- 1Jiangsu Co-Innovation Center of Efficient Processing and Utilization of Forest Resources, College of Chemical Engineering, Nanjing Forestry University, Nanjing, China
- 2Jiangsu Provincial Key Lab for the Chemistry and Utilization of Agro-forest Biomass, College of Chemical Engineering, Nanjing Forestry University, Nanjing, China
Lignocellulose is recognized as an ideal raw material for biorefinery as it may be converted into biofuels and value-added products through a series of chemical routes. Furfural, a bio-based platform chemical generated from lignocellulosic biomass, has been identified as a very versatile alternative to fossil fuels. Deep eutectic solvents (DES) are new “green” solvents, which have been employed as green and cheap alternatives to traditional organic solvents and ionic liquids (ILs), with the advantages of low cost, low toxicity, and biodegradability, and also have been proven to be effective media for the synthesis of biomass-derived chemicals. This review summarizes the recent advances in the conversion of carbohydrates to furfural in DES solvent systems, which mainly focus on the effect of adding different catalysts to the DES system, including metal halides, water, solid acid catalyst, and certain oxides, on the production of furfural. Moreover, the challenges and perspectives of DES-assisted furfural synthesis in biorefinery systems are also discussed in this review.
1 Introduction
Lignocellulose is a prominent biorefining raw material due to its wide availability and renewability, which helps to alleviate energy depletion (Somerville et al., 2010). In most lignocellulosic feedstocks, the proportions of cellulose, hemicellulose, and lignin are 30%–40%, 30%–35%, and 11%–25%, respectively. (Wang et al., 2016). Cellulose and hemicellulose separated from lignocellulose can be converted to high-value platform chemicals (e.g., 5-HMF and furfural) (Supplementary figure S1) (Bozell and Petersen, 2010).
Furfural is mainly obtained by hydrolysis and dehydration of xylan, which is abundant in hemicellulose and serves as a bridge between biomass and fuels, and chemicals. Furfural is widely used in oil refining, plastics manufacturing, pharmaceutical, and agrochemical industries, as well as a direct gasoline additive (Song et al., 2017; Luo et al., 2019; Song et al., 2020). Inorganic acids are utilized as the preferred catalysts for furfural production in the industry because furfural is produced in an acidic reaction system. High operating expenses, equipment corrosion, low furfural yields, and high energy consumption are all issues for this process (Mittal et al., 2017). Extraction of furfural from the reaction medium employing efficient catalysts or extraction solvents is a viable way for improving furfural yields. Some non-protonic solvents (e.g., tetrahydrofuran (THF), methyl isobutyl ketone (MIBK), and γ-pentyl lactone) are interesting solvents for furfural extraction because of their good ability to extract furfural. To extract furfural into the organic phase while minimizing side reactions in the aqueous phase, a biphasic reaction system is used. However, high reaction temperatures (150–230°C) are required for aqueous/organic biphasic systems (Romo et al., 2018). Ionic liquids with good solubility, chemical, and thermal stability, and enhanced catalytic activity were found to be alternative solvents, and polysaccharide dehydration in ionic liquids could be achieved at temperatures below 140°C (Wang et al., 2017; Wu et al., 2017). However, ionic liquids, are expensive and have poor biocompatibility.
Alternative deep eutectic solvents (DES) have been considered as a result of ongoing research on ionic liquids, which have similar properties to ionic liquids but are less expensive and entirely biodegradable (Hansen et al., 2021). DES are eutectic mixtures of hydrogen bond acceptor (HBA) and hydrogen bond donor (HBD) mixed in a certain ratio with a melting point lower than that of either component. For example, melting temperatures of chloroform and urea are 302°C and 135°C, respectively, and the resulting molar ratio of 1:2 chloroform/urea DES is liquid at 12°C (Abbott et al., 2003). DES has been used in biomass processing, for instance, fractionation and pretreatment of lignocellulose, as well as the catalytic conversion of carbohydrates (Satlewal et al., 2018).
The first half of this paper briefly covers DES and the mechanism of DES interacting with lignocellulose, with a focus on furfural synthesis. The second section summarizes the latest advances in the addition of various catalysts to DES systems for furfural production. This review also puts forward the challenges and perspectives for producing furfural from biomass using DES solvents.
2 Deep Eutectic Solvents
Deep Eutectic Solvents is a low melting point mixture of two or more component pairs consisting of HBD interacting with HBA (e.g., quaternary ammonium salts) via hydrogen bonding. Charge separation domains are promoted by hydrogen bonds (H-bonds) between HBA and HBD, showing a large decrease in the melting point of the DES mixture compared to the initial compounds, and the stronger H-bonds result in a bigger fall in melting point (Abbott et al., 2004). DES has been successfully applied in metallurgy and electrodeposition (Abbott et al., 2001; Abbott et al., 2006), biomass processing (Abbott et al., 2007; Francisco et al., 2012), fuel desulfurization (Jablonský et al., 2015), CO2 adsorption (Li et al., 2013), biodiesel purification (Li et al., 2008), biotransformation (Abbott et al., 2005), etc. since its first appearance in 2001 (Morrison et al., 2009). It can also be used as an effective extractant and has a wide range of applications in analytical sciences (Cunha and Fernandes, 2018), including chromatographic separation (Cai and Qiu, 2019), electrochemical analysis, liquid and solid sample decomposition (Chen Y.-L. et al., 2018), synthesis and modification of novel adsorbent materials (Hashemi et al., 2018).
2.1 Deep Eutectic Solvents Preparation and Principle
Autocorrelation intermolecular interactions, which are most likely induced by mixed entropy, van der Waals contacts, hydrogen bonding, and/or ionic bonding (Figure 1, exemplified for choline ChCl/urea 1:2), are responsible for the formation of DES (Abbott et al., 2003; Francisco et al., 2013; Hammond et al., 2016; Zahn et al., 2016; Mohd Zaid et al., 2017; Wagle et al., 2017; Delgado-Mellado et al., 2018; van Osch et al., 2019). Hydrogen bonds are the most important intramolecular bonds among these bonds between HBD and halide anions in DES (Perkins et al., 2014; Mbous et al., 2017). Hydrogen bonds are responsible for the generation of the room temperature liquid phase of DES (Smith et al., 2014), the enormous network of hydrogen bonds that generates the inherent qualities of DES, such as low melting point, low volatility, non-flammability, low vapor pressure, dipole nature, thermal stability, high solubility, and adjustability (Zhang et al., 2012; Florindo et al., 2014).
Heating and stirring, vacuum evaporation, grinding, and freeze-drying are the most common methods for preparing DES. The most common preparation method is heating and stirring, in which the components are mixed and stirred for a certain time at a set temperature (50°C–100°C) until a clear liquid is generated (Florindo et al., 2014; Yang et al., 2019). In addition, the excessive temperature may influence the formation of DES by this approach, resulting in the production of some contaminants (esters, HCl) (Florindo et al., 2014). Grinding is a better method when ChCl and carboxylic acid are used to obtain DES because the mixed components are ground in a mortar at room temperature until a homogeneous liquid is formed (Florindo et al., 2014). The evaporation method involves dissolving the components in water, evaporating most of the water under a vacuum at 50°C, and then drying it to a consistent weight in a desiccator (Dai et al., 2013). The components are dissolved in 5% water and then freeze-dried to generate a clear and transparent liquid (Gutierrez et al., 2009).
2.2 Deep Eutectic Solvents Categories
Most DES are obtained from non-ionic substances, such as salts and molecular components (Figure 2) (Zhang et al., 2012). The general formula for DES is Cat+X−zY (Smith et al., 2014). DES was first proposed and classified into four categories by Abbott et al., in 2003 (Table 1) (Abbott et al., 2003) (Smith et al., 2014).
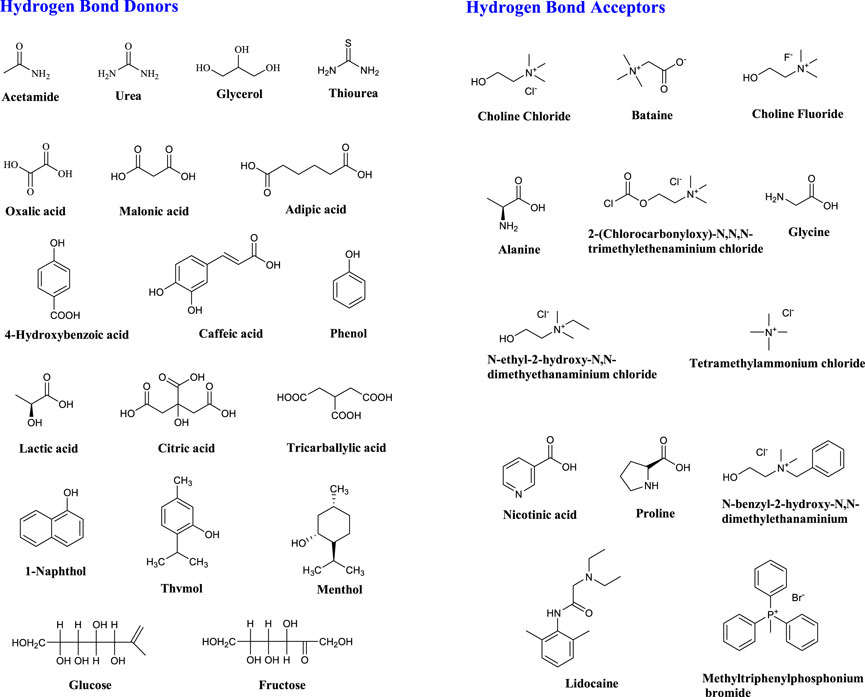
FIGURE 2. Typical structures of hydrogen bond donors (HBDs) and bond acceptors (HBAs) for DES synthesis.

TABLE 1. The general formula for the classification of DES. Reprinted from ref. (Smith et al., 2014). Copyright 2014 American Chemical Society.
Type II DES employs hydrated metal halides, which are less expensive and less susceptible to atmospheric moisture than type I metal salts, making them popular in the industry (Smith et al., 2014). The most widely used DES is type III DES, which has the advantages of simple and low-cost preparation (Smith et al., 2014), being environmentally friendly and mostly biodegradable, not reacting with water, and having a high potential for lignocellulosic biomass processing, so this review will mainly focus on type III DES. In fact, in addition to the four types of DES mentioned above, type V has been developed, which is made up entirely of nonionic, molecular HBA, and HBD. These nonionic DES overcome the drawbacks of quaternary ammonium salt-based DES, such as expense, hydrophobicity, and viscosity, and exhibit the ability to recover and recycle DES by evaporation. However, the research aspect of V-type DES is not mature enough to be described here due to its late discovery (Abranches et al., 2019).
2.3 Catalytic and Solubilizing Effects of DES on Lignocellulose
2.3.1 Dissolution
Lignocellulosic biomass has a complicated structural and chemical mechanism for resistance to microbial and enzymatic deconstruction, as well as a natural resistance known as “biomass resistance,” which significantly inhibits biomass conversion into value-added products (Himmel Michael et al., 2007; Mettler et al., 2012). This “biomass resistance” is considered to arise mainly from lignin and lignin-carbohydrate complexes, which are cross-linked to carbohydrates (especially hemicellulose) through a network of strong covalent and hydrogen bonds with benzyl esters, benzyl ethers, and phenyl glycosides functional groups (Liu et al., 2017). The crosslinking degree of the lignin-carbohydrate complex is associated with increased cell wall stiffness and resistance to enzymatic digestion. To achieve an efficient deconstruction process, these crosslinks must be destroyed by the chemical hydrolysis of ester bonds (Grabber et al., 1998; McCann and Carpita, 2015). Strong hydrogen bonding ligands in ionic liquids (ILs) preferentially solubilize lignin and hemicellulose in biomass while maintaining biopolymer integrity (Carrozza et al., 2019). Inspired by the high solubilization capacity of ILs for lignocellulose, DES has attracted increasing interest as an inexpensive alternative to ILs. DES can deliver and accept protons, which enables the formation of hydrogen bonds with other compounds, thus enhancing their solubilization properties (Pandey et al., 2017). Acidic DES (HBD: lactic, malic, and oxalic acids) are very effective in lignin solubilization (Xu et al., 2016; Liu et al., 2017; Yiin et al., 2017; Liu et al., 2019; Ramesh et al., 2020; Yiin et al., 2021). One of the reasons why DES selectively dissolves lignin over cellulose is that both cellulose and DES have strong hydrogen bonding networks, solubilizing cellulose in DES requires dissociation and reorganization of both hydrogen-bonding networks to generate a thermodynamically more stable network. However, the cohesive energy of cellulose is so strong that it may prevent its solubilization in any DES (Vigier et al., 2015). As shown in Figure 3, chloride ions present in DES can establish hydrogen bonds with hydroxyl groups that are present in carbohydrates and lignin, disrupting the carbohydrate-lignin bonding connections and hydrolyzing the LCC to remove hemicellulose while also enhancing enzyme accessibility and hydrolysis yields (Satlewal et al., 2018; Lee and Wu, 2021). DES can effectively dissolve the lignin and remove amorphous cellulose from lignocellulose. As a result, DES can fractionate lignocellulose to promote hemicellulose and lignin utilization while also improving cellulose crystallinity.
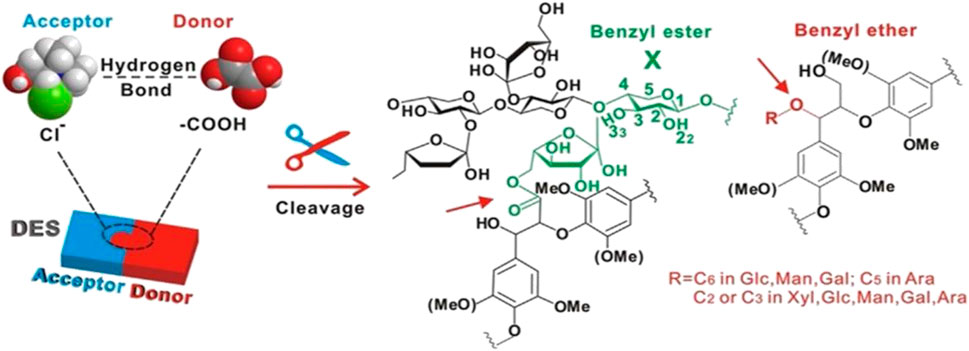
FIGURE 3. Disruption of lignin carbohydrate complexes with DES. Reprinted from ref. (Satlewal et al., 2018). Copyright 2018 Elsevier Inc.
2.3.2 Catalysis
DES formed by combining ChCl and carboxylic acids is commonly used in the production of furan compounds. ChCl-based DES has been found to be effective for xylan solubilization in biomass pretreatment processes in recent studies (Chen et al., 2018b; Chen et al., 2018c; Shen et al., 2019). These DES based on ChCl-carboxylic acid can act as both solvents and catalysts. After lignin removal with the help of DES, hemicellulose, and cellulose were converted to furfural and hydroxymethylfurfural, respectively (Parsell et al., 2015). Hemicellulose is more readily depolymerized than cellulose (especially under acidic conditions) due to its non-crystalline nature (Brandt et al., 2013). After disruption of the hemicellulose polymer by chloride ions in DES, the carboxylic acids can provide the required protons to break down the sugar-1,4 glycosidic bonds in xylan to produce xylose, which can then be dehydrated to furfural (Enslow and Bell, 2012; Li et al., 2019). Unlike hemicellulose, the quantity and strength of hydrogen bonds established with cellulose determine cellulose’s dissolution (Lynam et al., 2017). To increase the output of furans, novel DES that can concurrently catalyze hemicellulose and cellulose must be discovered.
3 Catalytic Conversion of Biomass to Furfural in Deep Eutectic Solvents
The conversion of carbohydrates to furans is a hot research topic in the biorefining of lignocellulose (Li et al., 2016; Peleteiro et al., 2016; Fan et al., 2018). The acid-catalyzed hydrolysis and dehydration of pentosans in lignocellulose produces furfural. Two methods are generally used to produce furfural in the DES system. In the “two-step method” in which the lignin carbohydrate complex is destroyed by pretreatment, the hemicellulose-derived sugar is selectively dissolved from the biomass, and then the acidified aqueous DES solution is used to produce furfural from the xylose-rich pretreatment solution (Arora et al., 2021), and the “one-step method” in which the acidified DES is used to produce furfural by directly treating pentosan or biomass (Lee et al., 2019; Rusanen et al., 2021). The reaction can be further enhanced to promote furfural synthesis if Brønsted acid or Lewis acid catalyst is added to the DES system to some extent (Wang et al., 2018; Chen and Wan, 2019). Recent progress in the addition of various catalysts to DES systems for furfural production is shown in the chart.
3.1 Catalysis in Neat DES System
Furfural is produced by dehydration of xylose in an acidic medium (Enslow and Bell, 2012). HBD for various acidic complexing agents such as oxalic acid, citric acid, p-toluene sulfonic acid, trichloroacetic acid, and other acidic complexing agents with ionizable protons is named Brønsted acidic deep eutectic solvents (BADES) in acidic DES (ADES) (Qin et al., 2020). The acidic HBD can catalyze the hydrolysis of hemicellulose to form xylose or arabinose, which is further dehydrated to produce furfural by releasing three water molecules (Marcotullio and De Jong, 2010; Cai et al., 2014). The acidic strength of the acid catalyst/solvent is well known to play a critical role in the hydrolysis of hemicellulose (Choudhary et al., 2012; Wang et al., 2015; Delbecq et al., 2018), and it is thus critical to optimize and mediate ADES acidity (Kore and Srivastava, 2011). The higher the pH grew closer to 1, the larger the productivity of furfural, according to Arora et al. (2021). The yield of furfural was 85.4% after treatment of hemicellulose with ChCl: p-toluene sulfonic acid (p-TSA) = 1:1 and pH = 1 at 120°C for 1.5 h (Table 2, entry 1). When the pH was increased from 1 to 3, the yield of furfural reduced to 51.4% (Table 2, entry 2) (Arora et al., 2021). The effect of acidity on the yield of furfural was also confirmed by Cornelius et al. when comparing choline chloride-dicarboxylate-based low eutectic solvents. The increasing carbon chain length of dicarboxylic acid HBD boosts the electron feeding effect of alkyl groups, reducing the strength of the hydrogen bond formed between HBA and HBD as well as the hydrogen feeding capacity of DES, according to the reaction mechanism. Finally, because the concentration of H+ in the dehydration and hydrolysis reaction system is lowered, which is detrimental to furfural production, DES (oxalic acid) with short carbon chain dicarboxylic acid gives greater furfural yields (Lee et al., 2019). Lurii and others used ChCl/OA at 100°C to convert certain underappreciated terrestrial and marine biomass into furfural, yielding up to 72% (Table 2, entries 3–6), further demonstrating the superiority of oxalic acid as an HBD (Bodachivskyi et al., 2019). Although larger yields of furfural can be achieved without the use of a catalyst in a neat DES system, DES has drawbacks such as higher viscosity that cannot be overlooked. Although neat DES has absolute advantages for compounds with low water solubility, and better yields of furfural can be achieved in this system, practical issues such as time-consuming solvent transfer and inefficient mass transfer due to DES drawbacks such as higher viscosity cannot be neglected. Exploring some novel DES with low viscosity, high acidity, and good thermal stability is the main research topic in the future.
3.2 Catalyst in Deep Eutectic Solvents System
3.2.1 Water
In biomass, water contributes to hemicellulose saccharification and xylose recovery. The addition of water in the DES system also reduces the viscosity of the DES (Dai et al., 2015), allowing for better DES penetration into the lignocellulosic matrix (New et al., 2019), improved mass transfer, and increased delignification, all of which aid hemicellulose extraction and subsequent furfural production (Loow et al., 2017). As a result, one of the most important influencing factors for hemicellulose conversion is the amount of water in the system. Annu et al. investigated the effect of different water contents on the yield of furfural in a deep eutectic solvent system. In the MIBK/DES biphasic system, higher furfural yields of 62% and 37.5% (Table 3, entry 1–2) were obtained for the sugar mixture and birch sawdust, respectively, when 32.9 wt% water was added to the microwave reactor (Rusanen et al., 2021). Water can promote furfural production, but too much water in the system can be harmful to getting enough furfural. When the amount of water injected exceeded 40 wt%, the yield of furfural decreased significantly (Rusanen et al., 2021). Excess water breaks the hydrogen bond between HBD and HBA and also promotes the formation of humins, thus obtaining a low furfural yield (Hammond et al., 2017; Gabriele et al., 2019). When comparing the yield of furfural before and after the addition of water, direct treatment of oil palm leaves with ChCl/OA aqueous solution (16.4 wt% H2O) at 100°C after the addition of 2 ml of water enhanced the production of furfural from 9.74% to 26.3%. (Table 3, entry 3) (Lee et al., 2019). A biphasic system was created using a ChCl: EG aqueous solution (30 percent water) combined with acetone (2:7), and xylose was reacted at 180°C for 30 min to get a high furfural yield of 75.6% (Table 3, entry 4) (Chen and Wan, 2019). The hydrogen bond between HBD and HBA can be weakened by the right amount of water employed in DES, making them more accessible and reactive. A deep eutectic solvent treatment with choline chloride-oxalic acid aqueous solution (16.4 wt% H2O) was added following ultrasonication of oil palm leaves to disrupt the LCC structure, yielding a furfural yield of 56.5% (Table 3, entry 5) (Lee et al., 2021). Unlike other similar studies, they used ultrasonic lignocellulose pretreatment. This technique produces acetic acid, which can efficiently increase furfural conversion while reducing reaction time (Lee et al., 2021). In the biphasic system, xylan was used as a starting material and 5 wt% water was added to ChCl/malic acid, then the system was heated by microwave at 150°C for 2.5 min to obtain a high yield of furfural (75%) (Table 3, entry 6) (Morais et al., 2020). This set of experiments was the shortest reaction time among these groups of similar experiments, and high yields of furfural were obtained. When compared to the traditional hydrothermal approach, either ultrasonic pretreatment or microwave heating can employ a shorter reaction time to get higher yields but raises the cost of industrial applications. The appropriate amount of water facilitates the production of furfural, but when the amount of water exceeds a certain threshold, the internal structure of DES is destroyed, and the interaction between DES and biomass is hampered, resulting in a reduction in furfural yield. Water mitigated several intrinsic flaws of DES and enhanced furfural yield, but this was limited. Compared to the reaction systems with the addition of Lewis acid, the furfural yield is lower when only DES acted as Brønsted acid without the presence of Lewis acid in the reaction because Lewis acid may catalyze the isomerization of xylose to xylulose, which is then further dehydrated to generate furfural. Finding an appropriate Lewis acid catalyst as well as deep eutectic solvents that are water-resistant is a pressing issue that must be addressed.
3.2.2 Metal Chlorides
Metal chlorides have been widely used as catalysts in the synthesis of furfural from lignocellulose (Chen et al., 2018b; Chen and Wan, 2019; Yu et al., 2019b). Metal chloride increases furfural yield due to the action of metal cations and Cl− (Gravitis et al., 2001; Marcotullio and De Jong, 2010). For example, using ChCl/citric acid aqueous solution as the solvent and adding AlCl3·6H2O catalyst, 73.1% furfural yield (Table 4, entry 1) can be obtained from xylose and 68.6% (Table 4, entry 2) from xylan (Zhang and Yu, 2013). On aldose or aldose polymers, metal chlorides have an excellent isomerization impact. The addition of metal chloride to DES produced Lewis and Brønsted acid reaction medium. The metal chloride promoted the enolization of xylose and improved the selectivity and yield of furfural (Marcotullio and de Jong, 2011), and it catalyzes the dehydration of xylose to form furfural, through xylose isomerization to xylulose (Binder et al., 2010; vom Stein et al., 2011; Yang et al., 2012). When the biphasic system is applied in combination with the catalyst, excellent yields of furfural can be obtained. A high furfural yield of 70.3% (Table 4, entry 3) could be obtained by adding a specified amount of metal chloride as a catalyst to the DES (ChCl/OA)/MIBK biphasic system to react with eucalyptus (Wang et al., 2018). Zhang et al. studied the conversion of xylose to furfural by trivalent metal chlorides in ChCl-oxalic acid at 100°C, with the greatest furfural yields of 60.4% and 55.5% (Table 4, entries 4, 6) from xylose and xylan, respectively. AlCl3·6H2O was demonstrated to be the most efficient in generating furfural from xylose (Zhang et al., 2014). However, the thermal stability of ChCl/oxalic acid is poor at temperatures above 379 K (Zhang et al., 2014). A comparative investigation by Qiang et al. found that adding SnCl4·5H2O (Table 4, entries 7–10) to the ChCl/formic (Fa) system was the most successful (Yu et al., 2019a). Because the metal cation catalyzed the conversion of carbohydrates to furfural is related to its ionization potential, the furfural yields produced with different metal chlorides can vary. Although metal chlorides have good results for furfural yield, certain drawbacks should not be ignored, such as difficulty in separation and recovery, instability, and toxicity. Alkali metal salt catalysts have a limited function in furfural production, and combining alkali metal salts with acid catalysts in deep eutectic solvents improves furfural production. Metal chlorides dissolved in DES are difficult to be recovered for reuse, which is contrary to the principle of green chemistry. In industrial applications, new extractants are needed to separate metal chlorides from DES, or catalysts that are insoluble in DES are required.
3.2.3 Alkali Metal Halides
Alkali metal halides have been shown to increase the yield of furfural when added to solvent systems (water or non-polar solvents) (Enslow and Bell, 2015; Mellmer et al., 2019), mainly in biphasic systems to afford the “salting out” effect and thus increase the strong extraction of furfural from the aqueous phase into the organic phase. Similarly, they play a crucial role in the kinetics of xylose dehydration in acidic media. Eduarda and coworkers compared the differences of eight alkali metal salts added to DES to increase furfural yield: sodium chloride (NaCl), sodium bromide (NaBr), sodium iodide (NaI), potassium chloride (KCl), potassium bromide (KBr), potassium iodide (KI), lithium chloride (LiCl) and lithium bromide (LiBr). In comparison to the unadded halide salts, NaBr, NaI, KI, and LiBr exhibited improvement in furfural yield to a certain extent (Morais et al., 2021). The carbon cation is generated after the loss of water from xylose in the dehydration process of xylose to furfural, which is stabilized by the presence of halides. When a metal cation with significant hydrophilic characteristics interacts with the negatively charged xylose, it prevents the carbon cation from rehydration, stabilizing the generated carbon cation (Enslow and Bell, 2015). The carbon cation is a reactive intermediate that can undergo rearrangement to form 2,5-no-hydroxy sugars, which are then further dehydrated to produce furfural (Enslow and Bell, 2015). The halide anion serves as a stabilizing agent interacting with critical intermediate in the DES system, whereas the cation largely interacts with the chloride anion, and these interactions then influence xylose solvation and dehydration. The yield of furfural was then further optimized, and it was found that adding LiBr to ChCl/MA (5 wt% H2O) at 175.3°C for 1.74 min resulted in a high furfural yield of 89.5% (Table 4, entry 1) (Morais et al., 2021). Alkali metal halides can boost the yield of furfural by increasing the salting effect of the biphasic system, but in the complex lignocellulose structure, alkali metal salts cannot cooperate with DES to accelerate the isomerization of xylose and thus decrease the yield of furfural more efficiently. Alkali metal salt catalysts have a limited function in furfural production, and combining alkali metal salts with acid catalysts in DES improves furfural production.
3.2.4 Solid Acid Catalysts
To avoid the potential corrosiveness and high energy cost of homogeneous catalysis (Li Y.-Y. et al., 2021), many solid acids have been used in furfural production which provides high furfural yields. A new heterogeneous catalyst B: LA-SG (SiO2) was prepared by immobilizing the deep eutectic solvent betaine/lactate on silica using the original tetraethyl silicate as the silica source by the sol-gel method. High furfural yields (45.3%) were obtained from corn cobs using the B: LA-SG (SiO2) catalyst (2.5 wt%) in water at 170°C for 0.5 h (Table 5, entry 2) (Li et al., 2022). However, the catalyst did not have an excellent catalytic performance in the production of furfural, owing to a lack of acidic sites in the catalyst. Lewis and Brønsted acid sites can isomerize aldose-ketose and accelerate furfural formation via ring dehydration (Li et al., 2022). Li et al. creatively used spent shrimp shells as a starting material to prepare sulfonated tin-based solid acids (Sn-SS) for the conversion of sugarcane bagasse (SB) to furfural in DES (ChCl/EG)-water at 170°C for 20 min, yielding 62.3% furfural (Table 5, entry 4) (Li et al., 2021a). The hydrogen bonds in SB were broken by ChCl/EG, and the Sn-SS solid acid could pretreat and depolymerize SB. Sn-SS contains Lewis and Brønsted acid sites, which can catalyze biomass into furfural. Unlike the previous study, Ji et al. directly used maize stover (CS) as a support to prepare the SO42-/SnO2-CS catalysts for the production of furfural in ChCl: EG-water (20:80, v: v) at 170°C for 0.5 h, and a high furfural yield of up to 61.8% (Table 5, entry 5) was obtained (Ji et al., 2021). Compared with some traditional metal-based solid acids, biomass-based solid acids have the advantages of being less expensive, unique in structure, environmentally friendly, and easy preparation. The spongy and squishy shape of CS allows it to immobilize additional functional groups on its surface or inside it (Jiang et al., 2020). During the synthesis of furfural, chlorides can stabilize the transition state and intermediate structures, and reduce the occurrence of undesired side reactions (Figure 4) (Campos Molina et al., 2012; Ji et al., 2021). For instance, MgCl2 was added to the reaction system to improve the furfural yield up to 68.2% (Table 5, entry 6) (Ji et al., 2021). Both research groups use waste biomass as a source of catalysts, which is in keeping with the concept of sustainable and green chemistry. Solid catalysts, on the other hand, have difficulty recovering due to the higher viscosity of DES or insoluble by-products such as humins compounds clinging to them. Solid acid catalysts can flexibly tune the number of acidic sites to efficiently boost furfural yield, but the fabrication procedure is complicated and the catalyst stability is not very good, posing an economic barrier for practical application on a large scale. Reactions incorporating solid catalysts often require high energy, and most DES are thermally unstable and do not function optimally at high temperatures. Future research will focus on the development of novel highly efficient solid acid catalysts that are both inexpensive, robust, and facile to prepare, as well as deep eutectic solvents with improved thermal stability.
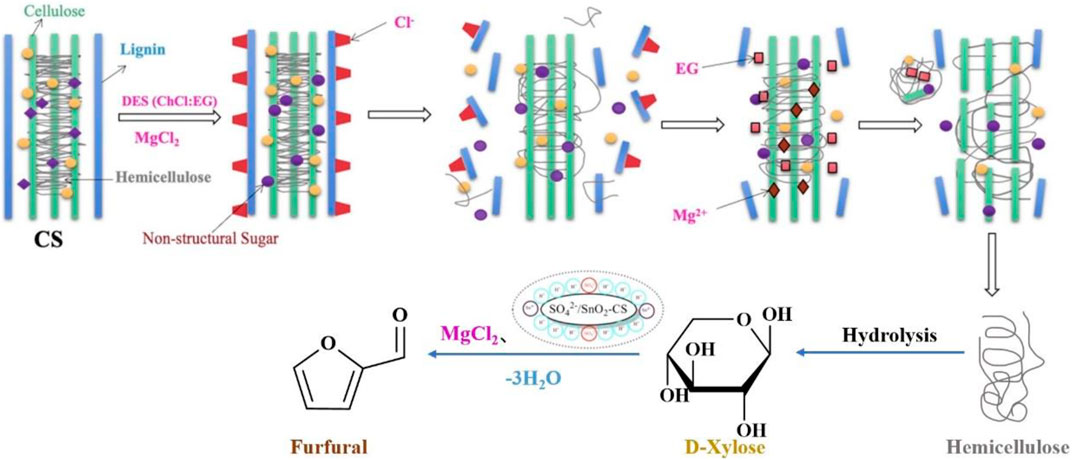
FIGURE 4. The catalytic mechanism for catalyzing corn stover into furfural and byproducts at 170°C for 0.5 h in ChCl: EG–water (20:80, v: v). Reprinted from ref. (Ji et al., 2021). Copyright 2021 Elsevier Ltd.
3.2.5 Other Catalysts
Other additions, such as oxides, organic acids, and heterogeneous catalysts, can boost furfural yields in addition to the foregoing catalysts. The addition of HCOOH has been shown to give a high furfural yield (58.3%) from biomass-derived xylose in the ChCl: Gly-water system within 30 min at 180°C (Table 5, entry 7), compared to the reaction system without the addition of catalyst (Li et al., 2021b). The simplest organic acid, HCOOH, can be employed as a dehydration catalyst in the production of furfural, overcoming the main disadvantage of corrosive inorganic acid catalysts to some extent. The addition of oxides can also improve the furfural yield, Viviane et al. mixed ChCl/OA, sulfolane, substrate, TiO2, and water to catalyze lignocellulosic materials and obtained the greatest yield of 53.2% for HMF + furfural (Table 5, entry 8) (da Silva Lacerda et al., 2015).
4 Conclusion and Perspective
This review summarizes the most recent progress on the conversion of biomass to furfural using various catalysts in DES systems. Unlike previous aqueous and organic systems, which required high temperatures and had low furfural yields, the DES/biphasic systems (DES/organic solvent) can achieve high furfural yield at lower temperatures and avoid the formation of humins by subsequent side reactions due to high furfural concentration. The ability to create furans at higher substrate concentrations and superior lignocellulose separation are two advantages of deep eutectic solvents. The addition of an appropriate catalyst promotes the dehydration of xylose/xylan into furfural with higher yields. At present, the manufacture of high-yield furfural utilizing a deep eutectic solvent is still in its initial stages of development, with numerous challenges and innovation constraints.
(1) When employing an organic phase and a deep eutectic solvent for a biphasic system, large yields can only be achieved in the laboratory, but it is very difficult for industrial applications. The extraction efficiency of organic solvents is high, but recovery requires energy-intensive devices and most organic solvents are toxic.
(2) It is necessary and cumbersome to firstly transform hemicellulose of lignocellulose into xylan with high yields because lignocellulose has recalcitrant in order to obtain high furfural yield, which results in the loss of some hemicellulose.
(3) Recycling deep eutectic solvents is a more energy-efficient and environmentally benign solution from the standpoint of sustainable development. On the other hand, deep eutectic solvents are polluted and cannot be reused due to their high viscosity and the fact that a portion of the catalyst will stay in DES.
(4) Some deep eutectic solvents have poor thermal stability, while some solid catalysts (e.g., Nb2O5) can only function at higher temperatures, resulting in a suboptimal reaction.
Recently, it has been discovered that there exists hydrophobic DES, which can extract furfural from water efficiently and can replace the role of organic solvent in the DES/biphasic system, thus alleviating the high energy consumption due to organic solvent. It is urgent to develop novel deep eutectic solvents and to explore a sustainable and more cost-effective biorefinery method for producing furfural from lignocellulosic biomass.
Author Contributions
XZ: Conceptualization, formal analysis, investigation, resources, writing original draft, visualization. PZ: Writing review and editing. QL: Investigation, formal analysis. HX: Writing review and editing, funding acquisition.
Conflict of Interest
The authors declare that the research was conducted in the absence of any commercial or financial relationships that could be construed as a potential conflict of interest.
Publisher’s Note
All claims expressed in this article are solely those of the authors and do not necessarily represent those of their affiliated organizations, or those of the publisher, the editors and the reviewers. Any product that may be evaluated in this article, or claim that may be made by its manufacturer, is not guaranteed or endorsed by the publisher.
Supplementary Material
The Supplementary Material for this article can be found online at: https://www.frontiersin.org/articles/10.3389/fchem.2022.911674/full#supplementary-material
References
Abbott, A. P., Boothby, D., Capper, G., Davies, D. L., and Rasheed, R. K. (2004). Deep Eutectic Solvents Formed between Choline Chloride and Carboxylic Acids: Versatile Alternatives to Ionic Liquids. J. Am. Chem. Soc. 126 (29), 9142–9147. doi:10.1021/ja048266j
Abbott, A. P., Capper, G., Davies, D. L., McKenzie, K. J., and Obi, S. U. (2006). Solubility of Metal Oxides in Deep Eutectic Solvents Based on Choline Chloride. J. Chem. Eng. Data 51 (4), 1280–1282. doi:10.1021/je060038c
Abbott, A. P., Capper, G., Davies, D. L., Munro, H. L., Rasheed, R. K., and Tambyrajah, V. (2001). Preparation of Novel, Moisture-Stable, Lewis-acidic Ionic Liquids Containing Quaternary Ammonium Salts with Functional Side Chains. Chem. Commun. 19, 2010–2011. doi:10.1039/b106357j
Abbott, A. P., Capper, G., Davies, D. L., Rasheed, R. K., and Shikotra, P. (2005). Selective Extraction of Metals from Mixed Oxide Matrixes Using Choline-Based Ionic Liquids. Inorg. Chem. 44 (19), 6497–6499. doi:10.1021/ic0505450
Abbott, A. P., Capper, G., Davies, D. L., Rasheed, R. K., and Tambyrajah, V. (2003). Novel Solvent Properties of Choline Chloride/urea mixturesElectronic Supplementary Information (ESI) Available: Spectroscopic Data. See http://www.rsc.Org/suppdata/cc/b2/b210714g/. Chem. Commun. 1, 70–71. doi:10.1039/b210714g
Abbott, A. P., Capper, G., McKenzie, K. J., and Ryder, K. S. (2007). Electrodeposition of Zinc-Tin Alloys from Deep Eutectic Solvents Based on Choline Chloride. J. Electroanal. Chem. 599 (2), 288–294. doi:10.1016/j.jelechem.2006.04.024
Abranches, D. O., Martins, M. A. R., Silva, L. P., Schaeffer, N., Pinho, S. P., and Coutinho, J. A. P. (2019). Phenolic Hydrogen Bond Donors in the Formation of Non-ionic Deep Eutectic Solvents: the Quest for Type V DES. Chem. Commun. 55 (69), 10253–10256. doi:10.1039/c9cc04846d
Arora, S., Gupta, N., and Singh, V. (2021). pH‐Controlled Efficient Conversion of Hemicellulose to Furfural Using Choline‐Based Deep Eutectic Solvents as Catalysts. ChemSusChem 14 (18), 3953–3958. doi:10.1002/cssc.202101130
Binder, J. B., Blank, J. J., Cefali, A. V., and Raines, R. T. (2010). Synthesis of Furfural from Xylose and Xylan. ChemSusChem 3 (11), 1268–1272. doi:10.1002/cssc.201000181
Bodachivskyi, I., Kuzhiumparambil, U., and Williams, D. B. G. (2019). Catalytic Valorization of Native Biomass in a Deep Eutectic Solvent: A Systematic Approach toward High-Yielding Reactions of Polysaccharides. ACS Sustain. Chem. Eng. 8 (1), 678–685. doi:10.1021/acssuschemeng.9b06528
Bozell, J. J., and Petersen, G. R. (2010). Technology Development for the Production of Biobased Products from Biorefinery Carbohydrates-The US Department of Energy's "Top 10" Revisited. Green Chem. 12 (4), 539. doi:10.1039/b922014c
Brandt, A., Gräsvik, J., Hallett, J. P., and Welton, T. (2013). Deconstruction of Lignocellulosic Biomass with Ionic Liquids. Green Chem. 15 (3), 550. doi:10.1039/c2gc36364j
Cai, C. M., Zhang, T., Kumar, R., and Wyman, C. E. (2014). Integrated Furfural Production as a Renewable Fuel and Chemical Platform from Lignocellulosic Biomass. J. Chem. Technol. Biotechnol. 89 (1), 2–10. doi:10.1002/jctb.4168
Cai, T., and Qiu, H. (2019). Application of Deep Eutectic Solvents in Chromatography: A Review. TrAC Trends Anal. Chem. 120, 115623. doi:10.1016/j.trac.2019.115623
Campos Molina, M. J., Mariscal, R., Ojeda, M., and López Granados, M. (2012). Cyclopentyl Methyl Ether: a Green Co-solvent for the Selective Dehydration of Lignocellulosic Pentoses to Furfural. Bioresour. Technol. 126, 321–327. doi:10.1016/j.biortech.2012.09.049
Carrozza, C. F., Papa, G., Citterio, A., Sebastiano, R., Simmons, B. A., and Singh, S. (2019). One-pot Bio-Derived Ionic Liquid Conversion Followed by Hydrogenolysis Reaction for Biomass Valorization: A Promising Approach Affecting the Morphology and Quality of Lignin of Switchgrass and Poplar. Bioresour. Technol. 294, 122214. doi:10.1016/j.biortech.2019.122214
Chen, Y.-L., Zhang, X., You, T.-T., and Xu, F. (2018a). Deep Eutectic Solvents (DESs) for Cellulose Dissolution: a Mini-Review. Cellulose 26 (1), 205–213. doi:10.1007/s10570-018-2130-7
Chen, Z., Bai, X., and A, C. (2018b). High-Solid Lignocellulose Processing Enabled by Natural Deep Eutectic Solvent for Lignin Extraction and Industrially Relevant Production of Renewable Chemicals. ACS Sustain. Chem. Eng. 6 (9), 12205–12216. doi:10.1021/acssuschemeng.8b02541
Chen, Z., Reznicek, W. D., and Wan, C. (2018c). Aqueous Choline Chloride: A Novel Solvent for Switchgrass Fractionation and Subsequent Hemicellulose Conversion into Furfural. ACS Sustain. Chem. Eng. 6 (5), 6910–6919. doi:10.1021/acssuschemeng.8b00728
Chen, Z., and Wan, C. (2019). A Novel Deep Eutectic Solvent/acetone Biphasic System for High-Yield Furfural Production. Bioresour. Technol. Rep. 8, 100318. doi:10.1016/j.biteb.2019.100318
Choudhary, V., Sandler, S. I., and Vlachos, D. G. (2012). Conversion of Xylose to Furfural Using Lewis and Brønsted Acid Catalysts in Aqueous Media. ACS Catal. 2 (9), 2022–2028. doi:10.1021/cs300265d
Cunha, S. C., and Fernandes, J. O. (2018). Extraction Techniques with Deep Eutectic Solvents. TrAC Trends Anal. Chem. 105, 225–239. doi:10.1016/j.trac.2018.05.001
da Silva Lacerda, V., López-Sotelo, J. B., Correa-Guimarães, A., Hernández-Navarro, S., Sánchez-Bascones, M., Navas-Gracia, L. M., et al. (2015). A Kinetic Study on Microwave-Assisted Conversion of Cellulose and Lignocellulosic Waste into Hydroxymethylfurfural/furfural. Bioresour. Technol. 180, 88–96. doi:10.1016/j.biortech.2014.12.089
Dai, Y., van Spronsen, J., Witkamp, G.-J., Verpoorte, R., and Choi, Y. H. (2013). Natural Deep Eutectic Solvents as New Potential Media for Green Technology. Anal. Chim. Acta 766, 61–68. doi:10.1016/j.aca.2012.12.019
Dai, Y., Witkamp, G.-J., Verpoorte, R., and Choi, Y. H. (2015). Tailoring Properties of Natural Deep Eutectic Solvents with Water to Facilitate Their Applications. Food Chem. 187, 14–19. doi:10.1016/j.foodchem.2015.03.123
Delbecq, F., Wang, Y., Muralidhara, A., El Ouardi, K., Marlair, G., and Len, C. (2018). Hydrolysis of Hemicellulose and Derivatives-A Review of Recent Advances in the Production of Furfural. Front. Chem. 6, 146. doi:10.3389/fchem.2018.00146
Delgado-Mellado, N., Larriba, M., Navarro, P., Rigual, V., Ayuso, M., García, J., et al. (2018). Thermal Stability of Choline Chloride Deep Eutectic Solvents by TGA/FTIR-ATR Analysis. J. Mol. Liq. 260, 37–43. doi:10.1016/j.molliq.2018.03.076
Enslow, K. R., and Bell, A. T. (2012). The Kinetics of Brønsted Acid-Catalyzed Hydrolysis of Hemicellulose Dissolved in 1-Ethyl-3-Methylimidazolium Chloride. RSC Adv. 2 (26), 10028. doi:10.1039/c2ra21650g
Enslow, K. R., and Bell, A. T. (2015). The Role of Metal Halides in Enhancing the Dehydration of Xylose to Furfural. ChemCatChem 7 (3), 479–489. doi:10.1002/cctc.201402842
Fan, G., Wang, Y., Hu, Z., Yan, J., Li, J., and Song, G. (2018). Synthesis of 5-hydroxymethyl Furfural from Cellulose via a Two-step Process in Polar Aprotic Solvent. Carbohydr. Polym. 200, 529–535. doi:10.1016/j.carbpol.2018.08.043
Florindo, C., Oliveira, F. S., Rebelo, L. P. N., Fernandes, A. M., and Marrucho, I. M. (2014). Insights into the Synthesis and Properties of Deep Eutectic Solvents Based on Cholinium Chloride and Carboxylic Acids. ACS Sustain. Chem. Eng. 2 (10), 2416–2425. doi:10.1021/sc500439w
Francisco, M., van den Bruinhorst, A., and Kroon, M. C. (2012). New Natural and Renewable Low Transition Temperature Mixtures (LTTMs): Screening as Solvents for Lignocellulosic Biomass Processing. Green Chem. 14 (8), 2153. doi:10.1039/c2gc35660k
Francisco, M., van den Bruinhorst, A., and Kroon, M. C. (2013). Low-transition-temperature Mixtures (LTTMs): a New Generation of Designer Solvents. Angew. Chem. Int. Ed. 52 (11), 3074–3085. doi:10.1002/anie.201207548
Gabriele, F., Chiarini, M., Germani, R., Tiecco, M., and Spreti, N. (2019). Effect of Water Addition on Choline Chloride/glycol Deep Eutectic Solvents: Characterization of Their Structural and Physicochemical Properties. J. Mol. Liq. 291, 111301. doi:10.1016/j.molliq.2019.111301
Grabber, J. H., Hatfield, R. D., and Ralph, J. (1998). Diferulate Cross-Links Impede the Enzymatic Degradation of Non-lignified Maize Walls. J. Sci. Food Agric. 77 (2), 193–200. doi:10.1002/(sici)1097-0010(199806)77:2<193::aid-jsfa25>3.0.co;2-a
Gravitis, J., Vedernikov, N., Zandersons, J., and Kokorevics, A. (2001). Furfural and Levoglucosan Production from Deciduous Wood and Agricultural Wastes. Chem. Mater. Renew. Resour., 110–122. doi:10.1021/bk-2001-0784.ch009
Gutiérrez, M. C., Ferrer, M. L., Mateo, C. R., and del Monte, F. (2009). Freeze-drying of Aqueous Solutions of Deep Eutectic Solvents: a Suitable Approach to Deep Eutectic Suspensions of Self-Assembled Structures. Langmuir 25 (10), 5509–5515. doi:10.1021/la900552b
Hammond, O. S., Bowron, D. T., and Edler, K. J. (2016). Liquid Structure of the Choline Chloride-Urea Deep Eutectic Solvent (Reline) from Neutron Diffraction and Atomistic Modelling. Green Chem. 18 (9), 2736–2744. doi:10.1039/c5gc02914g
Hammond, O. S., Bowron, D. T., and Edler, K. J. (2017). The Effect of Water upon Deep Eutectic Solvent Nanostructure: An Unusual Transition from Ionic Mixture to Aqueous Solution. Angew. Chem. Int. Ed. 56 (33), 9782–9785. doi:10.1002/anie.201702486
Hansen, B. B., Spittle, S., Chen, B., Poe, D., Zhang, Y., Klein, J. M., et al. (2021). Deep Eutectic Solvents: A Review of Fundamentals and Applications. Chem. Rev. 121 (3), 1232–1285. doi:10.1021/acs.chemrev.0c00385
Hashemi, B., Zohrabi, P., and Dehdashtian, S. (2018). Application of Green Solvents as Sorbent Modifiers in Sorptive-Based Extraction Techniques for Extraction of Environmental Pollutants. TrAC Trends Anal. Chem. 109, 50–61. doi:10.1016/j.trac.2018.09.026
Himmel, M. E., Ding, S.-Y., Johnson, D. K., Adney, W. S., Nimlos, M. R., Brady, J. W., et al. (2007). Biomass Recalcitrance: Engineering Plants and Enzymes for Biofuels Production. Science 315 (5813), 804–807. doi:10.1126/science.1137016
Jablonský, M., Škulcová, A., Kamenská, L., Vrška, M., and Šíma, J. (2015). Deep Eutectic Solvents: Fractionation of Wheat Straw. BioResources 10 (4), 8047. doi:10.15376/biores.10.4.8039-8047
Ji, L., Tang, Z., Yang, D., Ma, C., and He, Y.-C. (2021). Improved One-Pot Synthesis of Furfural from Corn Stalk with Heterogeneous Catalysis Using Corn Stalk as Biobased Carrier in Deep Eutectic Solvent-Water System. Bioresour. Technol. 340, 125691. doi:10.1016/j.biortech.2021.125691
Jiang, Q., Yang, G., Kong, F., Fatehi, P., and Wang, X. (2020). High Acid Biochar-Based Solid Acid Catalyst from Corn Stalk for Lignin Hydrothermal Degradation. Polymers 12 (7), 1623. doi:10.3390/polym12071623
Kore, R., and Srivastava, R. (2011). Synthesis and Applications of Novel Imidazole and Benzimidazole Based Sulfonic Acid Group Functionalized Brönsted Acidic Ionic Liquid Catalysts. J. Mol. Catal. A Chem. 345 (1-2), 117–126. doi:10.1016/j.molcata.2011.06.003
Lee, C. B. T. L., and Wu, T. Y. (2021). A Review on Solvent Systems for Furfural Production from Lignocellulosic Biomass. Renew. Sustain. Energy Rev. 137, 110172. doi:10.1016/j.rser.2020.110172
Lee, C. B. T. L., Wu, T. Y., Cheng, C. K., Siow, L. F., and Chew, I. M. L. (2021). Nonsevere Furfural Production Using Ultrasonicated Oil Palm Fronds and Aqueous Choline Chloride-Oxalic Acid. Industrial Crops Prod. 166, 113397. doi:10.1016/j.indcrop.2021.113397
Lee, C. B. T. L., Wu, T. Y., Ting, C. H., Tan, J. K., Siow, L. F., Cheng, C. K., et al. (2019). One-pot Furfural Production Using Choline Chloride-Dicarboxylic Acid Based Deep Eutectic Solvents under Mild Conditions. Bioresour. Technol. 278, 486–489. doi:10.1016/j.biortech.2018.12.034
Li, C., Li, D., Zou, S., Li, Z., Yin, J., Wang, A., et al. (2013). Extraction Desulfurization Process of Fuels with Ammonium-Based Deep Eutectic Solvents. Green Chem. 15 (10), 2793. doi:10.1039/c3gc41067f
Li, H., Dai, Q., Ren, J., Jian, L., Peng, F., Sun, R., et al. (2016). Effect of Structural Characteristics of Corncob Hemicelluloses Fractionated by Graded Ethanol Precipitation on Furfural Production. Carbohydr. Polym. 136, 203–209. doi:10.1016/j.carbpol.2015.09.045
Li, Q., Di, J., Liao, X., Ni, J., Li, Q., He, Y.-C., et al. (2021a). Exploration of Benign Deep Eutectic Solvent-Water Systems for the Highly Efficient Production of Furfurylamine from Sugarcane Bagasse via Chemoenzymatic Cascade Catalysis. Green Chem. 23 (20), 8154–8168. doi:10.1039/d1gc03010h
Li, Q., Ma, C., Di, J., Ni, J., and He, Y.-C. (2022). Catalytic Valorization of Biomass for Furfuryl Alcohol by Novel Deep Eutectic Solvent-Silica Chemocatalyst and Newly Constructed Reductase Biocatalyst. Bioresour. Technol. 347, 126376. doi:10.1016/j.biortech.2021.126376
Li, Q., Ren, J.-Q., Li, Q., Di, J.-H., Ma, C., and He, Y. (2021b). Sustainable Conversion of Biomass-Derived D-Xylose to Furfuryl Alcohol in a Deep Eutectic Solvent-Water System. ACS Sustain. Chem. Eng. 9 (30), 10299–10308. doi:10.1021/acssuschemeng.1c02965
Li, X., Hou, M., Han, B., Wang, X., and Zou, L. (2008). Solubility of CO2 in a Choline Chloride + Urea Eutectic Mixture. J. Chem. Eng. Data 53 (2), 548–550. doi:10.1021/je700638u
Li, X., Yang, J., Xu, R., Lu, L., Kong, F., Liang, M., et al. (2019). Kinetic Study of Furfural Production from Eucalyptus Sawdust Using H-SAPO-34 as Solid Brønsted Acid and Lewis Acid Catalysts in Biomass-Derived Solvents. Industrial Crops Prod. 135, 196–205. doi:10.1016/j.indcrop.2019.04.047
Li, Y.-Y., Li, Q., Zhang, P.-Q., Ma, C.-L., Xu, J.-H., and He, Y.-C. (2021c). Catalytic Conversion of Corncob to Furfuryl Alcohol in Tandem Reaction with Tin-Loaded Sulfonated Zeolite and NADPH-dependent Reductase Biocatalyst. Bioresour. Technol. 320 (Pt A), 124267. doi:10.1016/j.biortech.2020.124267
Liu, Q., Yuan, T., Fu, Q.-j., Bai, Y.-y., Peng, F., and Yao, C.-l. (2019). Choline Chloride-Lactic Acid Deep Eutectic Solvent for Delignification and Nanocellulose Production of Moso Bamboo. Cellulose 26 (18), 9447–9462. doi:10.1007/s10570-019-02726-0
Liu, Y., Chen, W., Xia, Q., Guo, B., Wang, Q., Liu, S., et al. (2017). Efficient Cleavage of Lignin-Carbohydrate Complexes and Ultrafast Extraction of Lignin Oligomers from Wood Biomass by Microwave-Assisted Treatment with Deep Eutectic Solvent. ChemSusChem 10 (8), 1692–1700. doi:10.1002/cssc.201601795
Loow, Y.-L., Wu, T. Y., Lim, Y. S., Tan, K. A., Siow, L. F., Md. Jahim, J. J., et al. (2017). Improvement of Xylose Recovery from the Stalks of Oil Palm Fronds Using Inorganic Salt and Oxidative Agent. Energy Convers. Manag. 138, 248–260. doi:10.1016/j.enconman.2016.12.015
Luo, Y., Li, Z., Li, X., Liu, X., Fan, J., Clark, J. H., et al. (2019). The Production of Furfural Directly from Hemicellulose in Lignocellulosic Biomass: A Review. Catal. Today 319, 14–24. doi:10.1016/j.cattod.2018.06.042
Lynam, J. G., Kumar, N., and Wong, M. J. (2017). Deep Eutectic Solvents' Ability to Solubilize Lignin, Cellulose, and Hemicellulose; Thermal Stability; and Density. Bioresour. Technol. 238, 684–689. doi:10.1016/j.biortech.2017.04.079
Marcotullio, G., and De Jong, W. (2010). Chloride Ions Enhance Furfural Formation from D-Xylose in Dilute Aqueous Acidic Solutions. Green Chem. 12 (10), 1739. doi:10.1039/b927424c
Marcotullio, G., and de Jong, W. (2011). Furfural Formation from D-Xylose: the Use of Different Halides in Dilute Aqueous Acidic Solutions Allows for Exceptionally High Yields. Carbohydr. Res. 346 (11), 1291–1293. doi:10.1016/j.carres.2011.04.036
Mbous, Y. P., Hayyan, M., Hayyan, A., Wong, W. F., Hashim, M. A., and Looi, C. Y. (2017). Applications of Deep Eutectic Solvents in Biotechnology and Bioengineering-Promises and Challenges. Biotechnol. Adv. 35 (2), 105–134. doi:10.1016/j.biotechadv.2016.11.006
McCann, M. C., and Carpita, N. C. (2015). Biomass Recalcitrance: a Multi-Scale, Multi-Factor, and Conversion-specific Property: Fig. 1. Exbotj 66 (14), 4109–4118. doi:10.1093/jxb/erv267
Mellmer, M. A., Sanpitakseree, C., Demir, B., Ma, K., Elliott, W. A., Bai, P., et al. (2019). Effects of Chloride Ions in Acid-Catalyzed Biomass Dehydration Reactions in Polar Aprotic Solvents. Nat. Commun. 10 (1), 1132. doi:10.1038/s41467-019-09090-4
Mettler, M. S., Vlachos, D. G., and Dauenhauer, P. J. (2012). Top Ten Fundamental Challenges of Biomass Pyrolysis for Biofuels. Energy Environ. Sci. 5 (7), 7797. doi:10.1039/c2ee21679e
Mittal, A., Black, S. K., Vinzant, T. B., O’Brien, M., Tucker, M. P., and Johnson, D. K. (2017). Production of Furfural from Process-Relevant Biomass-Derived Pentoses in a Biphasic Reaction System. ACS Sustain. Chem. Eng. 5 (7), 5694–5701. doi:10.1021/acssuschemeng.7b00215
Mohd Zaid, H. F., Chong, F. K., and Abdul Mutalib, M. I. (2017). Extractive Deep Desulfurization of Diesel Using Choline Chloride-Glycerol Eutectic-Based Ionic Liquid as a Green Solvent. Fuel 192, 10–17. doi:10.1016/j.fuel.2016.11.112
Morais, E. S., Freire, M. G., Freire, C. S. R., Coutinho, J. A. P., and Silvestre, A. J. D. (2020). Enhanced Conversion of Xylan into Furfural Using Acidic Deep Eutectic Solvents with Dual Solvent and Catalyst Behavior. ChemSusChem 13 (4), 784–790. doi:10.1002/cssc.201902848
Morais, E. S., Freire, M. G., Freire, C. S. R., and Silvestre, A. J. D. (2021). Enhanced Furfural Production in Deep Eutectic Solvents Comprising Alkali Metal Halides as Additives. Molecules 26 (23), 7374. doi:10.3390/molecules26237374
Morrison, H. G., Sun, C. C., and Neervannan, S. (2009). Characterization of Thermal Behavior of Deep Eutectic Solvents and Their Potential as Drug Solubilization Vehicles. Int. J. Pharm. 378 (1-2), 136–139. doi:10.1016/j.ijpharm.2009.05.039
New, E. K., Wu, T. Y., Tien Loong Lee, C. B., Poon, Z. Y., Loow, Y.-L., Wei Foo, L. Y., et al. (2019). Potential Use of Pure and Diluted Choline Chloride-Based Deep Eutectic Solvent in Delignification of Oil Palm Fronds. Process Saf. Environ. Prot. 123, 190–198. doi:10.1016/j.psep.2018.11.015
Pandey, A., BhawnaDhingra, D., Dhingra, D., and Pandey, S. (2017). Hydrogen Bond Donor/Acceptor Cosolvent-Modified Choline Chloride-Based Deep Eutectic Solvents. J. Phys. Chem. B 121 (16), 4202–4212. doi:10.1021/acs.jpcb.7b01724
Parsell, T., Yohe, S., Degenstein, J., Jarrell, T., Klein, I., Gencer, E., et al. (2015). A Synergistic Biorefinery Based on Catalytic Conversion of Lignin Prior to Cellulose Starting from Lignocellulosic Biomass. Green Chem. 17 (3), 1492–1499. doi:10.1039/c4gc01911c
Peleteiro, S., Santos, V., Garrote, G., and Parajó, J. C. (2016). Furfural Production from Eucalyptus Wood Using an Acidic Ionic Liquid. Carbohydr. Polym. 146, 20–25. doi:10.1016/j.carbpol.2016.03.049
Perkins, S. L., Painter, P., and Colina, C. M. (2014). Experimental and Computational Studies of Choline Chloride-Based Deep Eutectic Solvents. J. Chem. Eng. Data 59 (11), 3652–3662. doi:10.1021/je500520h
Qin, H., Hu, X., Wang, J., Cheng, H., Chen, L., and Qi, Z. (2020). Overview of Acidic Deep Eutectic Solvents on Synthesis, Properties and Applications. Green Energy & Environ. 5 (1), 8–21. doi:10.1016/j.gee.2019.03.002
Ramesh, R., Nair, A., Jayavel, A., Sathiasivan, K., Rajesh, M., Ramaswamy, S., et al. (2020). Choline Chloride-Based Deep Eutectic Solvents for Efficient Delignification of Bambusa Bambos in Bio-Refinery Applications. Chem. Pap. 74 (12), 4533–4545. doi:10.1007/s11696-020-01259-2
Romo, J. E., Bollar, N. V., Zimmermann, C. J., and Wettstein, S. G. (2018). Conversion of Sugars and Biomass to Furans Using Heterogeneous Catalysts in Biphasic Solvent Systems. ChemCatChem 10 (21), 4805–4816. doi:10.1002/cctc.201800926
Rusanen, A., Lappalainen, K., Kärkkäinen, J., and Lassi, U. (2021). Furfural and 5‐Hydroxymethylfurfural Production from Sugar Mixture Using Deep Eutectic Solvent/MIBK System. ChemistryOpen 10 (10), 1004–1012. doi:10.1002/open.202100163
Satlewal, A., Agrawal, R., Bhagia, S., Sangoro, J., and Ragauskas, A. J. (2018). Natural Deep Eutectic Solvents for Lignocellulosic Biomass Pretreatment: Recent Developments, Challenges and Novel Opportunities. Biotechnol. Adv. 36 (8), 2032–2050. doi:10.1016/j.biotechadv.2018.08.009
Shen, X.-J., Wen, J.-L., Mei, Q.-Q., Chen, X., Sun, D., Yuan, T.-Q., et al. (2019). Facile Fractionation of Lignocelluloses by Biomass-Derived Deep Eutectic Solvent (DES) Pretreatment for Cellulose Enzymatic Hydrolysis and Lignin Valorization. Green Chem. 21 (2), 275–283. doi:10.1039/c8gc03064b
Smith, E. L., Abbott, A. P., and Ryder, K. S. (2014). Deep Eutectic Solvents (DESs) and Their Applications. Chem. Rev. 114 (21), 11060–11082. doi:10.1021/cr300162p
Somerville, C., Youngs, H., Taylor, C., Davis, S. C., and Long, S. P. (2010). Feedstocks for Lignocellulosic Biofuels. Science 329 (5993), 790–792. doi:10.1126/science.1189268
Song, S., Di, L., Wu, G., Dai, W., Guan, N., and Li, L. (2017). Meso-Zr-Al-beta Zeolite as a Robust Catalyst for Cascade Reactions in Biomass Valorization. Appl. Catal. B Environ. 205, 393–403. doi:10.1016/j.apcatb.2016.12.056
Song, S., Fung Kin Yuen, V., Di, L., Sun, Q., Zhou, K., and Yan, N. (2020). Integrating Biomass into the Organonitrogen Chemical Supply Chain: Production of Pyrrole and D ‐Proline from Furfural. Angew. Chem. Int. Ed. 59 (45), 19846–19850. doi:10.1002/anie.202006315
van Osch, D. J. G. P., Dietz, C. H. J. T., van Spronsen, J., Kroon, M. C., Gallucci, F., van Sint Annaland, M., et al. (2019). A Search for Natural Hydrophobic Deep Eutectic Solvents Based on Natural Components. ACS Sustain. Chem. Eng. 7 (3), 2933–2942. doi:10.1021/acssuschemeng.8b03520
Vigier, K. D. O., Chatel, G., and Jérôme, F. (2015). Contribution of Deep Eutectic Solvents for Biomass Processing: Opportunities, Challenges, and Limitations. ChemCatChem 7 (8), 1250–1260. doi:10.1002/cctc.201500134
vom Stein, T., Grande, P. M., Leitner, W., and Domínguez de María, P. (2011). Iron-catalyzed Furfural Production in Biobased Biphasic Systems: from Pure Sugars to Direct Use of Crude Xylose Effluents as Feedstock. ChemSusChem 4 (11), 1592–1594. doi:10.1002/cssc.201100259
Wagle, D. V., Adhikari, L., and Baker, G. A. (2017). Computational Perspectives on Structure, Dynamics, Gas Sorption, and Bio-Interactions in Deep Eutectic Solvents. Fluid Phase Equilibria 448, 50–58. doi:10.1016/j.fluid.2017.04.018
Wang, S., Zhao, Y., Lin, H., Chen, J., Zhu, L., and Luo, Z. (2017). Conversion of C5 Carbohydrates into Furfural Catalyzed by a Lewis Acidic Ionic Liquid in Renewable γ-valerolactone. Green Chem. 19 (16), 3869–3879. doi:10.1039/c7gc01298e
Wang, W., Ren, J., Li, H., Deng, A., and Sun, R. (2015). Direct Transformation of Xylan-type Hemicelluloses to Furfural via SnCl4 Catalysts in Aqueous and Biphasic Systems. Bioresour. Technol. 183, 188–194. doi:10.1016/j.biortech.2015.02.068
Wang, Y., Fan, C., Hu, H., Li, Y., Sun, D., Wang, Y., et al. (2016). Genetic Modification of Plant Cell Walls to Enhance Biomass Yield and Biofuel Production in Bioenergy Crops. Biotechnol. Adv. 34 (5), 997–1017. doi:10.1016/j.biotechadv.2016.06.001
Wang, Z.-K., Shen, X.-J., Chen, J.-J., Jiang, Y.-Q., Hu, Z.-Y., Wang, X., et al. (2018). Lignocellulose Fractionation into Furfural and Glucose by AlCl3-Catalyzed DES/MIBK Biphasic Pretreatment. Int. J. Biol. Macromol. 117, 721–726. doi:10.1016/j.ijbiomac.2018.05.232
Wu, C., Yuan, W., Huang, Y., Xia, Y., Yang, H., Wang, H., et al. (2017). Conversion of Xylose into Furfural Catalyzed by Bifunctional Acidic Ionic Liquid Immobilized on the Surface of Magnetic γ-Al2O3. Catal. Lett. 147 (4), 953–963. doi:10.1007/s10562-017-1982-z
Xu, G.-C., Ding, J.-C., Han, R.-Z., Dong, J.-J., and Ni, Y. (2016). Enhancing Cellulose Accessibility of Corn Stover by Deep Eutectic Solvent Pretreatment for Butanol Fermentation. Bioresour. Technol. 203, 364–369. doi:10.1016/j.biortech.2015.11.002
Yang, D., Zhang, S., Sun, X.-g., Jiang, D.-e., and Dai, S. (2019). Deep Eutectic Solvents Formed by Quaternary Ammonium Salts and Aprotic Organic Compound Succinonitrile. J. Mol. Liq. 274, 414–417. doi:10.1016/j.molliq.2018.10.150
Yang, Y., Hu, C.-W., and Abu-Omar, M. M. (2012). Synthesis of Furfural from Xylose, Xylan, and Biomass Using AlCl3⋅6 H2O in Biphasic Media via Xylose Isomerization to Xylulose. ChemSusChem 5 (2), 405–410. doi:10.1002/cssc.201100688
Yiin, C. L., Quitain, A. T., Yusup, S., Uemura, Y., Sasaki, M., and Kida, T. (2017). Choline Chloride (ChCl) and Monosodium Glutamate (MSG)-based Green Solvents from Optimized Cactus Malic Acid for Biomass Delignification. Bioresour. Technol. 244 (Pt 1), 941–948. doi:10.1016/j.biortech.2017.08.043
Yiin, C. L., Yap, K. L., Ku, A. Z. E., Chin, B. L. F., Lock, S. S. M., Cheah, K. W., et al. (2021). Recent Advances in Green Solvents for Lignocellulosic Biomass Pretreatment: Potential of Choline Chloride (ChCl) Based Solvents. Bioresour. Technol. 333, 125195. doi:10.1016/j.biortech.2021.125195
Yu, Q., Bai, R., Wang, F., Zhang, Q., Sun, Y., Zhang, Y., et al. (2019a). A Sustainable System for Maleic Acid Synthesis from Biomass‐derived Sugar. J. Chem. Technol. Biotechnol. 95 (3), 751–757. doi:10.1002/jctb.6260
Yu, Q., Song, Z., Zhuang, X., Liu, L., Qiu, W., Shi, J., et al. (2019b). Catalytic Conversion of Herbal Residue Carbohydrates to Furanic Derivatives in a Deep Eutectic Solvent Accompanied by Dissolution and Recrystallisation of Choline Chloride. Cellulose 26 (15), 8263–8277. doi:10.1007/s10570-019-02372-6
Zahn, S., Kirchner, B., and Mollenhauer, D. (2016). Charge Spreading in Deep Eutectic Solvents. Chemphyschem 17 (21), 3354–3358. doi:10.1002/cphc.201600348
Zhang, L.-X., Yu, H., Yu, H.-B., Chen, Z., and Yang, L. (2014). Conversion of Xylose and Xylan into Furfural in Biorenewable Choline Chloride-Oxalic Acid Deep Eutectic Solvent with the Addition of Metal Chloride. Chin. Chem. Lett. 25 (8), 1132–1136. doi:10.1016/j.cclet.2014.03.029
Zhang, L., and Yu, H. (2013). Conversion of Xylan and Xylose into Furfural in Biorenewable Deep Eutectic Solvent with Trivalent Metal Chloride Added. BioResources 8, 6014. doi:10.15376/biores.8.4.6014-6025
Keywords: biomass, deep eutectic solvents, furfural, catalysis, solid acid
Citation: Zhang X, Zhu P, Li Q and Xia H (2022) Recent Advances in the Catalytic Conversion of Biomass to Furfural in Deep Eutectic Solvents. Front. Chem. 10:911674. doi: 10.3389/fchem.2022.911674
Received: 03 April 2022; Accepted: 25 April 2022;
Published: 09 May 2022.
Edited by:
Subhamay Pramanik, Oak Ridge National Laboratory (DOE), United StatesReviewed by:
Ankush Gupta, DAV University, IndiaKalavathy Rajan, The University of Tennessee, Knoxville, United States
Copyright © 2022 Zhang, Zhu, Li and Xia. This is an open-access article distributed under the terms of the Creative Commons Attribution License (CC BY). The use, distribution or reproduction in other forums is permitted, provided the original author(s) and the copyright owner(s) are credited and that the original publication in this journal is cited, in accordance with accepted academic practice. No use, distribution or reproduction is permitted which does not comply with these terms.
*Correspondence: Haian Xia, aGF4aWFAZGljcC5hYy5jbg==