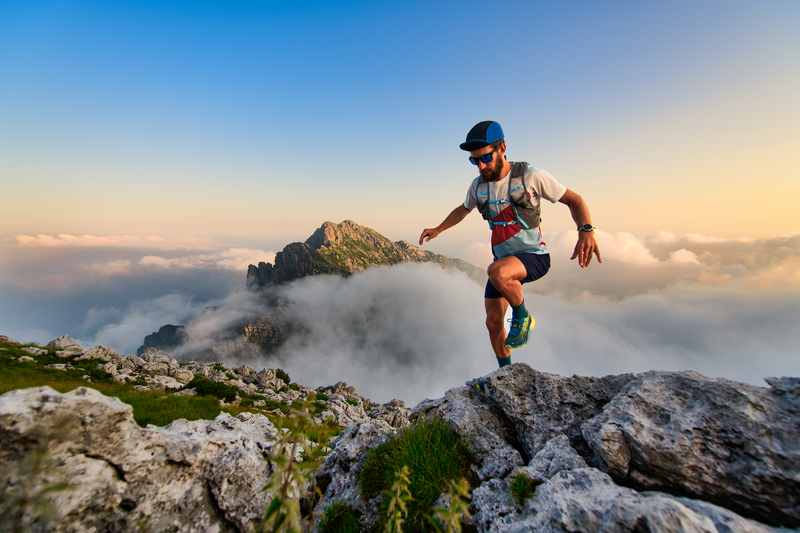
95% of researchers rate our articles as excellent or good
Learn more about the work of our research integrity team to safeguard the quality of each article we publish.
Find out more
MINI REVIEW article
Front. Chem. , 08 June 2022
Sec. Solid State Chemistry
Volume 10 - 2022 | https://doi.org/10.3389/fchem.2022.914930
This article is part of the Research Topic Interfacial Engineering of Carbon-Based Materials for Efficient Energy Conversion View all 6 articles
Lithium-ion batteries (LIBs) have attracted great attention as an advanced power source and energy-storage device for years due to their high energy densities. With rapid growing demands for large reversible capacity, high safety, and long-period stability of LIBs, more explorations have been focused on the development of high-performance cathode materials in recent decades. Carbon-based materials are one of the most promising cathode modification materials for LIBs due to their high electrical conductivity, large surface area, and structural mechanical stability. This feature review systematically outlines the significant advances of carbon-based materials for LIBs. The commonly used synthetic methods and recent research advances of cathode materials with carbon coatings are first represented. Then, the recent achievements and challenges of carbon-based materials in LiCoO2, LiNixCoyAl1-x-yO2, and LiFePO4 cathode materials are summarized. In addition, the influence of different carbon-based nanostructures, including CNT-based networks and graphene-based architectures, on the performance of cathode materials is also discussed. Finally, we summarize the challenges and perspectives of carbon-based materials on the cathode material design for LIBs.
Over the past decades, lithium-ion batteries (LIBs) have a wide range of applications due to their great advantages of low cost, long cycling lives, and high-energy densities, including portable electronic devices, electric vehicles, and grid energy storage (Okubo et al., 2010; Cui et al., 2016). In LIBs, lithium-based metal oxides act as a cathode and graphitic carbon acts as an anode, and the electrolyte is a liquid organic solvent containing lithium salt (Nara et al., 2019). As the important components of LIBs, cathode materials play a key role in electrochemical performance (Nitta et al., 2015). Up to now, many cutting-point cathodes have been successfully commercialized, such as LiCoO2, LiNixCoyAl1−x−yO2, and LiFePO4 cathodes (Li et al., 2020; Lu et al., 2020; Li et al., 2021a; Arabolla Rodríguez et al., 2021), promising LIBs a prominent position in the energy storage market. However, the cathode materials still face great challenges in practical applications, including slow charging, safety hazards, and high cost.
To solve these aforementioned problems, considerable efforts have been devoted to developing the following characteristics: 1) high ionic conductivity and Li diffusion coefficient; 2) high redox potential; 3) stable structure; 4) superior corrosion resistance; and 5) low cost (Du et al., 2019; Chakraborty et al., 2020; Logan and Dahn, 2020; Wu et al., 2020). Carbon-based materials like carbon nanofibers (CNFs) (Chen et al., 2022), carbon nanotubes (CNTs) (Li et al., 2021b; Chen et al., 2022), graphene (Chang et al., 2015), and their composites have been considered as crucial modifying agents owing to their outstanding properties, contributing to the improvement as follows: low cost, special structure, tunability, good dispersion, and stability. Moreover, the types of carbon-based materials show great differences in the dimensionality, morphology, and distribution of chemical bonding compared to traditional materials, which involve in the mixtures of local electronic structures between sp2 and sp3 (Palshin et al., 1995). As a result, fabricating cathode materials with carbon matrix can improve their conductivity and mitigate the volume variation.
In this review, we first summarize various strategies by using carbon-based materials to improve the properties of cathodes and their applications in LIBs. Furthermore, carbon-based materials in LiCoO2, LiNixCoyAl1−x−yO2, and LiFePO4 cathodes are discussed and summarized. Finally, the advantages and perspectives of the cathodes are also discussed.
The charge/discharge process in LIBs belongs to a chemical process of Li intercalation and deintercalation. The charge progress makes the cathode lithium deficient and the anode lithium rich, while the discharge process runs the other way. However, the structure of lithium-based metal oxides determines the charge/discharge rate and specific capacity compared to graphite anode. The cathode materials can be divided into the following types based on their crystal structures: layered structure, spinel structure, and olivine structure. Despite the advances, they still suffer from poor electrical conductivity, slow Li transport, unfavorable interactions with the electrolyte, low thermal stability, and high-volume expansion (Sun et al., 2016; Liu et al., 2018; Yuan et al., 2021). To solve the issues, various methods have been developed, including tuning morphology and structure, doping metallic cation, and integrating them with conductive carbon materials. Among them, it is an effective way to circumvent the problems by using conductive carbon materials to modify the cathode materials, such as constructing carbonaceous composites, doping carbon-based materials on cathodes, and coating carbon-based materials. In contrast, the advantages of the coating methods involve in providing good electrical conductivity, hindering the cathode dissolution and electrolyte degradation, and shortening the length of Li+ diffusion. As a result, to shed some light on the progress in cathodes, we summarize the recent reports of emerging carbon-based materials for various cathode materials by discussing their performance and underlying mechanisms (Figure 1).
FIGURE 1. Schematic diagram of carbon-based materials in addressing the challenges of LIBs in different cathodes. Reprinted with permission from Li H. et al. (2019) with permission from WILEY-VCH. Reprinted with permission from Lin J. et al. (2022), Wang X. et al. (2019), and Hwang. et al. (2020) with permission from Elsevier. Reprinted with permission from Lin J. et al. (2020) with permission from The Royal Society of Chemistry. Reprinted with permission from Cheng Q. et al. (2019) with permission from the American Chemical Society.
Layered LiMO2 (M = Co, Mn, Ni) oxides have been considered one of the most common cathode materials for LIBs. LiCoO2 is first employed as the intercalation cathode for LIBs by Goodenough, Mizushima et al. (1980), and Kubota (2020). The LiCoO2 cathode has the advantages of high theoretical specific capacity (274 mAh g−1), high theoretical volumetric capacity (1,363 mAh cm−3), high discharge voltage, and good cycling performance. However, the reversible intercalation/deintercalation amount of Li+ is only 0.5 units since the layered-structure collapse by LiCoO2 lattice distorting from hexagonal to monoclinic symmetry when discharge takes place deeply. In addition, LiCoO2 would emit oxygen during the charging process at high temperatures, which leads to volume change, resulting in a lower capacity.
It has been reported numerous efforts to enhance the LiCoO2 by using advanced materials, especially carbon-based materials. Wang reported the sandwich-structured LiCoO2 cathodes by using cross-stacked super-aligned carbon nanotubes (SACNTs) (Yan et al., 2017). The cathodes consist of a repeating and alternating stack of LiCoO2 layers and SACNT films, in which each layer of active materials could adhere to the SACNT conductive layers, realizing sufficient electron transfer throughout the electrodes. The sandwiched LiCoO2 cathode could deliver a specific discharge capacity of 109.6 mAh g−1 at 10C, demonstrating much higher energy densities. In addition, ZIF-67 precursors were annealed with Li2CO3 under air and followed by homogeneous AlF3 coating and carbon nanotubes wrapping, which can provide rapid internal electron channels and the external surface AlF3-coating (Cheng et al., 2019). Therefore, AlF3-coated LiCoO2/CNTs can enhance lithium storage performance at both room temperature and an elevated temperature (50°C) and illustrate an excellent rate and cycling performance with 120 mAh g−1 at 1C after 200 cycles. It can be seen that CNT materials not only lower the energy barrier but also improve the rate performance (Table 1).
Su designed an in-situ modification strategy to synthesize a metal–organic framework (MOF)-derived LiCoO2 heterostructure (Lin et al., 2020). The LiCoO2@C cathode shows long cycling life (171.1 mAh g−1 at 1C after 200 cycles) and superior rate capability (150.3 mAh g−1 even at 10C). Both experimental and DFT results prove that an in-situ MOF-derived carbon-coating strategy can not only reduce direct contact resistance between the LiCoO2 bulk and electrolyte but also boost the electrochemical conductivity and strain structural distortion, resulting in enhanced cyclability and rate performance. In addition, the low energy barriers of Li-vacancy migration pathways on the LiCoO2@C heterostructure are helpful to build a systematic diffusion network. Lee found that the LiF-C layer formed on LiCoO2 by defluorination reaction with carbon monofluoride at 400°C can suppress side reactions at the electrolyte/electrode interface (Lim et al., 2021). As a result, the LiCoO2 cathode with the LiF-C layers could deliver an initial discharge capacity of 186 mAh g−1 at 0.1C and achieve a long-term cycle at 4.5 V. Importantly, the modified LiCoO2 cathode indicates excellent cycling and rate performance, which delivers 161 mAh g−1 after 180 cycles and 115 mAh g−1 at 10C.
In general, the structural stability and cycle performance of LiCoO2 can be greatly improved by carbon-based materials at high voltage operation (>4.2 V). Constructing carbon-based coating is a promising synthetic approach to enhance the structural property and electrochemical performance of LiCoO2 cathode. The main contribution of carbon-based materials is to efficiently improve the electrical conductivity within the cathode host and reduce the direct contact resistance between the LiCoO2 bulk and electrolyte, leading to the extensive application of LiCoO2 cathodes in LIBs with good rate performance and cycling life.
The further massive usage of LiCoO2 cathodes for LIBs has been severely hindered by the sharp rising cost and limitation of cobalt resources. Considerable works have been committed to developing less Co or Co-free cathode materials, such as LiNiO2, LiNi0.8Co0.2O2, and LiNixCoyAl1-x-yO2. Until now, the most prospective alternative for LiCoO2 cathode is LiNixCoyAl1-x-yO2 (NCA) due to its affordable cost, high-energy density, and structural stability (Liu et al., 2015; Zheng et al., 2017). Tesla company first employs NCA cathodes to drive cars, and it has achieved remarkable success in the electric vehicle industry. However, Ni2+ ions could easily occupy the vacancy of Li+ ions during the charging process, which may increase the degree of cation mixing and generate irreversible phases, resulting in capacity loss. In addition, the thermal stability of NCA should be promoted for the reduction of unstable Ni3+ at high temperatures, which would destroy the layered structure.
To overcome the shortages of NCA, the modification of carbon-based materials can effectively enhance ionic conductivity and cycle performance by changing the transmission mechanism and increasing the electrical conductivity. Many approaches have been developed to coat LiNixCoyAl1-x-yO2 by using carbon-based materials, including atomic layer deposition (ALD) (Gupta et al., 2022), wet chemical method (Han et al., 2019), and chemical vapor deposition (CVD). The hydrolysis–condensation method was designed to synthesize the NCA-ethoxy-functional groups (EPS) cathodes, which can effectively suppress the side reactions and mitigate the instability of pristine NCA (Visbal et al., 2016). The cycling stability of the NCA-EPS cathode was significantly improved, in which EPS as a protective shell can reduce the trace water on the secondary particle surface and inhibit the side reaction. Visbal et al. (2016) used a CVD approach to investigate the impact of diamond-like carbon (DLC) layers on LiNi0.8Co0.15Al0.05O2 materials. The chemical inertness of the DLC coating can contribute to preventing unwanted reactions between the cathode active material and sulfide solid electrolyte. That is because the DLC layers consist of sp3 and sp2 carbon structures, which have superior properties, including high hardness, chemical inertness, and high thermal conductivity.
A solvent-free and economically feasible process was designed by using electrically conductive nanocarbon, which can produce LiNi0.8Co0.15Al0.05O2-carbon composites (Vadivel et al., 2019). LiNi0.8Co0.15Al0.05O2-carbon compounds could keep their initial structure under the high-speed operation (∼5,000 rpm) and display high reversible capacity and cycle performance (91% at 0.1C and 84% retention at 0.5C after 250 cycles). Interestingly, exfoliated graphene nanosheets that were functionalized with an amphiphilic surfactant were employed as the coatings (Park et al., 2021). The graphene nanosheets can attach onto Ni-rich oxides in a face-to-face manner owing to the adhesive amphiphilic surfactant. By eliminating the conventional conductive additive and minimizing the binder content, the graphene-coated electrode demonstrates a highly dense 99 wt% NCA (electrode density ∼4.3 g cm−3) with a high areal capacity of ∼5.4 mAh cm−2 (∼38% increase) and high volumetric capacity of ∼863 mAh cm−3 (∼34% increase) at a current rate of 0.2 C (∼1.1 mA cm−2), relative to the bare electrode with a commercial level of 96 wt% NCA (electrode density, ∼3.3 g cm−3).
As the prospective cathode of LIBs, it should pay more attention to the performance degradation and safety hazard of NCA materials, which are raised from aggressive chemical, structural, and mechanical deterioration and thermodynamic instability. The carbon-based coating can effectively improve the ion/electron conductivity and cycle performance of NCA materials by increasing the electronic transport pathways and changing the transmission mechanism. Therefore, the carbon-based coating can protect the structure of NCA particles from collapsing by puzzled side reactions and remarkably improve the stability of the battery during cycling.
LiFePO4 belongs to the lithium ortho-phosphates with an orthorhombic lattice structure, which takes many advantages, including low cost, thermal stability, and environmental compatibility. However, the slow intrinsic electronic conductivity, poor Li+ diffusion coefficient, and relatively low theoretical capacity obstruct their applications in high-energy-density fields. Carbon-based materials can increase electron migration and Li+ transfer and prevent particle aggregation, which have become the crucial materials to improve electrode properties.
To realize excellent rate performance, LiFePO4/reduced graphene oxide (RGO) hybrid was synthesized via a homogeneous coprecipitation method, which demonstrates a specific capacity of 172 mAh g−1 at 0.06C and 139 mAh g−1 at 11.8C (Zhu et al., 2014). LiFePO4 particles are uniformly and closely anchored upon conductive RGO sheets, which improve electron/ion transmission and prevent LiFePO4 particle aggregation. Ultrathin conformal carbon was coated on LiFePO4 nano/microspheres by using liquid carbon dioxide, exhibiting good reversible capacity (168 mAh g−1 at 0.1C), high energy density, and excellent long-term cyclability (84% cycle retention at 10C after 1,000 cycles) (Hwang et al., 2017). The average thickness of the carbon layer distributed over the primary LiFePO4 particles is 1.9 nm, providing facile penetration of liquid electrolytes and a rapid ion/electron transmission channel. Moreover, the high tap density (1.4 g cm−3) of the ultrathin carbon layer contributes to a high volumetric energy density (458 Wh L−1 at a 30C rate).
Fluorine-doped carbon (FC) materials are introduced to coat the LiFePO4 cathode by using polyvinylidene fluoride. A three-dimensional conductive network structure was obtained, in which FC is observed to attach to the LiFePO4 cathode (Wang et al., 2020). The LiFePO4 cathode with FC coating can deliver an attractive cycling stability over 1,000 cycles and possess a high-rate capability with maintaining 100.2 mAh g−1 at 20C, due to the shortening of Li+ diffusion distance and the rapid transfer of electrons. Moreover, C-F covalent bonds improve the strong chemical affinity between the electrolyte and LiFePO4 electrode because of their excellent permeation in the electrolyte. Lin et al. (2022) reported versatile LiFePO4 microparticles encapsulated in an O, F-codoped carbon matrix (LFP@OFC) via solid-state sintering. The O, F-codoped porous carbon matrix is energetically preferable for superior adsorption capability coupled with decreased diffusion barriers of Li+, contributing to abundant active sites, boosted electronic conductivity, and expedited diffusion aisles. Therefore, LFP@OFC could deliver a specific capacity (169.9 mAh g−1 at 0.1C) and have rate capacity (85.6 mAh g−1 even at 16.2C) and long-term cyclability (160.9 mAh g−1 over 500 cycles at 1C).
LiFePO4 has been widely acknowledged for its poor electronic conductivity (∼10−9 S cm−1) and low Li+ diffusion coefficient (∼10−14 cm2 s−1). To improve its capacity and rate performance, many approaches have been proposed with organic or inorganic carbon sources, such as citric acid, lactose and glucose, super P, carbon nanotubes (CNTs), and graphene. The basic purpose of the carbon-based coating is to cover LiFePO4 particles with a uniform conductive layer to improve the surface conductivity and utilize the active material at high rates. In addition, carbon coating can also prevent side reactions at the electrode–electrolyte interface and restrict the growth of the crystal.
In summary, carbon-based materials have been widely used due to their excellent stability and high electronic conductivity in LIBs. The electrochemical performance of typical cathodes has been greatly improved due to the protective effect of carbon coating. Carbon-based coatings not only construct the conductive ion/electron transfer path between active cathodes and electrolytes but also play an important role in restricting the cathode dissolution and electrolyte degradation. Therefore, the primary purpose of carbon-based materials on the surface of the LiCoO2 cathode is to stabilize the crystal structure at high-voltage (over 4.2 V) cycling, inhibiting the detrimental phase transition from the hexagonal structure to monoclinic structure. The carbon-based materials can prevent the crystal structure of LiNixCoyAl1-x-yO2 from collapsing due to the side reaction since the poor thermal stability of Ni3+ in a lattice structure is extremely unstable under high temperature and easily reactive with the HF. The most important improvement of carbon-based materials for LiFePO4 is to increase its intrinsic slow Li-ion diffusion and electronic conductivity, especially for high-rate performance.
Despite the great success of LIBs, the dramatic increase of energy-density need still promotes the fast development of cathode materials with superior specific capacity and affordable cost. As originally commercialized by SONY in the 1990s, LiCoO2 will continue its great success in the consumer electronic device industry if breaking through its practical energy density by further elevating charge cut-off voltage higher than 4.5 V. Meanwhile, LiNixCoyAl1-x-yO2 may play an important role in the practical fields of high-energy density. With consideration of its outstanding advantages in safety performance and thermal stability, LiFePO4 could be predicted to dominate the application market where prioritizing security and cost among the major commercialized cathodes. Carbon-coating engineering has been extensively studied to separate cathodes from electrolyte solutions and improve conductivity. It is also essential to take into consideration the compatibility of the modified cathode with the electrolyte and anode. To satisfy the demands of large-scale production, the modification process should avoid the usage of energy-intensive methods. In addition, carbon-based materials should be obtained easily from a wide range of sources. Undeniably, there is still a huge development potential for persistent exploration of carbon-based materials as a breakthrough research direction for further pursuing the higher energy density, better property, and longer cycle life of batteries.
All authors listed have made a substantial, direct, and intellectual contribution to the work and approved it for publication.
This work was supported by the Natural Science Foundation of Jiangsu Province (No. BK20200960), the Natural Science Foundation of Higher Education in Jiangsu Province (No. 20KJB150041), and the Natural Science Foundation of Nantong University for High-Level Talent (No. 03083033).
The authors declare that the research was conducted in the absence of any commercial or financial relationships that could be construed as a potential conflict of interest.
The handling editor YM declared a past co-authorship with the author LZ.
All claims expressed in this article are solely those of the authors and do not necessarily represent those of their affiliated organizations, or those of the publisher, the editors, and the reviewers. Any product that may be evaluated in this article, or claim that may be made by its manufacturer, is not guaranteed or endorsed by the publisher.
Arabolla Rodríguez, R., Della Santina Mohallem, N., Avila Santos, M., Sena Costa, D. A., Andrey Montoro, L., and Mosqueda Laffita, Y. (2021). Unveiling the Role of Mn-Interstitial Defect and Particle Size on the Jahn-Teller Distortion of the LiMn2O4 Cathode Material. J. Power Sources 490, 229519. doi:10.1016/j.jpowsour.2021.229519
Chakraborty, A., Kunnikuruvan, S., Kumar, S., Markovsky, B., Aurbach, D., Dixit, M., et al. (2020). Layered Cathode Materials for Lithium-Ion Batteries: Review of Computational Studies on LiNi1-X-yCoxMnyO2 and LiNi1-X-yCoxAlyO2. Chem. Mater. 32, 915–952. doi:10.1021/acs.chemmater.9b04066
Chang, J., Huang, X., Zhou, G., Cui, S., Mao, S., and Chen, J. (2015). Three-dimensional Carbon-Coated Si/rGO Nanostructures Anchored by Nickel Foam with Carbon Nanotubes for Li-Ion Battery Applications. Nano Energy 15, 679–687. doi:10.1016/j.nanoen.2015.05.020
Chen, C., Lin, S., Wu, Y., Su, J., Cheng, C., Cheng, P., et al. (2022). MOF-derived Cobalt Disulfide/nitrogen-Doped Carbon Composite Polyhedrons Linked with Multi-Walled Carbon Nanotubes as Sulfur Hosts for Lithium-Sulfur Batteries. Chem. Eng. J. 431, 133924. doi:10.1016/j.cej.2021.133924
Chen, L., Lin, X., Gao, J., Zou, M., Huang, Y., Zhao, G., et al. (2022). Porous Carbon Nanofibers as Anode for High-Performance Potassium-Ion Batteries. Electrochim. Acta 403, 139654. doi:10.1016/j.electacta.2021.139654
Cheng, Q., Zhou, J., Ke, C., Qin, L., Lin, X., Lokesh, B., et al. (2019). Method for Synthesis of Zeolitic Imidazolate Framework-Derived LiCoO2/CNTs@AlF3 with Enhanced Lithium Storage Capacity. Inorg. Chem. 58, 11993–11996. doi:10.1021/acs.inorgchem.9b01727
Cui, S., Wei, Y., Liu, T., Deng, W., Hu, Z., Su, Y., et al. (2016). Optimized Temperature Effect of Li-Ion Diffusion with Layer Distance in Li(NixMnyCoz)O2 Cathode Materials for High Performance Li-Ion Battery. Adv. Energy Mater 6. doi:10.1002/aenm.201501309
Du, F., Sun, P., Zhou, Q., Zeng, D., Hu, D., Fan, Z., et al. (2020). Interlinking Primary Grains with Lithium Boron Oxide to Enhance the Stability of LiNi0.8Co0.15Al0.05O2. ACS Appl. Mater. Interfaces 12, 56963–56973. doi:10.1021/acsami.0c16159
Du, M., Liao, K., Lu, Q., and Shao, Z. (2019). Recent Advances in the Interface Engineering of Solid-State Li-Ion Batteries with Artificial Buffer Layers: Challenges, Materials, Construction, and Characterization. Energy Environ. Sci. 12, 1780–1804. doi:10.1039/c9ee00515c
Gui, S., Zhang, Q., Zhuo, H., and Liu, J. (2019). Enhancing the Electrochemical Performance of LiNi0.8Co0.15Al0.05O2 by a Facile Doping Method: Spray-drying Doping with Liquid Polyacrylonitrile. J. Power Sources. 409, 102–111. doi:10.1016/j.jpowsour.2018.11.001
Gupta, B., Hossain, M. A., Riaz, A., Sharma, A., Zhang, D., Tan, H. H., et al. (2022). Recent Advances in Materials Design Using Atomic Layer Deposition for Energy Applications. Adv. Funct. Mater 32, 2109105. doi:10.1002/adfm.202109105
Han, B., Key, B., Lipton, A. S., Vaughey, J. T., Hughes, B., Trevey, J., et al. (2019). Influence of Coating Protocols on Alumina-Coated Cathode Material: Atomic Layer Deposition versus Wet-Chemical Coating. J. Electrochem. Soc. 166, 3679–3684. doi:10.1149/2.0681915jes
Hwang, J., Kong, K. C., Chang, W., Jo, E., Nam, K., and Kim, J. (2017). New Liquid Carbon Dioxide Based Strategy for High Energy/power Density LiFePO4. Nano Energy 36, 398–410. doi:10.1016/j.nanoen.2017.04.046
Kubota, K. (2020). Electrochemistry and Solid-State Chemistry of Layered Oxides for Li-, Na-, and K on Batteries. Electrochemistry 88, 507–514. doi:10.5796/electrochemistry.20-00092
Li, H., Peng, L., Wu, D., Wu, J., Zhu, Y. J., and Hu, X. J. (2019). Ultrahigh‐capacity and Fire‐resistant LiFePO4-Based Composite Cathodes for Advanced Lithium-Ion Batteries. Adv. Energy Mater. 9, 1802930. doi:10.1002/aenm.201802930
Li, P., Guo, X., Zang, R., Wang, S., Zuo, Y., Man, Z., et al. (2021). Nanoconfined SnO2/SnSe2 Heterostructures in N-Doped Carbon Nanotubes for High-Performance Sodium-Ion Batteries. Chem. Eng. J. 418, 129501. doi:10.1016/j.cej.2021.129501
Li, P., Zhu, J., Wang, C., Wang, J., Yang, X., Zhang, K., et al. (2021). Preparation of Cathode Material with LiMn2O4 Using Conductive Carbon-Sodium Alginate as Three Dimensional Collector System. Electrochim. Acta 389, 138784. doi:10.1016/j.electacta.2021.138784
Li, Z., Li, A., Zhang, H., Ning, F., Li, W., Zangiabadi, A., et al. (2020). Multi-scale Stabilization of High-Voltage LiCoO2 Enabled by Nanoscale Solid Electrolyte Coating. Energy Stor. Mater. 29, 71–77. doi:10.1016/j.ensm.2020.03.031
Lim, J., Myung, Y., Yang, M., and Lee, J. (2021). Facile Formation of a LiF-Carbon Layer as an Artificial Cathodic Electrolyte Interphase through Encapsulation of a Cathode with Carbon Monofluoride. ACS Appl. Mater. Interfaces 13, 31741–31748. doi:10.1021/acsami.1c08419
Lin, J., Sun, Y. H., and Lin, X. (2022). Metal-organic Framework-Derived LiFePO4 Cathode Encapsulated in O,F-codoped Carbon Matrix towards Superior Lithium Storage. Nano Energy 91, 106655. doi:10.1016/j.nanoen.2021.106655
Lin, J., Zeng, C., Chen, Y., Lin, X., Xu, C., and Su, C. (2020). In Situ construction of a MOF-Derived Carbon-Encapsulated LiCoO2 Heterostructure as a Superior Cathode for Elevated-Voltage Lithium Storage: from Experimental to Theoretical Study. J. Mater. Chem. A 8, 6607–6618. doi:10.1039/D0TA00679C
Liu, K., Liu, Y., Lin, D., Pei, A., and Cui, Y. (2018). Materials for Lithium-Ion Battery Safety. Sci. Adv. 4, eaas9820. doi:10.1126/sciadv.aas9820
Liu, W., Oh, P., Liu, X., Lee, M. J., Cho, W., Chae, S., et al. (2015). Nickel-rich Layered Lithium Transition-Metal Oxide for High-Energy Lithium-Ion Batteries. Angew. Chem. Int. Ed. 54, 4440–4457. doi:10.1002/anie.201409262
Logan, E. R., and Dahn, J. R. (2020). Electrolyte Design for Fast-Charging Li-Ion Batteries. Trends Anal. Chem. 2, 354–366. doi:10.1016/j.trechm.2020.01.011
Lu, J., Ran, H., Li, J., Wan, J., Wang, C., Ji, P., et al. (2020). A Fast Composite-Hydroxide-Mediated Approach for Synthesis of 2D-LiCoO2 for High Performance Asymmetric Supercapacitor. Electrochim. Acta 331, 135426. doi:10.1016/j.electacta.2019.135426
Mizushima, K., Jones, P. C., Wiseman, P. J., and Goodenough, J. B. (1980). LixCoO2 (0<x<-1): A New Cathode Material for Batteries of High Energy Density. Mater. Res. Bull. 15, 783–789. doi:10.1016/0025-5408(80)90012-4
Nara, H., Mukoyama, D., Shimizu, R., Momma, T., and Osaka, T. (2019). Systematic Analysis of Interfacial Resistance between the Cathode Layer and the Current Collector in Lithium-Ion Batteries by Electrochemical Impedance Spectroscopy. J. Power Sources 409, 139–147. doi:10.1016/j.jpowsour.2018.09.014
Nitta, N., Wu, F., Lee, J. T., and Yushin, G. (2015). Li-ion Battery Materials: Present and Future. Mater. Today 18, 252–264. doi:10.1016/j.mattod.2014.10.040
Okubo, M., Mizuno, Y., Yamada, H., Kim, J., Hosono, E., Zhou, H., et al. (2010). Fast Li-Ion Insertion into Nanosized LiMn2O4 without Domain Boundaries. ACS Nano 4, 741–752. doi:10.1021/nn9012065
Palshin, V., Meletis, E. I., Ves, S., and Logothetidis, S. (1995). Characterization of Ion-Beam-Deposited Diamond-like Carbon Films. Thin Solid Films 270, 165–172. doi:10.1016/0040-6090(95)06912-7
Park, C. W., Lee, J. H., Seo, J. K., Jo, W. Y., Whang, D., Hwang, S. M., et al. (2021). Graphene Collage on Ni-Rich Layered Oxide Cathodes for Advanced Lithium-Ion Batteries. Nat. Commun. 12, 2145. doi:10.1038/s41467-021-22403-w
Saikia, D., Deka, J. R., Chou, C. J., Lin, C. H., Yang, Y. C., and Kao, H. M. (2019). Encapsulation of LiFePO4 Nanoparticles into 3D Interpenetrating Ordered Mesoporous Carbon as a High-Performance Cathode for Lithium-Ion Batteries Exceeding Theoretical Capacity. ACS Appl. Energy Mater. 2, 1121–1133. doi:10.1021/acsaem.8b01682
Sun, Y., Liu, N., and Cui, Y. (2016). Promises and Challenges of Nanomaterials for Lithium-Based Rechargeable Batteries. Nat. Energy 1, 16071. doi:10.1038/nenergy.2016.71
Tian, F., Nie, W., Zhong, S., Liu, X., Tang, X., Zhou, M., et al. (2020). Highly Ordered Carbon Nanotubes to Improve the Conductivity of LiNi0.8Co0.15Al0.05O2 for Li-Ion Batteries. J. Mater. Sci. 55, 12082–12090. doi:10.1007/s10853-020-04809-x
Vadivel, S., Phattharasupakun, N., Wutthiprom, J., duangdangchote, S., and Sawangphruk, M. (2019). High-performance Li-Ion Batteries Using Nickel-Rich Lithium Nickel Cobalt Aluminium Oxide-Nanocarbon Core–Shell Cathode: in Operando X-Ray Diffraction. ACS Appl. Mater. Interfaces 11, 30719–30727. doi:10.1021/acsami.9b06553
Visbal, H., Aihara, Y., Ito, S., Watanabe, T., Park, Y., and Doo, S. (2016). The Effect of Diamond-like Carbon Coating on LiNi0.8Co0.15Al0.05O2 Particles for All Solid-State Lithium-Ion Batteries Based on Li2S–P2s5 Glass-Ceramics. J. Power Sources 314, 85–92. doi:10.1016/j.jpowsour.2016.02.088
Wang, X., Feng, Z., Hou, X., Liu, L., He, M., He, X., et al. (2020). Fluorine Doped Carbon Coating of LiFePO4 as a Cathode Material for Lithium-Ion Batteries. Chem. Eng. J. 379, 122371. doi:10.1016/j.cej.2019.122371
Wu, F., Maier, J., and Yu, Y. (2020). Guidelines and Trends for Next-Generation Rechargeable Lithium and Lithium-Ion Batteries. Chem. Soc. Rev. 49, 1569–1614. doi:10.1039/c7cs00863e
Yan, L., Wang, K., Luo, S., Wu, H., Luo, Y., Yu, Y., et al. (2017). Sandwich-structured Cathodes with Cross-Stacked Carbon Nanotube Films as Conductive Layers for High-Performance Lithium-Ion Batteries. J. Mater. Chem. A 5, 4047–4057. doi:10.1039/C6TA10024D
Yuan, B., Wen, K., Chen, D., Liu, Y., Dong, Y., Feng, C., et al. (2021). Composite Separators for Robust High Rate Lithium Ion Batteries. Adv. Funct. Mater. 31. doi:10.1002/adfm.202101420
Zheng, J., Myeong, S., Cho, W., Yan, P., Xiao, J., Wang, C., et al. (2017). Li- and Mn-Rich Cathode Materials: Challenges to Commercialization. Adv. Energy Mater. 7, 1601284. doi:10.1002/aenm.201601284
Keywords: carbon-based materials, interfacial engineering, cathode materials, synthetic strategies, lithium-ion batteries
Citation: Zhou L, Yang H, Han T, Song Y, Yang G and Li L (2022) Carbon-Based Modification Materials for Lithium-ion Battery Cathodes: Advances and Perspectives. Front. Chem. 10:914930. doi: 10.3389/fchem.2022.914930
Received: 07 April 2022; Accepted: 19 April 2022;
Published: 08 June 2022.
Edited by:
Yulin Min, Shanghai University of Electric Power, ChinaReviewed by:
Jinchen Fan, University of Shanghai for Science and Technology, ChinaCopyright © 2022 Zhou, Yang, Han, Song, Yang and Li. This is an open-access article distributed under the terms of the Creative Commons Attribution License (CC BY). The use, distribution or reproduction in other forums is permitted, provided the original author(s) and the copyright owner(s) are credited and that the original publication in this journal is cited, in accordance with accepted academic practice. No use, distribution or reproduction is permitted which does not comply with these terms.
*Correspondence: Linsen Li, bGluc2VubGlAc2p0dS5lZHUuY24=
†These authors have contributed equally to this work
Disclaimer: All claims expressed in this article are solely those of the authors and do not necessarily represent those of their affiliated organizations, or those of the publisher, the editors and the reviewers. Any product that may be evaluated in this article or claim that may be made by its manufacturer is not guaranteed or endorsed by the publisher.
Research integrity at Frontiers
Learn more about the work of our research integrity team to safeguard the quality of each article we publish.