., Zhang, Y., Chen, J., Li, Y., Ren, X., Zhang, P., et al. (2022). MoS2 Nanosheets Vertically Grown on CoSe2 Hollow Nanotube Arrays as an Efficient Catalyst for the Hydrogen Evolution Reaction. Nanoscale 14 (6), 2490–2501. doi:10.1039/D1NR05941F
Zhang, J., Xu, X., Yang, L., Cheng, D., and Cao, D. (2019a). Single‐Atom Ru Doping Induced Phase Transition of MoS 2 and S Vacancy for Hydrogen Evolution Reaction. Small Methods 3 (12), 1900653. doi:10.1002/smtd.201900653
Zhang, X., Zhou, F., Zhang, S., Liang, Y., and Wang, R. (2019b). Engineering MoS 2 Basal Planes for Hydrogen Evolution via Synergistic Ruthenium Doping and Nanocarbon Hybridization. Adv. Sci. 6 (10), 1900090. doi:10.1002/advs.201900090
Zhao, G., Lin, Y., Rui, K., Zhou, Q., Chen, Y., Dou, S. X., et al. (2018). Epitaxial Growth of Ni(OH)2 Nanoclusters on MoS2 Nanosheets for Enhanced Alkaline Hydrogen Evolution Reaction. Nanoscale 10 (40), 19074–19081. doi:10.1039/C8NR07045H
Zhou, G., Wu, X., Zhao, M., Pang, H., Xu, L., Yang, J., et al. (2021a). Interfacial Engineering‐Triggered Bifunctionality of CoS 2/MoS 2 Nanocubes/Nanosheet Arrays for High‐Efficiency Overall Water Splitting. ChemSusChem 14 (2), 699–708. doi:10.1002/cssc.202002338
Zhou, W., Dong, L., Tan, L., and Tang, Q. (2021b). First-principles Study of Sulfur Vacancy Concentration Effect on the Electronic Structures and Hydrogen Evolution Reaction of MoS2. Nanotechnology 32 (14), 145718. doi:10.1088/1361-6528/abd49f
Keywords: S-vacancy of MoS2, heterogeneous interfaces, exposed Mo atoms, electrocatalysis, hydrogen evolution reaction
Citation: Li X-Y, Zhu S-J, Wang Y-L, Lian T, Yang X-y, Ye C-F, Li Y, Su B-L and Chen L-H (2022) Synergistic Regulation of S-Vacancy of MoS2-Based Materials for Highly Efficient Electrocatalytic Hydrogen Evolution. Front. Chem. 10:915468. doi: 10.3389/fchem.2022.915468
Received: 08 April 2022; Accepted: 02 May 2022;
Published: 08 June 2022.
Edited by:
Liuqingqing Yang, Shanghai Jiao Tong University, ChinaReviewed by:
Guigao Liu, Nanjing University of Science and Technology, ChinaDafeng Yan, Huazhong University of Science and Technology, China
Copyright © 2022 Li, Zhu, Wang, Lian, Yang, Ye, Li, Su and Chen. This is an open-access article distributed under the terms of the Creative Commons Attribution License (CC BY). The use, distribution or reproduction in other forums is permitted, provided the original author(s) and the copyright owner(s) are credited and that the original publication in this journal is cited, in accordance with accepted academic practice. No use, distribution or reproduction is permitted which does not comply with these terms.
*Correspondence: Yi-Long Wang, d2FuZ3lpbG9uZ0B3aHV0LmVkdS5jbg==; Li-Hua Chen, Y2hlbmxpaHVhQHdodXQuZWR1LmNu