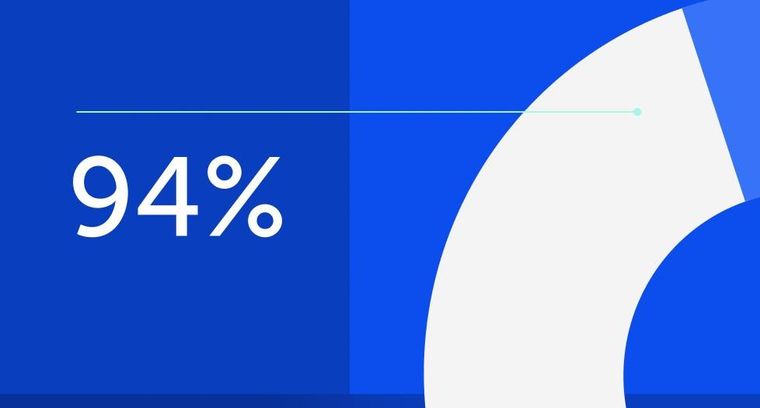
94% of researchers rate our articles as excellent or good
Learn more about the work of our research integrity team to safeguard the quality of each article we publish.
Find out more
REVIEW article
Front. Chem., 27 April 2022
Sec. Nanoscience
Volume 10 - 2022 | https://doi.org/10.3389/fchem.2022.905256
This article is part of the Research TopicBioinspired Nanomaterials: design principles for imaging and therapeuticView all 8 articles
Red Blood Cells (RBCs)-derived particles are an emerging group of novel drug delivery systems. The natural attributes of RBCs make them potential candidates for use as a drug carrier or nanoparticle camouflaging material as they are innately biocompatible. RBCs have been studied for multiple decades in drug delivery applications but their evolution in the clinical arena are considerably slower. They have been garnering attention for the unique capability of conserving their membrane proteins post fabrication that help them to stay non-immunogenic in the biological environment prolonging their circulation time and improving therapeutic efficiency. In this review, we discuss about the synthesis, significance, and various biomedical applications of the above-mentioned classes of engineered RBCs. This article is focused on the current state of clinical translation and the analysis of the hindrances associated with the transition from lab to clinic applications.
Through the past decades, the evolution of conventional drug formulations to novel drug delivery systems has brought a significant advancement in terms of patient compliance, safety, and efficacy. Researchers have extensively focused on designing optimal drug formulations that have potential to deliver cargos to the desired target sites effectively. Bio-inspired solutions have come into limelight in pursuit of improved circulation, to facilitate release of drugs at the targeted sites, and preventing clearance of drugs from the human system. Erythrocytes/red blood cell (RBC)-derived particles are novel bioinspired drug delivery platforms, in which RBCs are the vital components of the circulatory system and, in combination with different synthetic materials, exhibit the potential to impart both natural benefits and synthetic characteristics for drug delivery. Natural RBCs have a lifespan of 90–120 days in circulation that makes RBCs-derived platforms lucrative for drug delivery applications, as conventional molecular drugs are cleared from the body before exerting adequate therapeutic actions, and produce toxicity when higher dosages of drugs are administered. Apart from delivering a drug to a specific site of interest, the RBC-derived systems also have favorable interactions with the neighboring complex biological environment which stimulates the functional attributes of the drug delivery systems in turn. Owing to the intrinsic biocompatible, biodegradable, and non-immunogenic nature of RBCs, they can be utilized to engineer delivery systems that have the potential for clinical translation. Besides drug loading and physico-chemical conjugation strategies, it is also important to focus on the interactions at the biological interfaces for successful bench to bedside translation (Cai et al., 2018). RBCs are innately the most abundant circulating cells in the biological environment and have inspired the engineering of various man-made RBC-based drug delivery systems in the past several decades. In the last two decades, there is a rapid increase in the research and development of RBC-based drug delivery systems (Figure 1).
FIGURE 1. The research trend of using RBCs as delivery vehicles in the past two decades (2001–2021). The keywords used are “Engineered erythrocytes”, “Erythrocyte derived vesicles”, “Erythrocyte camouflaged” and “Cell membrane-derived vesicles”. The data were obtained from Web of Science.
In this review, we summarize the development of RBC coated/camouflaged drug delivery systems along with the popular RBC carrier systems. Various researchers have extensively delved into the exploration and advancement of RBCs as “Smart Delivery Systems” (Han et al., 2018). The last decade has witnessed a vast improvement in drug encapsulation methods for RBC carriers along with the emergence of cell membrane coating technologies for RBC camouflaged delivery systems (Hu et al., 2012; Fang et al., 2018). Engineered RBC carriers have the potential to harbor and transport a vast array of bioactive agents like drugs, imaging agents, enzymes, proteins, and other macromolecules (Hanley et al., 2021). Various strategies have been employed to load RBCs with therapeutic agents without jeopardizing the structural and functional characteristics of RBCs. Additionally, the use of RBC membranes as a camouflaging coating on synthetic carriers like polymeric nanoparticles have been extensively developed in quest for improved circulation half-life and stability. These systems provide combined benefits of a natural coating along with a synthetic core, leading to controlled drug release (Figure 2). RBC-camouflaged drug delivery systems were extensively explored in the field of nanomedicine, and are anticipated to overcome several challenges in the path to clinical translation. RBCs are innately suited for intravascular delivery. Native RBCs are comparatively larger, micron sized cellular components but exploration of their nano dimensions as RBC-based nanocarriers comes with added advantages of drug delivery vehicles. They readily protect the encapsulated cargos and lead to sustained release through their semi-permeable membranes leading to increased therapeutic efficiency. The RBC-coated nanocarriers have prolonged circulation time as compared to bare nanocarriers, combatting the issue of premature clearance in most of the nanocarriers.
This review article is focused on the current state of clinical translation of the RBC based drug delivery systems. We discuss the challenges related to the large-scale fabrication of such constructs, and also revisit the strategies that need to be undertaken to achieve RBC based drug delivery systems for successful translation.
RBCs are a-nucleated cells, rendering easiness in the method of extraction and purification (Pierigè et al., 2008). The preparation of RBC membrane-derived vesicles and RBC-camouflaged nanoparticles can be categorized into several methods.
Encapsulation of therapeutic or imaging agents inside the RBC derived vesicles are mediated through various techniques. Hypotonic treatment is the most commonly used method for synthesizing carrier RBCs without adversely affecting their biochemical integrity (Xia et al., 2019). Following the principle of osmotic lysis, the RBCs swell up and burst when placed in a hypotonic solution, accompanied by the depletion of the intracellular components through pores of sizes ranging from approximately 10–500 nm (Pierigè et al., 2008). There is an entrapment of cargos inside the RBCs by passive diffusion through these pores from the adjacent solution containing drug molecules. The pores on the RBC membrane are reversible and reseal preserving the mechanical integrity and biochemical functions of the membrane. Various other techniques following the same principle of osmotic lysis have also been developed, namely hypotonic hemolysis, hypotonic dilution, hypotonic dialysis and hypotonic pre-swelling (Hu et al., 2012). The hypotonic dilution technique has drawbacks related to low entrapment efficiency and poor circulation life of the resultant construct. To address these drawbacks, hypotonic dialysis was employed and proved to be a suitable method for sustaining physiological and biochemical functionalities of the RBCs.
RBC-camouflaged drug delivery systems are an efficient way to coalesce the mechanical and biological advantages of the natural RBCs with those of synthetic materials. The synthesis and assembly of the RBC membranes with the nanoparticles of interest is pivotal for designing RBC-camouflaged nanoparticles. Extrusion (Guo et al., 2018) and sonication are the most commonly used techniques for preparation of RBC-camouflaged nanoparticles (Figure 3) (Guido et al., 2021). However, in situ polymerisation and microfluidic electroporation are less popular, and are gradually being explored considering the difficulties in the synthesis processes, reproducibility and scale-up challenges associated with the manufacturing for clinical translation. The biological membrane extrusion method enables achieving uniformly sized particles by passage through porous membranes of required dimensions (Hu et al., 2012; Gao et al., 2013). Extrusion has been reported to be an efficient method to obtain homogenous RBC membrane coating on a poly (D, L-lactic-co-glycolic acid) (PLGA) nanoparticle (HuChe Ming et al., 2011). Other researchers have also demonstrated the utility of the extrusion method to coat RBC membranes on other particles of interest (Piao et al., 2014). However, the major disadvantage of this method is the accumulation of material on the porous membranes that leads to the loss of samples. This has caused hindrance to the applicability of the extrusion technique for large-scale fabrication.
FIGURE 3. Synthesis of nano-erythrosomes using extrusion and sonication approaches. Adapted from Guido et al. (2021). Copyright @ 2021 (MDPI).
Sonication is another commonly used method to synthesize RBC-camouflaged particles where sound energy is utilised to disperse particles in a liquid sample containing both the RBC membrane and the core particles, using a probe or a bath sonicator. RBC membrane-camouflaged cross-linked 2-hydroxyethyl acrylate (HEA) hydrogel microparticles have been reported to have been synthesised by the sonication method (Hayashi et al., 2018). Sonication is advantageous over extrusion as loss of sample can be avoided during fabrication, but unlike extrusion, sonication may lead to various sizes of the resulting RBC-coated particles. Sonication factors like time, frequency and input power should be cautiously optimised and monitored to reduce chances of protein denaturation. Also, the size and stability of the particles impacted due to sonication makes the search for better synthetic strategies more essential.
Techniques like in situ polymerisation and microfluidic electroporation have also been reported to obtain RBC-camouflaged particles. RBC membrane-coated hydrogel nanoparticles have been synthesised through in situ polymerisation (Zhang et al., 2015; Zhang et al., 2017a). The RBC membrane-derived vesicles were used as an initiator to form polymeric cores, mediated through radiation or heat. The prospects of this method need to be explored further as it might make absolute coating possible through polymerisation.
Microfluidic electroporation (Figure 4) enables the formation of pores temporarily in the RBC membrane allowing the passive ingestion of the nanoparticles into the RBC-derived vesicles when exposed to electric impulse. The RBC-camouflaged Fe3O4 nanoparticles were synthesised using this approach and showed superior colloidal stability, homogenous size distribution and enhanced magnetic resonance imaging (MRI) and photothermal therapy (PTT) in vivo as compared to those synthesized using conventional extrusion method (Rao et al., 2017). Microfluidic electroporation technique is expected to address the scale-up and storage challenges faced during industrial translation, and also possess the potential for application in personalised diagnostics and therapeutics as the RBCs can be autologously acquired.
FIGURE 4. Schematic of microfluidic electroporation-facilitated synthesis of RBC-membrane capped magnetic nanoparticles. (A) Microfluidic electroporation facilitates the synthesis of RBC-MNs. (B) Subsequently, the RBC-MNs, which are collected from the microfluidic chip, accumulate at the tumor site after the blood circulation. (C) Biomimetic RBC-MNs are further used for enhanced in vivo tumor MRI and PTT. Adapted from Rao et al. (2017). Copyright @ 2017 (American Chemical Society).
RBC-derived particles exhibit prolonged circulation time, and can readily evade the phagocytic clearance in the biological environment, due to the retention of RBC membrane proteins and their specialized chemical structure. Besides, the physical characteristics of the RBC-derived particles, the biochemical parameters are also very significant for evaluation of the particle systems. The mechanical characterization of the RBC-derived systems needs further exploration, though. The following criteria are taken into consideration for characterization:
Both quantitative and qualitative assessments are crucial for studying the structural integrity of the RBC derived particles. Various techniques have been employed to observe the surface thickness, homogenous distribution, stability, deformability, and permeability of the RBC membrane-derived particles.
Dynamic Light Scattering (DLS) is the commonly employed technique to assess the average hydrodynamic diameter, polydispersity index (PDI) and zeta potential (surface charge) of both the carrier RBCs and RBC-camouflaged particles. There is no significant change in the sizes of carrier RBCs due to cargo loading. But an increase of 10–20 nm can be usually observed in the diameter of the camouflaged nanoparticles as the lipid bilayer of RBC membranes is around 8 nm thick (Su et al., 2016; Zhou et al., 2016). The surface of RBCs is negatively charged due to the presence of ionized carboxyl groups of N-acetyl neuraminic acid (NANA), commonly known as sialic acid. It was reported that the sialic acid was persistent post hypotonic treatment and mechanical extrusion imparting negative charge to the RBC membrane. But sonication presumably results in the degradation of the sialic acid content of the RBC membrane as a decrease in zeta potential of the RBCs and the engineered RBCs was noted in a study (Tang et al., 2022). Hence, the surface charges of both the RBC carriers and RBC-camouflaged nanoparticles have been noted to be negative owing to the negative surface charge of the native RBC membrane.
The shape, morphology, and structural integrity of carrier RBC and RBC-camouflaged nanoparticles are verified by microscopic techniques. Transmission electron microscopy (TEM) and scanning electron microscopy (SEM) offer excellent visualization of the RBC-derived particle systems. The RBC-camouflaged nanoparticles can also be negatively stained with uranyl acetate and observed under TEM to verify their characteristic “core-shell” structure upon successful coating (Wang et al., 2018). Different spectroscopic methods have been employed to study the physicochemical characteristics of the RBC membrane-derived systems. Protein content of the RBC membrane can be quantitatively analyzed by mass spectroscopy technique. UV-visible absorption spectroscopy has been used to confirm successful encapsulation of RBC carrier and RBC camouflaged systems. For RBC-camouflaged systems, Fourier transform infra-red (FT-IR) spectroscopy is an useful method to confirm proper coating (Chugh et al., 2021). Fluorescence co-localization enables introduction of hydrophobic and lipophilic fluorophores into the RBC-camouflaged systems to form “dual-fluorophore labelled” systems, and verifies successful coating and the structural integrity of the “core-shell” arrangement after cellular internalization (Hu et al., 2012).
The greatest advantage of RBC-derived drug delivery systems is their ability to achieve a prolonged circulatory effect in the biological environment. Specific RBC membrane proteins present the particles as “self” to the body, thereby decreasing the host immune response and prolonging the systemic circulation. Hence, the verification of surface proteins is of utmost significance to confirm successful coating on the nanoparticles in the RBC-camouflaged systems. Sodium dodecyl sulphate-polyacrylamide gel electrophoresis (SDS-PAGE) and staining with Commassie blue enable the visualization of the proteins to confirm the presence of all proteins on the RBC and the RBC-derived particles, alike (HuChe-Ming et al., 2013). Specific proteins are confirmed with the help of Western blot analysis. CD235a, also known as glycoprotein A (Gagneux and Varki, 1999), is the main RBC sialic acid glycoprotein, and the blood group A antigen is shown to be present in RBC vesicles as well as in RBC-coated nanoparticles. Cluster of differentiation 47 (CD47) is found to be in an equal degree in RBC vesicles, RBC membrane-camouflaged nanoparticles, and RBC cells. Besides analyzing the presence of the surface proteins, their biological activities can also be ascertained through cellular studies. Researchers have demonstrated in mouse macrophage RAW264.7 cell lines that the uptake of RBC-camouflaged nanoparticles was considerably lower than the uptake of naked nanoparticles, thereby indicating the immune escape capability of RBC camouflaged systems. More studies have also been performed to establish the protein orientation and interactions.
The drug loading and drug encapsulation efficiencies are the prime factors to dictate the plethora of possible medical applications, which the RBC-based drug delivery systems can achieve. Researchers employ chromatographic techniques to quantify the drugs in the RBC carriers and in the RBC-camouflaged systems. A vast array of biological molecules comprising of nanoparticles, pharmaceutical drugs, imaging agents, and genetic materials, etc. can be encapsulated within the RBC-derived membranes. Drug loading efficiency and drug release behavior have been studied for RBC carriers and RBC-camouflaged particles. It was reported that RBC-camouflaged nanoparticles after 72 h showed only 20% drug release while PEGylated nanoparticles showed 40% drug release, proving that RBC membrane creates a barrier for external diffusion of drugs facilitating gradual and sustained release (Xia et al., 2019). Also, if RBC membrane-derived vesicles contain photosensitizers like hematoporphyrin derivatives, or if RBC-camouflaged nanoparticles contain photosensitive nanoparticles like semiconducting polymer nanoparticles (SPN) (Zheng et al., 2020), then stimulus-responsive, time-dependent drug release studies can be undertaken. Studies have also shown near infrared (NIR) light-initiated and pH-triggered drug release. Drug release studies by many researchers have established that RBC-based drug delivery systems are advantageous in terms of prolonged circulation time and sustained release (Zhang et al., 2021).
RBCs are the major components of the circulatory system. These cells are unique as compared to other body cells on account of their long circulation life, high deformability, and lack of organelles. RBCs are 7–8 µm biconcave discs with a high surface area to volume ratio. They survive in circulation for almost 120 days in humans and 50 days in mice without being cleared by macrophages. This property makes RBCs a suitable drug delivery system preventing encapsulated cargos from rapid clearance, thus achieving sustainable release (Han et al., 2018). The extended circulation half-life is a result of the self-markers present on the membrane. CD47 is a surface protein that is highly expressed on the RBC membrane. CD47 specifically binds to signal-regulatory protein alpha (SIRP α) glycoprotein to generate a “do not eat me” signal, that negatively controls the effector functions of innate immune cells including phagocytes (Chambers and Mitragotri 2007). Also, RBC surface proteins like Complement Receptor 1 (CR1) and Decay Accelerating Factor (DAF) can prevent inappropriate self-recognition by an alternate complement pathway affected by C3 Convertase (Fearon 1979). Antibody-mediated complement activation protein referred to as Membrane Attack Complex (MAC) is also inhibited by C8 binding protein (C8bp) present in the RBC membrane, thus escaping immune response. Researchers have reported that CD47 is retained on both micron-sized and nano-sized RBC membranes post mechanical extrusion. Due to the above-mentioned factors, the engineered RBCs are designated as “self” by the human body and subsequently could escape from the phagocytic clearance that any foreign substance in the body is primarily subjected to. Evasion of macrophagic uptake by the RBC carriers and RBC-camouflaged nanoparticles in turn prolongs their circulation time and hence has popularized the concept of RBC-based drug delivery systems. Also, the spleen exhibits a potent filtering mechanism to separate healthy RBCs from the physiologically senescent or pathologically altered RBCs that can be recognized when there is any alteration in size, shape, or deformability. Nano-sized RBC constructs, owing to their lesser diameter, are capable of passing through the splenic endothelial slits and re-entering the circulation.
Healthy RBCs possess appropriate mechanical attributes that differentiate them from aged or damaged RBCs with reduced deformability (Mohanty et al., 2014). Nash et al. have reported an almost 35% increase in the surface viscosity of the membrane of old RBCs in comparison to the young ones (Nash and Meiselman, 1983). Due to a decrease in the deformability, aged RBCs are unable to pass through the endothelial slits of the splenic venous sinus and will be removed from the circulation by phagocytosis in due course. In a similar manner, micron-sized RBC constructs that have been reported to have a decreased deformability remain within the cords unable to pass through the splenic slits, eventually get phagocytosed by the splenic macrophages (Vankayala et al., 2019).
Phosphatidylserine (PS), one of the major phospholipids, usually presents on the inner leaflet of the lipid bilayer of the RBC membrane. During the fabrication of RBC-based particles, there is PS flipping from the inner to the outer leaflet, signaling phagocytic clearance of the particles from the circulation due to macrophage recognition. Researchers have reported a cholesterol enrichment method for the RBC membrane that has successfully reduced PS externalization to enhance the longevity of the RBC-based micron-sized particles in circulation (Tang et al., 2022).
RBC-based drug delivery systems have come into the limelight with a focus on evading phagocytic clearance, leading to prolonged circulation time and less toxicity due to the “self” nature of RBCs in the biological environment. Researchers have studied the circulation characteristics of the RBC membrane-derived vesicles over the synthetic materials, and concluded that retention of CD47 is the major cause to prevent RES uptake and to improve the plasma circulation time (Gao et al., 2013). RBC-derived constructs have been reported to be biocompatible and potentially non-toxic in animal studies. They showed no long-term toxicity in mice where only 20% of the injected particles could be detected after 48 h in the hepatic circulation (Vankayala et al., 2019). Biotoxicity has been evaluated in vivo by examining serum biochemistry profiles and histological specimens (Rao et al., 2017). Further short-term and long-term studies are essential to establish the non-toxic behavior of these constructs. RBCs are intrinsically biocompatible, biodegradable and apparently non-immunogenic, yet further evaluation of any other type of immune toxicity, complement activation-related pseudo-allergy (CARPA) or hypersensitivity states need to be conducted. Clarity is required regarding the interaction of the RBC membrane-derived vesicles with the complement system. Researchers have recommended the evaluation of CARPA and hypersensitivity potential of RBC-based delivery systems prior human administration (Malhotra et al., 2022). Utilization of clinically approved components in the RBC-based delivery systems might be advantageous in terms of validating therapeutic potential and immunogenicity. Selection of proper in vivo animal models is also crucial for assessment of immune compatibility and systemic toxicity.
There has been an expedited development of RBC-based drug delivery systems with the advent of various cargo loading methods and membrane coating techniques. RBC-derived vesicles acting as carrier for drugs and other active agents provide a wide range of opportunities in transporting genes, nanoparticles, drugs, contrast agents that are poorly soluble in water, have low bioavailability and are in need of prolonged circulation period by trapping them which make them more stable and inherently biocompatible. On the other hand, they can also act as camouflaging mantle overlaying the nanoparticles. Some nanomaterials, despite excellent intrinsic properties, cannot be administered in their bare forms because of their immunogenic effects, rapid clearance and toxic bioaccumulation. For example, a very promising photosensitizer, copper sulfide (CuS) nanoplates showed accumulation in spleen, liver, and lungs of mice (Feng et al., 2015). Heavy metal quantum dots like zinc sulfide (ZnS) and cadmium sulfide (CdS) have been shown to Feng et al., 2015 accumulate in zebrafish model (Matos et al., 2020). In order to be used extensively for its outstanding properties, RBC membrane is used as a camouflage to hide the native characteristics of nanoparticles and to endow them with prolonged circulation with minimal or no immune effects and accumulation. Figure 5 shows the applications of the RBC-based drug delivery systems. Table 1 enumerates the various biomedical applications for which RBC-based delivery systems have been explored.
FIGURE 5. Applications of RBC carriers and RBC-camouflaged systems as carriers for diagnostics, genetic materials, therapeutics, etc.
The major disadvantage of conventional chemotherapeutic drugs is the inability to escape from clearance during blood circulation before exerting their therapeutic efficacies. In order to achieve optimal effect, a large dose of drug needs to be administered so that the required amount of drug is able to reach the target site and act effectively. To achieve enhanced bioavailability of drugs in the tumor region even with low concentrations, tumor targeting modifications are required especially for deep-seated tumors. Also, for the diagnostics of deep-seated tumors and circulating/metastatic tumors, conventional imaging system is unable to give properly defined tumor outlines that can be used as reference in surgery. RBC cloaking provide the properties of RBC that makes the diagnostic agent or drug reach any part which makes the drug delivery and diagnostics facile and feasible.
Camptothecin, a lipophilic drug/dye along with CM-DiI, was co-loaded in RBC-derived nanovesicles for theranostic applications (Figure 6). The nanoparticle was non-phagocytic, and showed strong retention with slow release and superior stealth because of the naturally derived carrier (Malhotra et al., 2019).
FIGURE 6. Schematic representation showing usages of RBC membrane for loading a lipophilic drug, camptothecin, for theranostic application. Adapted from Malhotra et al. (2019). Copyright @ 2019 (American Chemical Society).
To overcome the problems of rapid phagocytosis and short intravascular half-life, contrast agents were cloaked in RBC membranes, thereby circumventing the macrophage system and elimination from the body. Superparamagnetic iron oxide nanoparticles (SPIONs) (Brähler et al., 2006) (Figure 7) have been camouflaged by RBC membrane and reported to show enhanced contrast in MRI for diagnostic applications. Researchers have portrayed several ways of functionalizing contrast agents with RBC membrane for optical imaging and diagnostics applications using nano-erythrosomes (Fornasier et al., 2021).
FIGURE 7. Using RBC membrane as a camouflage for diagnostics. (A) Magnetite-loaded RBCs respond to an attached external permanent magnet. (B) TEM pictures of (a) non-loaded control RBCs and (b) SPION-loaded RBCs. Adapted from Brähler et al. (2006). Copyright @ 2006 (American Chemical Society).
Combinatorial therapy involving two therapies at the same time is been carried out to achieve better efficacies. Doxorubicin (DOX)-loaded into hollow prussian blue nanoparticle (Chen et al., 2017), paclitaxel and IR-780 co-loaded in lipid multi-chambered nanoparticles (Zhang et al., 2021) and DOX-loaded hollow copper sulfide (CuS) nanoparticles (Wang et al., 2018) were administered for light activated combinatorial therapies of cancer. All these nanoparticles were RBC-cloaked helping them to escape from the reticuloendothelial system (RES) and immune evasion, achieving longer circulation. The drugs or diagnostic agents can also be directly loaded into RBC membranes. A complex of bovine serum albumin (BSA), indocyanine green (ICG) and doxorubicin (DOX) which can be light-activated were encapsulated directly in the RBC vesicles of which the surface was modified with RGD peptide for targeting at the αvβ3-intergrins on tumor membrane accomplishing combinatorial chemotherapy and photothermal therapy. There is also a theranostic nano-riceball with a core made of dextran loaded with DOX and purpurin-18 buried in RBC membrane. The RBC membrane was surface-modified with NGR peptide from CRISPR engineered mice. It is a synergistic nanoparticle capable of responsiveness to endogenous H2O2 and ultrasound performing sonodynamic therapy (SDT) and chemotherapy under photoacoustic or fluorescence image guidance. This nanoparticle exhibited dramatic inhibition of tumor growths with negligible side effects (Shi et al., 2021).
For cardiovascular drugs to be impactful, it is demanded to have enhanced stay in the circulation and targeting which could be achieved using nanoparticles (NPs)-based nanocarriers. Though nanoparticles could save the drugs from rapid clearance, it remains a challenge for the nanoparticles to escape from clearance by the reticuloendothelial system (RES) and mononuclear phagocyte system (MPS). The use of RBC-derived membrane to cloak the NPs or RBC-derived vesicles to directly load the drugs makes it a win-win situation to administer any drug on its own and through nanoparticle for the treatment of Cardiovascular Diseases (CVDs). To increase the circulation half-life and to achieve targeting in arthrosclerosis, PLGA nanoparticle loaded with rapamycin was cloaked in RBC derived membrane. This nanocomplex showed superior bio-interface without significant side effects even after long term administration while nanoparticles with polyethylene glycol (PEG) elicited the host immune response after multiple administrations (Zinger et al., 2021).
Comparatively, some researchers reported a delayed progression of arthrosclerosis when the nanoparticle is cloaked with RBC (Figure 8) (Wang P. et al., 2019). For treatment of thrombus, janus nanomotors made of the polymer’s heparin and chitosan, sputter coated with gold, were cloaked with RBC membrane. It was shown that the movement of the motors were controlled by near infrared (NIR) light. Since they were cloaked, they possessed the RBC properties which made their movement more efficient in the relevant biological environments (Shao et al., 2018).
FIGURE 8. The ORO-stained images of aortas from each group (A) control; (B) free drug; (C) RAP@PLGA; and (D) RBC/RAP@PLGA. Adapted from Wang et al. (2019b). Copyright @ 2019 (Wiley).
Comprehending the inefficacy of fullerenol in thrombolysis in vivo as compared to its in vitro efficacy, Chen et al. cloaked mesoporous silica nanoparticles bearing the fullerenols with RBC membrane. They claimed that the reduced inefficacy was due to low concentrations of fullerenols at the thrombus site. This intrinsic biotaxi based on cloaking with RBC exhibits an improved the circulation time by 3.1 times, reduced the phagocytosis by 69% and reduced bleeding by 35.3 times (Chen et al., 2020). Considering the thrombosis caused by artificial heart valve, RBC membrane loaded with rapamycin and atorvastatin calcium were cross-linked onto the artificial heart valve. Such a modification improves the biocompatibility with anti-coagulation, anti-inflammation, anti-endothelialization and anti-calcification with the combination of RBC membrane and the drugs it harbors (Figure 9) (Hu et al., 2020).
FIGURE 9. Schematic of erythrocyte membrane biomimetic drug-loaded nanoparticles and mechanism of cross-linking with heart valves for anti-coagulation/accelerating endothelialization process, anti-inflammation and anti-calcification. Adapted from C. Hu et al. (2020). Copyright @ 2020 (American Chemical Society).
The shield of the blood brain barrier (BBB) to protect the brain makes it arduous to deliver drugs to the brain. It has been difficult for drug delivery systems to cross the BBB and ingress into the neural region despite considerable advancement in the field. This makes the treatment of brain diseases very challenging and the success rate with regards to encephalopathy stands very low (Li et al., 2021). RBC membrane cloaking will make it trouble-free for any molecule or nanoparticle to have their way across the BBB as their membrane properties possess the privilege of passing through all the systems of the body.
Curcumin, a multitarget drug, wielded its neuroprotective effects by activating several neurotrophic factors in the brain through various mechanisms. But due to its low water solubility, it has poor bioavailability. The use of curcumin in Alzheimer’s disease to make use of its protective effects was achieved by RBC-cloaked nanoparticles. PLGA nanoparticles loaded with curcumin was cloaked with RBC membrane and functionalized with DSPE-PEG–T807 with a size of 170 nm which could target at neuronal tau. The RBC protects curcumin by increasing its bioavailability while the T807 helps crossing the BBB with good biocompatibility, and sustained curcumin release (Figure 8B) and prolonged circulation (Figure 10) (Gao et al., 2020a). Another study has been designed with the same nanoparticle for Alzheimer’s disease except that human serum albumin was used as a carrier in place of PLGA with a particle size less than 120 nm (Gao et al., 2020b). Both studies have shown good uptakes of the nanoparticles with desired targeting for the treatment of Alzheimer’s. Immunotherapeutic effect in glioblastoma therapy was also achieved by using RBC-cloaked nanogels with a size about 131 nm carrying miRNAs. Reinforcement of microglia and macrophages by miRNAs reprograms the immunotherapeutic impact. The delivery of miRNAs was aided by nanogel cloaked with RBC membrane which contains functional peptides for targeting microglia and macrophage, and also enhanced the fusion with endosomal membrane for increased miRNA release. On a whole, the particle mimicked a virus, delivered and protected miRNAs from degradation with easy travel across the BBB due to the presence of RBC camouflaging (Gao et al., 2021).
FIGURE 10. Using RBC membrane as a camouflage for therapeutics. (A) Preparation and characterization of nanoparticles. (B) The stealth properties of RBC membrane-coated NPs in vitro and in vivo. Adapted from Gao et al. (2020a).
Most of the drugs, chemicals and molecules used for treatment of multifarious diseases and conditions experience tremendous side-effects. This is because of their poor bioavailability and rapid clearance. RBC cloaking provides refuge by protecting the payloads from endogenous factors, ensuring decrease in concentration fluctuations, longer retention time and reduced dosage (Hamidi et al., 2007).
In a study, primaquine phosphate was used for malaria, but upon broken down metabolites are toxic to blood cells. Delivery of primaquine was stabilized by loading them in RBC membranes which were treated with glutaraldehyde for liver targeting. The drug-loaded erythrocytes have a size of 5.55 µm which were smaller in size than normal RBCs (6.35 µm) (Talwar and Jain 1992). To protect macrophage in murine AIDs, nucleoside analogues were loaded in glutathione-loaded erythrocytes which could deliver the payload drugs selectively to macrophages, thereby reducing the viral load (Fraternale et al., 2002). The same group designed a modified RBC vesicle loaded with glutathione which could be selectively phagocytized by macrophages to exert antiretroviral therapy. RBC as a drug carrier protected the glutathione from being oxidized, and delivered them only in macrophages (Fraternale et al., 2003). Isoniazid, a tuberculosis drug with a rapid clearance rate of 1–1.5 h, was encapsulated in RBC along with magnetite for targeted release under an external magnetic field. Such a controlled release highly reduces the hepatotoxicity and neurotoxicity caused by the drug upon repeated administration (Jain et al., 1997). Fludarabine-loaded RBCs were administered to kill the persistent chronically HIV-1 infected macrophages in circulation, that selectively killed infected macrophages and showed significant efficacy (>98%) in single exposure which lasted for 4 weeks (Magnani et al., 2003). Researchers have also reported RBC coated nano sponges that have shown detoxifying effects in organophosphate poisoning (Chen et al., 2019). The same group has also reported RBC coated nanogels and RBC coated sponges for antibiotic delivery in methicillin resistant Staphylococcus aureus (MRSA) infections (Chen et al., 2019a) (Zhang Z. et al., 2017).
RBCs labelled with radiotracers like Tc99m have been successfully used in the clinics as imaging probes for diagnosis of vascular abnormalities. The growing use of radiolabelled RBCs for diagnostics has kindled curiosity and motivation among researchers to explore RBC as a therapeutic agent as well. In pursuit of discovering other avenues for diagnostics and therapeutics, RBC membrane derived vesicles have attracted much interest. Engineered RBCs exhibit great potential to be utilized for various diagnostic and therapeutic purposes, and have slowly but sturdily made it to the clinical development stage from the primary research arena. Despite challenges, varied industrial assistance and government initiatives have enabled the technology to be translated for further clinical and commercial approval (Glassman et al., 2020). Several RBC carrier systems have reached the preclinical or clinical stages but RBC camouflaged systems are yet to overcome the barriers. EryDex, a sustained dexamethasone delivery system, has been developed by an Italian company, EryDel, which showed satisfactory therapeutic efficacy against ataxia-telangiectasia, a rare genetic disease, during its pilot Phase II trial (NCT01255358) (Chessa et al., 2014). The product is under pharmacokinetic investigations in the U.S. and awaiting a Phase III trial at this moment. French pharmaceutical company ERYTECH has expanded the technology by developing a novel solution (GR-ASPA) for treating acute lymphoblastic leukemia in pediatric and adult patients (NCT01518517) (Thomas and le Jeune, 2016). The therapeutic applications of homologous erythrocytes loaded with L-asparaginase (GR-ASPA) have been further supported by a Phase I clinical study in the U.S and other clinical trials in Europe. A trial IIb for investigating the effect of GR-ASPA in acute myeloid leukaemia is in process (NCT01810705) (Agrawal et al., 2013). Therapeutic effects of L-asparaginase encapsulated in RBC combined with Gemcitabine or FOLFOX have also been validated in Phase II trial for progressive metastatic pancreatic carcinoma (NCT02195180) (Hammel et al., 2020). Table 2 lists the details of various RBC-based formulations that have reached different stages of clinical trials.
TABLE 2. Overview of clinical trials of various RBC-based formulations (Source: ClinicalTrials.gov).
Though the RBC-camouflaged systems are unique and superior structurally and functionally than those without RBC-coating layers, there are roadblocks that need to be overcome for a smooth transition from academic settings to clinics. Primarily, there is lack of precise guidelines regarding characterization of these constructs that limit their reproducibility at an industrial scale. Additionally, therapeutic considerations in an actual clinical setting are vital as the in vitro and in vivo validation studies are restricted to labs and will require stringent modifications. Several factors that are likely to pose as hindrances in the path of clinically translating and commercializing the formulations, need to be catered.
RBC-based drug delivery systems mimic the natural environment and display robustness, biocompatibility, and adaptability typical of biological systems. As a result, the research community has been exploring RBC-based systems extensively. The arena is still open for various investigations in the field of RBC membrane-derived carriers and RBC-camouflaged nanoparticle systems. Several challenges exist that need to be combatted for smooth transitioning of the RBC-based drug delivery systems from basic research to the clinics that may be enumerated as the following:
• Source of RBC: Laboratory research till date has utilized whole mice or bovine blood or blood from human subjects to fabricate the RBC based constructs. Implementation in the clinics will require blood from individual patients as the technology holds great potential for personalized therapy. Autologous source of blood is the most preferred to validate maximal functionality. These constructs can also be developed using stored blood from the blood bank but possible mechanical alterations and the biochemical fluctuations need to be cautiously explored. RBCs obtained from an erythroid culture system may serve as a source but the process is cumbersome and may not be practically feasible.
• Challenges related to scale-up and reproducibility in batch-to-batch variations: Minuscule differences in the physicochemical characteristics can also cause major pharmacological issues affecting the overall precision of the system. Besides, explicit monitoring of the manufacturing process, standardized protocols for isolation of RBC membranes on a large scale is crucial.
• Post fabrication storage and stability: Use of cryoprotectants is vital for long term storage of the RBC membrane-derived systems. More evidences are essential for selection of correct cryoprotectants and verification of physical and optical stabilities of the constructs in response to the freeze-pump-thaw cycles.
• Patient specific mass manufacturing: The constructs are expected to be sensitive to different blood groups. Donor screening will be indispensable to rule out hemocompatibility issues. Researchers have used freshly obtained blood from human sources or mice blood to fabricate these constructs in the lab but replicating the same in an industrial setting is cumbersome. Utilization of stored blood in this regard can prove advantageous, but studies have reported reduction in membrane protein content, especially a decreased CD47 in stored blood (Kriebardis et al., 2008). CD47 plays a fundamental role in evasion of phagocytic clearance of the RBC-camouflaged nano constructs, hence precise studies need to be conducted to establish the therapeutic potential of the constructs sourced from stored blood.
• Sterilization of RBC-camouflaged delivery systems: Optimal sterilization techniques need to be employed to ensure de-contamination and removal of denatured membrane proteins without compromising the safety and efficacy of the constructs.
• Regulatory approval: Achieving regulatory approvals for nanomedicines has been a tough challenge despite them showing great promises. With extensive ongoing researches globally, these difficulties can be anticipated to be combatted very soon.
• Production economics: Drug development economics is a significant issue that needs to be taken care of meticulously.
The RBC based drug delivery systems have been found to be beneficial in terms of bioavailability, drug loading and controlled drug release. Besides engineering of the delivery systems, major focus should be put on conducting studies, and considering the clinical perspective. Immuno-compatibility of these constructs needs to be strongly established prior to clinical use to minimize cross reactivity. The biodistribution profiles and clearance of these constructs should also be evaluated in larger animal models besides mice. Xenografts from patients can be inspected instead of cell line derived pathologies in mice to achieve analogous results that might serve beneficial for clinical translation. We have also discussed the difficulties in large-scale manufacturing related to commercialization of the RBC delivery systems. The RBC-derived drug delivery systems have great potential to contribute to personalized therapy. Addressing the above-mentioned challenges will bring about a paradigm shift in the field of novel drug delivery systems.
RV designed and conceptualized the review. AV, SM, and Amrita drafted the manuscript. KCH and IB have reviewed and provided significant inputs. RV critically reviewed and prepared the final version of the manuscript. All authors have read and agreed to the published version of the manuscript.
The authors declare that the research was conducted in the absence of any commercial or financial relationships that could be construed as a potential conflict of interest.
All claims expressed in this article are solely those of the authors and do not necessarily represent those of their affiliated organizations, or those of the publisher, the editors and the reviewers. Any product that may be evaluated in this article, or claim that may be made by its manufacturer, is not guaranteed or endorsed by the publisher.
RV acknowledges the funding support provided by the Department of Biotechnology (DBT), Government of India for the Ramalingaswami Biomedical Fellowship (BT/RLF/Re-entry/17/2018) and also the seed grant (I/SEED/RRV/20200076) funded by Indian Institute of Technology Jodhpur.
Agrawal, V., Hee WooWoo, J., Borthakur, G., Kantarjian, H., and E. Frankel, A. (2013). Red Blood Cell-Encapsulated L-Asparaginase: Potential Therapy of Patients with Asparagine Synthetase Deficient Acute Myeloid Leukemia. Ppl 20 (4), 392–402. doi:10.2174/0929866511320040003
Antonelli, A., Sfara, C., Weber, O., Pison, U., Manuali, E., Salamida, S., et al. (2016). Characterization of Ferucarbotran-Loaded RBCs as Long Circulating Magnetic Contrast Agents. Nanomedicine 11 (21), 2781–2795. doi:10.2217/nnm-2016-0216
Bahmani, B., Bacon, D., and Anvari, B. (2013). Erythrocyte-Derived Photo-Theranostic Agents: Hybrid Nano-Vesicles Containing Indocyanine Green for Near Infrared Imaging and Therapeutic Applications. Sci. Rep. 3 (1), 2180. doi:10.1038/srep02180
Biagiotti, S., Rossi, L., Bianchi, M., Giacomini, E., Pierigè, F., Serafini, G., et al. (2011). Immunophilin-Loaded Erythrocytes as a New Delivery Strategy for Immunosuppressive Drugs. J. Controlled Release 154 (3), 306–313. doi:10.1016/j.jconrel.2011.05.024
Brähler, M., Georgieva, R. S. M., Buske, N., Müller, A., Müller, S., Pinkernelle, J., et al. (2006). Magnetite-Loaded Carrier Erythrocytes as Contrast Agents for Magnetic Resonance Imaging. Nano Lett. 6 (11), 2505–2509. doi:10.1021/nl0618501
Cai, P., Zhang, X., Wang, M., Wu, Y.-L., and Chen, X. (2018). Combinatorial Nano-Bio Interfaces. ACS Nano 12, 5078–5084. doi:10.1021/acsnano.8b03285
Chambers, E., and Mitragotri, S. (2007). Long Circulating Nanoparticles via Adhesion on Red Blood Cells: Mechanism and Extended Circulation. Exp. Biol. Med. (Maywood) 232 (7), 958–966. doi:10.3181/00379727-232-2320958
Chen, K., Wang, Y., Liang, H., Xia, S., Liang, W., Kong, J., et al. (2020). Intrinsic Biotaxi Solution Based on Blood Cell Membrane Cloaking Enables Fullerenol Thrombolysis In Vivo. ACS Appl. Mater. Inter. 12 (13), 14958–14970. doi:10.1021/acsami.0c01768
Chen, M., Liu, A., Chen, B., Zhu, D.-M., Xie, W., Deng, F.-F., et al. (2019). Erythrocyte-Derived Vesicles for Circulating Tumor Cell Capture and Specific Tumor Imaging. Nanoscale 11 (25), 12388–12396. doi:10.1039/c9nr01805k
Chen, W., Zeng, K., Liu, H., Ouyang, J., Wang, L., Liu, Y., et al. (2017). Cell Membrane Camouflaged Hollow Prussian Blue Nanoparticles for Synergistic Photothermal-/Chemotherapy of Cancer. Adv. Funct. Mater. 27 (11), 1605795. doi:10.1002/adfm.201605795
Chen, Y., Zhang, Y., Chen, M., Zhuang, J., Fang, R. H., Gao, W., et al. (2019b). Biomimetic Nanosponges Suppress In Vivo Lethality Induced by the Whole Secreted Proteins of Pathogenic Bacteria. Small 15 (6), 1804994. doi:10.1002/smll.201804994
Chen, Y., Zhang, Y., Zhuang, J., LeeLee, J. H., Wang, L., Fang, R. H., et al. (2019a). Cell-Membrane-Cloaked Oil Nanosponges Enable Dual-Modal Detoxification. ACS Nano 13 (6), 7209–7215. doi:10.1021/acsnano.9b02773
Chessa, L., Leuzzi, V., Plebani, A., Soresina, A., Micheli, R., D’Agnano, D., et al. (2014). Intra-Erythrocyte Infusion of Dexamethasone Reduces Neurological Symptoms in Ataxia Teleangiectasia Patients: Results of a Phase 2 Trial. Orphanet J. Rare Dis. 9, 5. doi:10.1186/1750-1172-9-5
Chugh, V., Krishna, K. V., and Pandit, A. (2021). Cell Membrane-Coated Mimics: A Methodological Approach for Fabrication, Characterization for Therapeutic Applications, and Challenges for Clinical Translation. ACS Nano. 15 (11), 17080–17123. doi:10.1021/acsnano.1c03800
Cui, Y., Sun, J., Hao, W., Chen, M., Wang, Y., Xu, F., et al. (2020). Dual-Target Peptide-Modified Erythrocyte Membrane-Enveloped PLGA Nanoparticles for the Treatment of Glioma. Front. Oncol. 10, 563938. doi:10.3389/fonc.2020.563938
Fang, R. H., Kroll, A. V., Gao, W., and Zhang, L. (2018). Cell Membrane Coating Nanotechnology. Adv. Mater. 30 (23), 1706759. doi:10.1002/adma.201706759
Fearon, D. T. (1979). Regulation of the Amplification C3 Convertase of Human Complement by an Inhibitory Protein Isolated from Human Erythrocyte Membrane. Proc. Natl. Acad. Sci. U.S.A. 76 (11), 5867–5871. doi:10.1073/pnas.76.11.5867
Feng, W., Nie, W., Cheng, Y., Zhou, X., Chen, L., Qiu, K., et al. (2015). In Vitro and In Vivo Toxicity Studies of Copper Sulfide Nanoplates for Potential Photothermal Applications. Nanomedicine: Nanotechnology, Biol. Med. 11 (4), 901–912. doi:10.1016/j.nano.2014.12.015
Fornasier, M., Porcheddu, A., Casu, A., Raghavan, S. R., Jönsson, P., Schillén, K., et al. (2021). Surface-Modified Nanoerythrosomes for Potential Optical Imaging Diagnostics. J. Colloid Interf. Sci. 582, 246–253. doi:10.1016/j.jcis.2020.08.032
Fraternale, A., Casabianca, A., Orlandi, C., Cerasi, A., Chiarantini, L., Brandi, G., et al. (2002). Macrophage Protection by Addition of Glutathione (GSH)-Loaded Erythrocytes to AZT and DDI in a Murine AIDS Model. Antiviral Res. 56 (3), 263–272. doi:10.1016/s0166-3542(02)00128-6
Fraternale, A., Casabianca, A., Rossi, L., Chiarantini, L., Schiavano, G. F., Palamara, A. T., et al. (2003). Erythrocytes as Carriers of Reduced Glutathione (GSH) in the Treatment of Retroviral Infections. J. Antimicrob. Chemother. 52 (4), 551–554. doi:10.1093/jac/dkg428
Gagneux, P., and Varki, A. (1999). Evolutionary Considerations in Relating Oligosaccharide Diversity to Biological Function. Glycobiology 9 (8), 747–755. doi:10.1093/GLYCOB/9.8.747
Gao, C., Chu, X., Gong, W., Zheng, J., Xie, X., Wang, Y., et al. (2020a). Neuron Tau-Targeting Biomimetic Nanoparticles for Curcumin Delivery to Delay Progression of Alzheimer's Disease. J. Nanobiotechnol 18 (1), 71. doi:10.1186/s12951-020-00626-1
Gao, C., Wang, Y., Sun, J., Han, Y., Gong, W., Li, Y., et al. (2020b). Neuronal Mitochondria-Targeted Delivery of Curcumin by Biomimetic Engineered Nanosystems in Alzheimer's Disease Mice. Acta Biomater. 108, 285–299. doi:10.1016/j.actbio.2020.03.029
Gao, M., Hu, A., Sun, X., Wang, C., Dong, Z., Feng, L., et al. (2017). Photosensitizer Decorated Red Blood Cells as an Ultrasensitive Light-Responsive Drug Delivery System. ACS Appl. Mater. Inter. 9 (7), 5855–5863. doi:10.1021/acsami.6b15444
Gao, W., Hu, C.-M. J., Fang, R. H., Luk, B. T., Su, J., and Zhang, L. (2013). Surface Functionalization of Gold Nanoparticles with Red Blood Cell Membranes. Adv. Mater. 25 (26), 3549–3553. doi:10.1002/ADMA.201300638
Gao, X., Li, S., Ding, F., Liu, X., Wu, Y., Li, J., et al. (2021). A Virus‐Mimicking Nucleic Acid Nanogel Reprograms Microglia and Macrophages for Glioblastoma Therapy. Adv. Mater. 33 (9), 2006116. doi:10.1002/adma.202006116
Glassman, P. M., Villa, C. H., Ukidve, A., Zhao, Z., Smith, P., Mitragotri, S., et al. (2020). Vascular Drug Delivery Using Carrier Red Blood Cells: Focus on RBC Surface Loading and Pharmacokinetics. Pharmaceutics 12 (5), 440–521. doi:10.3390/pharmaceutics12050440
Guido, C., Maiorano, G., Gutiérrez-Millán, C., Cortese, B., Trapani, A., D’Amone, S., et al. (2021). Erythrocytes and Nanoparticles: New Therapeutic Systems. Appl. Sci. 11 (5), 2173. doi:10.3390/app11052173
Guo, P., Huang, J., Zhao, Y., Martin, C. R., Zare, R. N., and Moses, M. A. (2018). Nanomaterial Preparation by Extrusion through Nanoporous Membranes. Small 14 (18), 1703493. doi:10.1002/SMLL.201703493
Hamidi, M., Zarrin, A., Foroozesh, M., and Mohammadi-Samani, S. (2007). Applications of Carrier Erythrocytes in Delivery of Biopharmaceuticals. J. Controlled Release 118 (2), 145–160. doi:10.1016/j.jconrel.2006.06.032
Hammel, P., Fabienne, P., Mineur, L., Metges, J.-P., Andre, T., de La Fouchardiere, C., et al. (2020). Erythrocyte-Encapsulated Asparaginase (Eryaspase) Combined with Chemotherapy in Second-Line Treatment of Advanced Pancreatic Cancer: An Open-Label, Randomized Phase IIb Trial. Eur. J. Cancer 124, 91–101. doi:10.1016/j.ejca.2019.10.020
Han, X., Wang, C., and Liu, Z. (2018). Red Blood Cells as Smart Delivery Systems. Bioconjug. Chem. 29 (4), 852–860. doi:10.1021/acs.bioconjchem.7b00758
Hanley, T., Vankayala, R., Lee, C.-H., Tang, J. C., Burns, J. M., and Anvari, B. (2021). Phototheranostics Using Erythrocyte-Based Particles. Biomolecules 11 (5), 729. doi:10.3390/biom11050729
Hayashi, K., Yamada, S., Sakamoto, W., Usugi, E., Watanabe, M., and Yogo, T. (2018). Red Blood Cell-Shaped Microparticles with a Red Blood Cell Membrane Demonstrate Prolonged Circulation Time in Blood. ACS Biomater. Sci. Eng. 4 (8), 2729–2732. doi:10.1021/acsbiomaterials.8B00197
Hu, C.-M. J., Fang, R. H., and Zhang., L. (2012). Erythrocyte-Inspired Delivery Systems. Adv. Healthc. Mater. 1 (5), 537–547. doi:10.1002/adhm.201200138
Hu, C., Luo, R., and Wang, Y. (2020). Heart Valves Cross-Linked with Erythrocyte Membrane Drug-Loaded Nanoparticles as a Biomimetic Strategy for Anti-coagulation, Anti-inflammation, Anti-calcification, and Endothelialization. ACS Appl. Mater. Inter. 12 (37), 41113–41126. doi:10.1021/acsami.0c12688
HuChe Ming, C.-M. J., Zhang, L., Aryal, S., Cheung, C., FangFang, R. H., and Zhang, L. (2011). Erythrocyte Membrane-Camouflaged Polymeric Nanoparticles as a Biomimetic Delivery Platform. Proc. Natl. Acad. Sci. U.S.A. 108 (27), 10980–10985. doi:10.1073/pnas.1106634108
HuChe-Ming, C.-M. J., FangFang, R. H., LukLuk, B. T., Chen, K. N. H., Carpenter, C., Gao, W., et al. (2013). 'Marker-of-self' Functionalization of Nanoscale Particles through a Top-Down Cellular Membrane Coating Approach. Nanoscale 5, 2664. doi:10.1039/c3nr00015j
Jain, S., Jain, S. K., and Dixit, V. K. (1997). Magnetically Guided Rat Erythrocytes Bearing Isoniazid: Preparation, Characterization, and Evaluation. Drug Dev. Ind. Pharm. 23, 999–1006. doi:10.3109/03639049709149153
Jiang, A., Song, B., Ji, X., Peng, F., Wang, H., Su, Y., et al. (2018). Doxorubicin-Loaded Silicon Nanoparticles Impregnated into Red Blood Cells Featuring Bright Fluorescence, Strong Photostability, and Lengthened Blood Residency. Nano Res. 11 (4), 2285–2294. doi:10.1007/s12274-017-1850-6
Kriebardis, A. G., Antonelou, M. H., Stamoulis, K. E., Economou-Petersen, E., Margaritis, L. H., and Papassideri, I. S. (2008). RBC-derived Vesicles during Storage: Ultrastructure, Protein Composition, Oxidation, and Signaling Components. Transfusion 48 (9), 1943–1953. doi:10.1111/j.1537-2995.2008.01794.x
Li, C., Yang, X.-Q., An, J., Cheng, K., Hou, X.-L., Zhang, X.-S., et al. (2020a). Red Blood Cell Membrane-Enveloped O2 Self-Supplementing Biomimetic Nanoparticles for Tumor Imaging-Guided Enhanced Sonodynamic Therapy. Theranostics 10 (2), 867–879. doi:10.7150/thno.37930
Li, H., Peng, Q., Yang, L., Lin, Y., Chen, S., Qin, Y., et al. (2020b). High-Performance Dual Combination Therapy for Cancer Treatment with Hybrid Membrane-Camouflaged Mesoporous Silica Gold Nanorods. ACS Appl. Mater. Inter. 12 (52), 57732–57745. doi:10.1021/acsami.0c18287
Li, Y.-J., Wu, J.-Y., Liu, J., Qiu, X., Xu, W., Tang, T., et al. (2021). From Blood to Brain: Blood Cell-Based Biomimetic Drug Delivery Systems. Drug Deliv. 28 (1), 1214–1225. doi:10.1080/10717544.2021.1937384
Liu, T., Shi, C., Duan, L., Zhang, Z., Luo, L., Goel, S., et al. (2018). A Highly Hemocompatible Erythrocyte Membrane-Coated Ultrasmall Selenium Nanosystem for Simultaneous Cancer Radiosensitization and Precise Antiangiogenesis. J. Mater. Chem. B 6 (29), 4756–4764. doi:10.1039/c8tb01398e
Magnani, M., Balestra, E., Fraternale, A., Aquaro, S., Paiardini, M., Cervasi, B., et al. (2003). Drug-Loaded Red Blood Cell-Mediated Clearance of HIV-1 Macrophage Reservoir by Selective Inhibition of STAT1 Expression. J. Leukoc. Biol. 74 (5), 764–771. doi:10.1189/jlb.0403156
Malhotra, S., Dumoga, S., and Singh, N. (2022). Red Blood Cells Membrane‐derived Nanoparticles: Applications and Key Challenges in Their Clinical Translation. WIREs Nanomed Nanobiotechnol. doi:10.1002/wnan.1776
Malhotra, S., Dumoga, S., Sirohi, P., and Singh, N. (2019). Red Blood Cells-Derived Vesicles for Delivery of Lipophilic Drug Camptothecin. ACS Appl. Mater. Inter. 11 (25), 22141–22151. doi:10.1021/acsami.9B04827
Matos, B., Martins, M., Samamed, A. C., Sousa, D., FerreiraDiniz, I., and Diniz, M. S. (2020). Toxicity Evaluation of Quantum Dots (ZnS and CdS) Singly and Combined in Zebrafish (Danio Rerio). Ijerph 17 (1), 232. doi:10.3390/ijerph17010232
Mohanty, J. G., Nagababu, E., and Rifkind, J. M. (2014). Red Blood Cell Oxidative Stress Impairs Oxygen Delivery and Induces Red Blood Cell Aging. Front. Physiol. 5, 84. doi:10.3389/fphys.2014.00084
Nash, G. B., and Meiselman, H. J. (1983). Red Cell and Ghost Viscoelasticity. Effects of Hemoglobin Concentration and In Vivo Aging. Biophysical J. 43 (1), 63–73. doi:10.1016/S0006-3495(83)84324-0
Piao, J.-G., Wang, L., Gao, F., You, Y.-Z., Xiong, Y., and Yang, L. (2014). Erythrocyte Membrane Is an Alternative Coating to Polyethylene Glycol for Prolonging the Circulation Lifetime of Gold Nanocages for Photothermal Therapy. ACS Nano 8 (10), 10414–10425. doi:10.1021/nn503779d
Pierigè, F., Serafini, S., Rossi, L., and Magnani, M. (2008). Cell-Based Drug Delivery. Adv. Drug Deliv. Rev. 60 (2), 286–295. doi:10.1016/J.ADDR.2007.08.029
Rao, L., Cai, B., Bu, L.-L., Liao, Q.-Q., Guo, S.-S., ZhaoZhao, X.-Z., et al. (2017). Microfluidic Electroporation-Facilitated Synthesis of Erythrocyte Membrane-Coated Magnetic Nanoparticles for Enhanced Imaging-Guided Cancer Therapy. ACS Nano 11 (4), 3496–3505. doi:10.1021/acsnano.7b00133
Shao, J., Abdelghani, M., Shen, G., Cao, S., Williams, D. S., and van Hest, J. C. M. (2018). Erythrocyte Membrane Modified Janus Polymeric Motors for Thrombus Therapy. ACS Nano 12 (5), 4877–4885. doi:10.1021/acsnano.8b01772
Shi, X., Zhang, Y., Tian, Y., Xu, S., Ren, E., Bai, S., et al. (2021). Multi‐Responsive Bottlebrush‐Like Unimolecules Self‐Assembled Nano‐Riceball for Synergistic Sono‐Chemotherapy. Small Methods 5 (3), 2000416. doi:10.1002/smtd.202000416
Su, J., Sun, H., Meng, Q., Yin, Q., Tang, S., Zhang, P., et al. (2016). Long Circulation Red-Blood-Cell-Mimetic Nanoparticles with Peptide-Enhanced Tumor Penetration for Simultaneously Inhibiting Growth and Lung Metastasis of Breast Cancer. Adv. Funct. Mater. 26 (8), 1243–1252. doi:10.1002/adfm.201504780
Su, J., Sun, H., Meng, Q., Zhang, P., Yin, Q., and Li, Y. (2017). Enhanced Blood Suspensibility and Laser-Activated Tumor-specific Drug Release of Theranostic Mesoporous Silica Nanoparticles by Functionalizing with Erythrocyte Membranes. Theranostics 7 (3), 523–537. doi:10.7150/thno.17259
Talwar, N., and Jaind, N. K. (1992). Erythrocyte Based Delivery System of Primaquine:in Vitrocharacterization. J. Microencapsulation 9 (3), 357–364. doi:10.3109/02652049209021250
Tang, J. C., Hanley, T., Lee, C.-H., Lu, T., Vankayala, R., Azubuogu, C., et al. (2022). Membrane Cholesterol Enrichment of Red Blood Cell-Derived Microparticles Results in Prolonged Circulation. ACS Appl. Bio Mater. 5 (2), 650–660. doi:10.1021/acsabm.1c01104
Thomas, X., and le Jeune, C. (2016). Erythrocyte Encapsulated L-Asparaginase (GRASPA) in Acute Leukemia. Int. J. Hematologic Oncol. 5 (1), 11–25. doi:10.2217/ijh-2016-0002
Vankayala, R., Mac, J. T., Burns, J. M., Dunn, E., Carroll, S., Bahena, E. M., et al. (2019). Biodistribution and Toxicological Evaluation of Micron- and Nano-Sized Erythrocyte-Derived Optical Particles in Healthy Swiss Webster Mice. Biomater. Sci. 7 (5), 2123–2133. doi:10.1039/c8bm01448e
Wang, C., Sun, X., Cheng, L., Yin, S., Yang, G., Li, Y., et al. (2014). Multifunctional Theranostic Red Blood Cells for Magnetic-Field-Enhanced In Vivo Combination Therapy of Cancer. Adv. Mater. 26 (28), 4794–4802. doi:10.1002/adma.201400158
Wang, D., Dong, H., Li, M., Cao, Y., Yang, F., Zhang, K., et al. (2018). Erythrocyte-Cancer Hybrid Membrane Camouflaged Hollow Copper Sulfide Nanoparticles for Prolonged Circulation Life and Homotypic-Targeting Photothermal/Chemotherapy of Melanoma. ACS Nano 12 (6), 5241–5252. doi:10.1021/acsnano.7b08355
Wang, P., Wang, X., Luo, Q., Li, Y., Lin, X., Fan, L., et al. (2019a). Fabrication of Red Blood Cell-Based Multimodal Theranostic Probes for Second Near-Infrared Window Fluorescence Imaging-Guided Tumor Surgery and Photodynamic Therapy. Theranostics 9 (2), 369–380. doi:10.7150/thno.29817
Wang, Y., Zhang, K., Qin, X., Li, T., Qiu, J., Yin, T., et al. (2019b). Biomimetic Nanotherapies: Red Blood Cell Based Core-Shell Structured Nanocomplexes for Atherosclerosis Management. Adv. Sci. 6 (12), 1900172. doi:10.1002/advs.201900172
Xia, Q., Zhang, Y., Li, Z., Hou, X., and Feng, N. (2019). Red Blood Cell Membrane-Camouflaged Nanoparticles: A Novel Drug Delivery System for Antitumor Application. Acta Pharmaceutica Sinica B 9 (4), 675–689. doi:10.1016/j.apsb.2019.01.011
Zhang, J., Gao, W., FangFang, R. H., Dong, A., and Zhang, L. (2015). Synthesis of Nanogels via Cell Membrane-Templated Polymerization. Small 11 (34), 4309–4313. doi:10.1002/smll.201500987
Zhang, K., Cao, Y., Kuang, Y., Liu, M., Chen, Y., Wang, Z., et al. (2016). Gd2O3 and GH Combined with Red Blood Cells to Improve the Sensitivity of Contrast Agents for Cancer Targeting MR Imaging. Biomater. Sci. 5, 46–49. doi:10.1039/c6bm00627b
Zhang, Y., Xia, Q., Wu, T., He, Z., Li, Y., Li, Z., et al. (2021). A Novel Multi-Functionalized Multicellular Nanodelivery System for Non-small Cell Lung Cancer Photochemotherapy. J. Nanobiotechnol 19 (1), 245. doi:10.1186/s12951-021-00977-3
Zhang, Y., Zhang, J., Chen, W., Angsantikul, P., Spiekermann, K. A., Fang, R. H., et al. (2017a). Erythrocyte Membrane-Coated Nanogel for Combinatorial Antivirulence and Responsive Antimicrobial Delivery against Staphylococcus Aureus Infection. J. Controlled Release 263, 185–191. doi:10.1016/j.jconrel.2017.01.016
Zhang, Z., Qian, H., Yang, M., Li, R., Hu, J., Li, L., et al. (2017b). Gambogic Acid-Loaded Biomimetic Nanoparticles in Colorectal Cancer Treatment. Ijn Vol. 12, 1593–1605. doi:10.2147/IJN.S127256
Zheng, D., Yu, P., Wei, Z., Zhong, C., Wu, M., and Liu, X. (2020). RBC Membrane Camouflaged Semiconducting Polymer Nanoparticles for Near-Infrared Photoacoustic Imaging and Photothermal Therapy. Nanomicro Lett. 12 (1), 94–17. doi:10.1007/s40820-020-0042910.1007/s40820-020-00429-x
Zhou, H., Fan, Z., Lemons, P. K., and Cheng, H. (2016). A Facile Approach to Functionalize Cell Membrane-Coated Nanoparticles. Theranostics 6 (7), 1012–1022. doi:10.7150/thno.15095
Keywords: red blood cells, erythrocytes, nanoerythrosomes, drug delivery systems, nanovesicles
Citation: Vincy A, Mazumder S, Amrita , Banerjee I, Hwang KC and Vankayala R (2022) Recent Progress in Red Blood Cells-Derived Particles as Novel Bioinspired Drug Delivery Systems: Challenges and Strategies for Clinical Translation. Front. Chem. 10:905256. doi: 10.3389/fchem.2022.905256
Received: 26 March 2022; Accepted: 08 April 2022;
Published: 27 April 2022.
Edited by:
Peilin Chen, Academia Sinica, TaiwanReviewed by:
Che-Ming Jack Hu, Academia Sinica, TaiwanCopyright © 2022 Vincy, Mazumder, Amrita, Banerjee, Hwang and Vankayala. This is an open-access article distributed under the terms of the Creative Commons Attribution License (CC BY). The use, distribution or reproduction in other forums is permitted, provided the original author(s) and the copyright owner(s) are credited and that the original publication in this journal is cited, in accordance with accepted academic practice. No use, distribution or reproduction is permitted which does not comply with these terms.
*Correspondence: Raviraj Vankayala, cnZhbmtheWFsYUBpaXRqLmFjLmlu
†These authors have contributed equally to this work
Disclaimer: All claims expressed in this article are solely those of the authors and do not necessarily represent those of their affiliated organizations, or those of the publisher, the editors and the reviewers. Any product that may be evaluated in this article or claim that may be made by its manufacturer is not guaranteed or endorsed by the publisher.
Research integrity at Frontiers
Learn more about the work of our research integrity team to safeguard the quality of each article we publish.