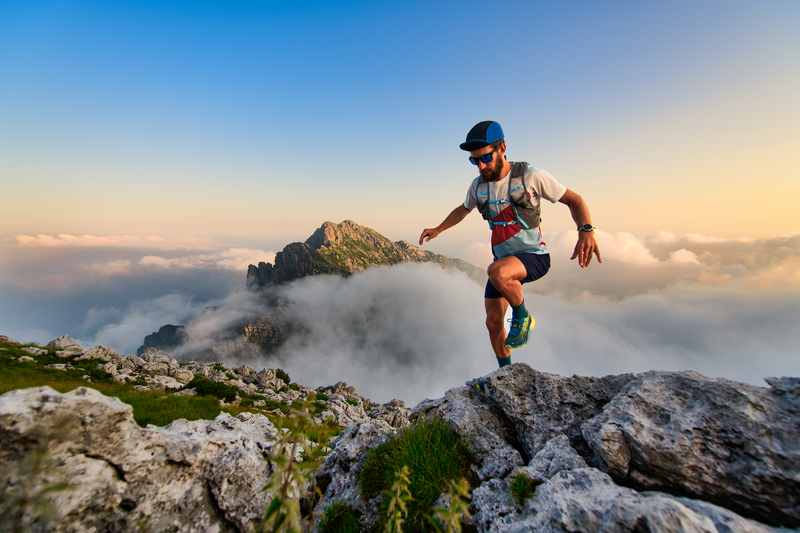
95% of researchers rate our articles as excellent or good
Learn more about the work of our research integrity team to safeguard the quality of each article we publish.
Find out more
ORIGINAL RESEARCH article
Front. Chem. , 29 April 2022
Sec. Electrochemistry
Volume 10 - 2022 | https://doi.org/10.3389/fchem.2022.904241
This article is part of the Research Topic Photo- and Electro-catalytic Materials for Energy Conversion and Pollutant Degradation View all 5 articles
Bimetallic materials are one of the most promising catalysts for the electrochemical reduction of CO2, but there are still many challenges to be overcome on the route to industrialization. Herein, a series of carbon nanofiber-supported bimetallic cobalt–copper catalysts (CoxCuy/CFs) are designed and constructed through the electrospinning technique and a subsequent pyrolysis procedure. Small-sized Co–Cu nanoparticles are homogenously distributed on the porous carbon nanofibers, which can significantly improve the utilization rate of metal sites and greatly reduce the loading amount of metals. Moreover, different product distributions and catalytic performance can be obtained in CO2 reduction via adjusting the metal proportion of CoxCuy/CFs. Especially, Co3Cu/CFs can bring forth a 97% total faradaic efficiency (FE) of CO (68%) and HCOOH (29%) at –0.8 VRHE cathode potential in 0.5 M KHCO3 electrolyte. Furthermore, the hierarchical pores can firmly confine the small Co–Cu nanoparticles and keep them from easy agglomeration during electrolysis, eventually leading to 60 h of stability for Co3Cu/CFs in CO2 electroreduction. This study might provide a facile and economic method to fabricate efficient bimetallic catalysts for CO2 electroreduction and other electrocatalysis applications.
CO2 electroreduction can convert greenhouse gas CO2 into renewable fuels and industrial building-block chemicals, which has been advocated as a promising candidate for the artificial carbon cycle (Qiao et al., 2014; Sharifian et al., 2021; Zhang et al., 2021). Electrochemical reduction of CO2 can be motivated by vast amounts of excess electricity from renewable energy resources, for example, wind, tide, and solar power plants (Benson et al., 2009; Fu et al., 2019). However, the CO2 molecule has a very high chemical stability; hence, appropriate catalysts are needed to activate them (Hori et al., 2008; Appel et al., 2013).
Therefore, a series of catalysts have been constructed to enhance the efficiency of CO2 electroreduction, including molecular catalysts (Appel and Helm, 2014; Nichols and Machan, 2019; and Bonin et al., 2017), heterodoped carbon catalysts (Sun, 2021; Kumar et al., 2013), oxide-derived catalysts (Duan et al., 2021; Duan et al., 2018), single-atom catalysts (Yang et al., 2019; Chen Jia et al., 2021; Wei et al., 2022; and Zhang et al., 2022), and multimetallic catalysts (Lin Jia et al., 2021; Vasileff et al., 2018; and Jia et al., 2022). Among these available catalysts, bimetallic catalysts have exhibited remarkable performance in CO2 reduction. Bimetallic materials not only change the electronic structures of the single component (Yang et al., 2021) but also create new active sites to regulate the binding energy of key intermediates during CO2 reduction (Jeoung and Dobbek, 2007; Yu et al., 2021; Cheng et al., 2021). Meanwhile, carbon materials (e.g., carbon black, graphite powder, and carbon nanotubes) are utilized as supports or carriers for bimetallic catalysts in actual electrolysis, which can improve the dispersion and conductivity (Jia et al., 2022). However, these carbon materials need pretreatment, including purification or surface functionalization, which might damage the porous structure and electrical conductivity (Liu et al., 2014). Therefore, it is still urgent to design a simple and effective approach to carbon supported with excellent activity in CO2 electroreduction.
In this study, we report the facile synthesis of several Co–Cu bimetallic catalysts, that is, Co–Cu bimetallic nanoparticles/porous carbon nanofiber (CoxCuy/CF) composites using the electrospinning technique and thermal treatment. In the composites, CoxCuy nanoparticles are uniformly and stably dispersed on the abundant poles of carbon nanofibers, rather than simply being absorbed or drop-coated on the surface. This structure can largely expose the CoxCuy nanoparticles onto the reaction interface of CO2 electroreduction and greatly improve the efficiency of electronic transmission. Furthermore, we also systematically investigated the effect of the mole ratio of Co and Cu components on the product distribution and faradaic efficiency. The results indicated that the Co3Cu/CF catalyst with a mole ratio of 3:1 displayed an outstanding catalytic activity and long-term stability in CO2 electroreduction.
All reagents were used as received without further purification.
Electrochemical tests were performed with a CHI 760e electrochemical Station (Shanghai Chenhua Instruments Company). Gaseous products were detected by gas chromatography (Shimadzu, GC-2014c) with a flame ionization detector (FID) and a thermal conductivity detector (TCD). Liquid products were detected using a nuclear magnetic resonance spectrometer (NMR, Ascend 400, Bruker, Germany). The micromorphology, crystalline structure, and element mapping were obtained by a field emission scanning electron microscope (FE-SEM, FEI JEOL-7800F) and a high-resolution transmission electron microscope (HR-TEM, JEM-2100F). The metal amount in the as-synthesized catalyst was detected using inductively coupled plasma-optical emission spectrometry (ICP-OES, OPTIMA2100DV). N2 adsorption/desorption curves were achieved by a specific surface and porosity analyzer (Micromeritics ASAP 2460) and calculated using the Brunauer–Emmett–Teller (BET) equation. X-ray diffraction (XRD) patterns were recorded with an X-ray powder diffractometer (Rigaku MiniFlex 600) with Cu Kα radiation (k = 1.5406 Å). Raman spectra were acquired with a laser Raman spectrometer (LabRAM HR Evolution, HORBIA FRANCE SAS) with a 633-nm laser excitation. X-ray photoelectron spectra (XPS) were recorded on an X-ray photoelectron spectrometer (ThermoVG Scientific ESCALAB 250) with Al Kα X-ray as the source.
All the five samples in this study were prepared by electrospinning technology. The preparation steps are as follows: 7 ml of N, N-dimethylformamide, 0.5 g polyacrylonitrile (PAN), and 0.75 g ZIF-8 nanoparticles were put into a beaker and stirred until they were evenly mixed into a white viscous solution. Then, 0.2183 g of Co(NO3)2·6H2O (0.00075 mol) and 0.061 g of Cu(NO3)2·3H2O (0.00025 mol) were added, and stirring was continued for at least 20 h or until the mixture was fully mixed to obtain a purple viscous spinning precursor solution. This precursor solution was injected into the syringe and electrospun to polymer fibers. After spinning, the polymer fibers were put into vacuum drying oven at 60°C for at least 12 h, and the dried polymer fiber was pre-oxidized in a muffle furnace. Then those pre-oxidized fibers were carbonized in nitrogen atmosphere. The initial temperature was set at 25°C, raised to 900°C at the rate of 5°C/min, and maintained for another 2 h. The as-synthesized catalyst was named as Co3Cu/CFs.
Another four catalysts with different metal ratios can be obtained by changing the molar ratio of metal precursors Co (NO3)2·6H2O and Cu (NO3)2·3H2O, including 1/0, 1/1, 1/3, and 0/1. The as-prepared samples were named as Co/CFs, CoCu/CFs, CoCu3/CFs, and Cu/CFs.
All the five catalysts were powdered and drop-coated onto a carbon paper (SGL Carbon Corporate) to get a useful working electrode. CO2 reduction activity was tested in a typical H-type electrochemical cell separated by an anion exchange membrane between anodic and cathodic chambers, with a Pt foil as the counter electrode and an Ag/AgCl as the reference electrode; 0.5 M KHCO3 solution was employed as the electrolyte and bubbled with high purity CO2 or N2 (99.995%). The original potentials measured in this manuscript were converted to the reversible hydrogen electrode (RHE) via the Nernst equation:
Products from CO2 reduction were analyzed at various cathodic potentials with a fixed time of 15 min, and the gaseous components were directly injected into gas chromatography. The liquid-phase products were detected via 1H NMR spectra. The Faraday efficiencies of the products were calculated via the following equations. Q is the total charge transferred through the working electrode at different potentials; m is the number of electrons transferred, which is 2 for HCOOH, CO, and H2, and 8 for CH4; n is the mole numbers of products; and F is the Faradaic constant (96,485 C mol−1).
The specific preparation process of the material is described in Figure 1. First, Co(NO3)2·6H2O, Cu(NO3)2·3H2O, ZIF-8 nanoparticles as well as PAN were dissolved in DMF to prepare a precursor solution, and then an electrospinning technology was used under constant conditions to get PAN nanofibers with different Co/Cu mole ratios. Then, the Co–Cu/PAN nanofibers were heated to 900°C under a N2 atmosphere for carbonization. Notably, there are no extra surfactants or reductants involved in the whole procedure. The polymer linkers were pyrolyzed and carbonized to generate the main body of carbon nanofibers, and ZIF-8 nanoparticles collapsed to form the abundant mesopores and macropores through these nanofibers (Yang et al., 2020a). Co2+ and Cu2+ ions were reduced by organic linkers, and the bimetallic nanoparticles were engendered with a smaller particle size due to the confinement of the polymer and ZIF-8 nanoparticles. The compositions of these bimetallic nanoparticles were tuned by the feeding ratio of Co(NO3)2·6H2O and Cu(NO3)2·3H2O precursors, eventually generating Co3Cu/CFs, Co/CFs, CoCu/CFs, CoCu3/CFs, and Cu/CFs.
As shown in Figure 2, the surface morphology and nanostructure of the as-synthesized Co3Cu/CF catalyst were recorded by FE-SEM and HR-TEM, respectively. The diameter of the carbon nanofibers in Co3Cu/CFs ranges from 500 to 600 nm. The length is in the scale of hundreds of micrometers, and the interlaced nanofibers furtherly form a network structure (Figures 2A,B). Moreover, abundant hollow pores, in the size range of dozens of nanometers, could be easily seen throughout Co3Cu/CFs (Figures 2C,D). N2 sorption isotherms (Figure 3A) further demonstrate that Co3Cu/CFs have type Ⅳ sorption isotherm, belonging to the mesoporous structure. Co–Cu nanoparticles, in an ∼20 nm diameter range, are evenly immobilized within the hollow pores of carbon nanofibers (Figures 2D,E).
FIGURE 2. (A–C) SEM and (D–E) TEM images with different resolutions of Co3Cu/CFs, respectively; (F) HR-TEM images of Co3Cu/CFs: inset shows the lattice fringes of Co and Cu; (G) HAADF-STEM and elemental mapping images of Co3Cu/CFs.
FIGURE 3. (A) N2 sorption isotherms of Co3Cu/CFs: inset displays the pore size distribution; (B) XRD patterns, (C) Raman spectra, and (D) XPS survey spectra of the five catalysts; (E) Co 2p and (F) Cu 2p fine XPS spectra of Co3Cu/CFs.
In addition, Figure 2F shows the clear HR-TEM image of an independent Co–Cu bimetallic nanoparticle, and the interplanar spacing of crystalline lattices marked with red lines is measured as 2.04 Å and 2.46 Å, corresponding to the Co (111) and Cu (111) planes (Liu et al., 2014; Kim et al., 2017), respectively. Furthermore, the high-angle annular dark field STEM (HAADF-STEM) and elemental mapping images indicate that Co3Cu/CFs contain Co and Cu elements. The good match between the emerging positions of Co and Cu elements directly proves the formation of Co–Cu bimetallic nanoparticles. According to the SEM, HR-TEM (Supplementary Figure S1,S2), and N2 sorption isotherms (Supplementary Figure S3, Supplementary Table S1), Co/CFs, CoCu/CFs, CoCu3/CFs, and Cu/CFs display similar interlaced nanofibers, abundant hollow pores, and uniform nanoparticles. Hence, the regulation of metal ratio would not significantly change the surface morphology and nanostructure. The actual Co/Cu ratios in the as-synthesized catalyst were detected by ICP-OES. CoCu/CFs, Co3Cu/CFs, and CoCu3/CFs own Co/Cu ratios of 1/0.95, 3/0.96, and 0.98/3, respectively, which are close to the original ratios in precursor solutions.
As shown in the XRD patterns (Figure 3B), the sharp diffraction peaks at 44.9°, 52.5°, and 76.2° can be seen in Co/CF samples, which are attributed to the Co (111), (200), and (220) planes (JCPDS 89-7093), respectively. Those peaks at 43.3°, 50.5°, and 74.2° of the Cu/CF samples are in accordance with the Cu (111), (200), and (220) planes (JCPDS 89-7093), respectively. The XRD patterns of Co3Cu/CFs, CoCu/CFs, and CoCu3/CFs possess both Co and Cu diffraction peaks, further verifying the formation of Co–Cu bimetallic nanoparticles. The Raman spectra (Figure 3C) of the five samples contain characteristic peaks around 1,350 cm−1 and 1,578 cm−1, related to the d band of defective carbon and the G band of graphite carbon (Kumar et al., 2013), respectively. Co3Cu/CFs have the largest intensity ratio of D and G bands (ID/IG, 1.01), indicating more defects and more potential active sites. The survey XPS spectra of Co3Cu/CFs (Figure 3D, Supplementary Figure S4) confirm the presence of Co, Cu, C, N, and O elements. The high-resolution Co 2p XPS spectrum of Co3Cu/CFs (Figure 3E) exhibits four peaks, including Co 2p3/2 (780.3 eV), Co 2p1/2 (795.6 eV), and two Co satellite peaks. Two strong peaks in the Cu 2p XPS spectrum (Figure 2F) that appear at 932.3 and 952.0 eV can be indexed to Cu 2p3/2 and Cu 2p1/2, respectively. Notably, oxide peaks can be detected in Co and Cu 2p XPS spectra of Co3Cu/CFs because of the easy oxidation of metallic Co and Cu nanoparticles (Xie et al., 2021; Kim et al., 2017). Similar C, N, Co, or Cu species could also be observed in the XPS spectra of another four samples (Supplementary Figure S5–S8).
The CO2 electroreduction performances of Co–Cu bimetallic catalysts were investigated and compared with the performance of pure Co or Cu catalysts in a typical H-type electrochemical cell. All the catalysts were pre-activated using cyclic voltammetry until stable profiles were obtained. Figure 4A and Supplementary Figure S9 present the linear sweep voltammetry (LSV) curves of five samples in N2-saturated or CO2-saturated 0.5 M KHCO3. The cathodic current densities of all five catalysts were measured to be approximately doubled in CO2 than those in the N2-saturated electrolyte, indicating the potential catalytic activity in CO2 reduction (Zhang et al., 2014). In addition, LSV tests also prove that Co3Cu/CFs show the highest current density among the five catalysts (Figure 4A).
FIGURE 4. (A) LSV curves of the five samples recorded in a CO2-saturated 0.5 M KHCO3 electrolyte; Faradaic efficiencies of (B) Co/CFs, (C) Co3Cu/CFs, and (D) Cu/CFs in a 0.5 M KHCO3 electrolyte; (E) C1 product partial current densities of the five samples; (F) long-term tests of Co3Cu/CFs at −0.8 VRHE in a 0.5 M KHCO3 electrolyte.
In order to further evaluate the catalytic activities and quantify the product distribution from CO2 electroreduction, potential dependent CO2 electrolysis using five catalysts was conducted in a 0.5 M KHCO3 electrolyte from –0.5 VRHE to –1.2 VRHE applied cathode potential. Gas products were directly injected into gas chromatography for on-line analysis, and liquid products in the catholyte were detected using 1H NMR after each electrolysis. The product distribution and faradaic efficiencies (FEs) are summarized in Figure 4 and Supplementary Figure S10. For comparison of the electrocatalytic activities, Co/CFs and Cu/CFs with pure Co and Cu nanoparticles were also synthesized and assessed in CO2 electrolysis. As shown in Figure 4B, Co/CFs generated CO as the only product from the CO2 reduction and H2 from the hydrogen evolution reaction (HER), with a 57% maximum FE for CO at –0.8 VRHE applied potential. As for Cu/CFs (Figure 4D), it produced H2, CO, and CH4 during electrolysis, with a 49% maximum FE for CO at –0.9 VRHE. CH4 is one of the urgent products with the transfer of eight electrons (Sharifian et al., 2021); however, highest FEs of CH4 only reach 7% at –0.8 VRHE. Both Co/CFs and Cu/CFs show certain activities in CO2 electroreduction, but neither of them could effectively suppress the HER process, and the FEs of H2 range from 43 to 74%. As displayed in Figure 4C and Supplementary Figure S10, doping Co with Cu causes a significant increment in CO2 catalysis. Compared to Co/CFs and Cu/CFs, the CO2 reduction procedure on Co3Cu/CFs, CoCu/CFs, and CoCu3/CFs became much more dominant than the HER procedure. In particular, the maximum FE for C1 production (HCOOH and CO) increases to 97% at –0.8 VRHE using the Co3Cu/CFs catalyst, and the HER is totally suppressed to only a 3% FE of H2. CoCu/CFs and CoCu3/CFs have a similar tendency of FEs for CO2 electrolysis as that of Co3Cu/CFs, but they acquire lower total FEs of CO and HCOOH throughout the applied potential.
Chronoamperometry (CA) was used to evaluate the total current density during CO2 electrolysis, and five samples achieved very close total current densities from –0.5 VRHE to –1.2 VRHE (Supplementary Figure S11). In addition, the partial current densities (jC1) for C1 products (CO, HCOOH, and CH4) were normalized by the total current densities and FEs at each cathode potential. Co3Cu/CFs brought forth a significantly higher partial current density than Co/CFs, Cu/CFs, CoCu/CFs, and CoCu3/CFs within the potential range, and got a maximum jC1 of 78.1 mA cm−2 at –1.2 VRHE (Figure 4E). Therefore, Co3Cu/CFs possess remarkable catalytic activity in CO2 reduction, and they can also successfully suppress the HER procedure at relatively high cathode potentials. Moreover, it is extremely important to estimate the long-term durability of bimetallic catalysts because increasing the applied potentials and heavy current densities might seriously impact the structural stability (Vasileff et al., 2018; Jia et al., 2022). Long-term tests of potentiostatic CO2 electrolysis were conducted using Co3Cu/CFs catalysts at –0.8 VRHE cathode potential where the best FEs of C1 products were obtained. The gaseous products were detected on-line every 6 h, and the corresponding CO FEs and current densities versus time are plotted in Figure 4F. Both CO FEs and partial current densities of the Co3Cu/CF catalyst exhibited only small declines during the 60 h electrolysis, retaining approximately 90% of the original values and manifesting excellent stability in CO2 electroreduction. Co3Cu/CFs were characterized after a long-term electrolysis by TEM (Supplementary Figure S12), and the small Co3Cu/CFs did not agglomerate together. As described previously (Figures 2D,E), the Co–Cu bimetallic nanoparticles are uniformly dispersed and firmly confined within the hierarchical pores of carbon nanofibers, and separated from easy agglomeration during electrolysis.
The aforementioned experimental results demonstrate the outstanding activities of Co3Cu/CFs in CO2 reduction. The mechanism of the high performance was first investigated using Tafel slopes within sufficiently low overpotential ranges. As presented in Figure 5A, 105 mV dec−1 Tafel value is observed on Co3Cu/CFs, confirming the first electron transfer from CO2 to CO2•− as the rate determining step (Chen Jia et al., 2021). Compared to those of Co/CFs (108 mV dec−1), Cu/CFs (127 mV dec−1), CoCu/CFs (111 mV dec−1), and CoCu3/CFs (137 mV dec−1), the lower Tafel value of Co3Cu/CFs indicates faster reaction kinetics in CO2 reduction. As mentioned previously, four electron product CH4 was only obtained with the Cu/CF catalyst, and the bimetallic CoxCuy/CFs could produce CO and HCOOH. Metallic Cu owns relatively strong binding energies of *COOH and *CO intermediates compared to pure metallic Co, and these intermediates could be stabilized and further reduced to hydrocarbons or alcohols (Duan et al., 2018; Mun et al., 2019). In CoxCuy/CF samples, the catalytic behavior of metallic Cu was totally altered via fusing it with Co composition within the same nanoparticles. The binding energies of *COOH and *CO intermediates were weakened enough to be released from the catalyst surface, increasing the tendency toward HCOOH and CO production.
FIGURE 5. (A) Tafel plots of five samples during the CO2 electroreduction process; (B) EIS Nyquist spectra of the five samples; (C) catalytic mechanism of Co3Cu/CFs for the reduction of CO2 to CO and HCOOH.
The electrochemical active surface area and the conductivity property of these five samples were also measured via double-layer capacitance (Cdl) and electrochemical impedance spectroscopy (EIS). Compared with Co/CFs (31.5 mF cm−2), Cu/CFs (17.5 mF cm−2), CoCu/CFs (30.0 mF cm−2), and CoCu3/CFs (21.5 mF cm−2), Co3Cu/CFs have a much higher Cdl value of 45.5 mF cm−2 (Supplementary Figure S13), manifesting the larger ECSA and more active sites for CO2 reduction (Yang et al., 2020b; Hao et al., 2022). In addition, the EIS curves in Figure 5B prove that Co3Cu/CFs show a relatively small impedance than those of the other samples, which is beneficial to faster electron transport as well as better conductivity (Wei et al., 2022; Liu et al., 2014). The larger ECSA and good conductivity of Co3Cu/CFs is consistent with its higher current densities in LSV and electrolysis tests. As illustrated in Figure 5C, Co3Cu/CFs possess highly graphitized and multi-level porous carbon nanofibers, which can accelerate the electron transmission and expose abundant bimetallic Co–Cu sites for CO2 reduction, eventually leading to the remarkable partial current densities for C1 products.
In summary, an efficient Co3Cu/CF catalyst was created with bimetallic Co–Cu nanoparticles evenly distributed within porous carbon nanofibers, which exhibited superior catalytic activities in CO2 reduction. A total 97% total faradaic efficiency of CO and HCOOH could be achieved with the Co3Cu/CFs catalyst at –0.8 VRHE cathode potential in a 0.5 M KHCO3 electrolyte. In addition, Co3Cu/CFs could also bring forth a maximum 78.1 mA cm−2 partial current density for C1 production and maintain 60-h of stability in long-term electrolysis. In Co3Cu/CFs catalysts, the doping of metallic Cu with Co can decrease the binding energies of key intermediates and increase the selectivity of CO and HCOOH. Moreover, the hierarchically porous carbon nanofibers are in favor of electron transmission and exposing active sites for CO2 electroreduction. Consequently, this effective strategy of composition tuning along with a tailored structure might inspire the design and preparation of robust catalysts for CO2 electroreduction.
The original contributions presented in the study are included in the article/Supplementary Material, further inquiries can be directed to the corresponding authors.
All authors listed have made a substantial, direct, and intellectual contribution to the work and approved it for publication.
This work was supported by the National Natural Science Foundation of China (22172099, 21975162, and 51902209), the Natural Science Foundation of Guangdong Province (2020A1515010840), and Shenzhen Science and Technology Program (Grant Nos. JCYJ20200109105803806, RCYX20200714114535052, RCBS20200714114819161, and JCYJ20190808111801674).
The authors declare that the research was conducted in the absence of any commercial or financial relationships that could be construed as a potential conflict of interest.
All claims expressed in this article are solely those of the authors and do not necessarily represent those of their affiliated organizations, or those of the publisher, the editors, and the reviewers. Any product that may be evaluated in this article, or claim that may be made by its manufacturer, is not guaranteed or endorsed by the publisher.
The Supplementary Material for this article can be found online at: https://www.frontiersin.org/articles/10.3389/fchem.2022.904241/full#supplementary-material
Appel, A. M., Bercaw, J. E., Bocarsly, A. B., Dobbek, H., DuBois, D. L., Dupuis, M., et al. (2013). Frontiers, Opportunities, and Challenges in Biochemical and Chemical Catalysis of CO2 Fixation. Chem. Rev. 113 (8), 6621–6658. doi:10.1021/cr300463y
Appel, A. M., and Helm, M. L. (2014). Determining the Overpotential for a Molecular Electrocatalyst. ACS Catal. 4, 630–633. doi:10.1021/cs401013v
Benson, E. E., Kubiak, C. P., Sathrum, A. J., and Smieja, J. M. (2009). Electrocatalytic and Homogeneous Approaches to Conversion of CO2to Liquid Fuels. Chem. Soc. Rev. 38, 89–99. doi:10.1039/B804323J
Bonin, J., Maurin, A., and Robert, M. (2017). Molecular Catalysis of the Electrochemical and Photochemical Reduction of CO2 with Fe and Co Metal Based Complexes. Recent Advances. Coord. Chem. Rev. 334, 184–198. doi:10.1016/j.ccr.2016.09.005
Chen Jia, C., Li, S., Zhao, Y., Hocking, R. K., Ren, W., Chen, X., et al. (2021). Nitrogen Vacancy Induced Coordinative Reconstruction of Single‐Atom Ni Catalyst for Efficient Electrochemical CO 2 Reduction. Adv. Funct. Mater. 31, 2107072. doi:10.1002/adfm.202107072
Cheng, Z., Wang, X., Yang, H., Yu, X., Lin, Q., Hu, Q., et al. (2021). Construction of Cobalt-Copper Bimetallic Oxide Heterogeneous Nanotubes for High-Efficient and Low-Overpotential Electrochemical CO2 Reduction. J. Energ. Chem. 54, 1–6. doi:10.1016/j.jechem.2020.04.018
Duan, Y.-X., Meng, F.-L., Liu, K.-H., Yi, S.-S., Li, S.-J., Yan, J.-M., et al. (2018). Amorphizing of Cu Nanoparticles toward Highly Efficient and Robust Electrocatalyst for CO2 Reduction to Liquid Fuels with High Faradaic Efficiencies. Adv. Mater. 30, 1706194. doi:10.1002/adma.201706194
Duan, Y. X., Zhou, Y. T., Yu, Z., Liu, D. X., Wen, Z., Yan, J. M., et al. (2021). Boosting Production of HCOOH from CO 2 Electroreduction via Bi/CeO X. Angew. Chem. Int. Ed. 60, 8798–8802. doi:10.1002/anie.202015713
Fu, H.-C., You, F., Li, H.-R., and He, L.-N. (2019). CO2 Capture and In Situ Catalytic Transformation. Front. Chem. 7, 525. doi:10.3389/fchem.2019.00525
Hao, Q., Liu, D.-X., Deng, R., and Zhong, H.-X. (2022). Boosting Electrochemical Carbon Dioxide Reduction on Atomically Dispersed Nickel Catalyst. Front. Chem. 9, 837580. doi:10.3389/fchem.2021.837580
Hori, Y., Vayenas, C. G., White, R. E., and Gamboa-Aldeco, M. E. (2008). Electrochemical CO2 Reduction on Metal Electrodes. New York: Springer, 89–189. doi:10.1007/978-0-387-49489-0_342
Jeoung, J.-H., and Dobbek, H. (2007). Carbon Dioxide Activation at the Ni,Fe-Cluster of Anaerobic Carbon Monoxide Dehydrogenase. Science 318, 1461–1464. doi:10.1126/science.1148481
Jia, Y., Li, F., Fan, K., and Sun, L. (2022). Cu-based Bimetallic Electrocatalysts for CO2 Reduction. Adv. Powder Mater. 1, 100012. doi:10.1016/j.apmate.2021.10.003
Kim, D., Xie, C., Becknell, N., Yu, Y., Karamad, M., Chan, K., et al. (2017). Electrochemical Activation of CO2 through Atomic Ordering Transformations of AuCu Nanoparticles. J. Am. Chem. Soc. 139, 8329–8336. doi:10.1021/jacs.7b03516
Kumar, B., Asadi, M., Pisasale, D., Sinha-Ray, S., Rosen, B. A., Haasch, R., et al. (2013). Renewable and Metal-free Carbon Nanofibre Catalysts for Carbon Dioxide Reduction. Nat. Commun. 4, 2819. doi:10.1038/ncomms3819
Lin Jia, L., Sun, M., Xu, J., Zhao, X., Zhou, R., Pan, B., et al. (2021). Phase‐Dependent Electrocatalytic CO 2 Reduction on Pd 3 Bi Nanocrystals. Angew. Chem. Int. Ed. 60, 21741–21745. doi:10.1002/anie.202109288
Liu, D., Guo, Q., Hou, H., Niwa, O., and You, T. (2014). PdxCoy Nanoparticle/Carbon Nanofiber Composites with Enhanced Electrocatalytic Properties. ACS Catal. 4, 1825–1829. doi:10.1021/cs5000153
Mun, Y., Lee, S., Cho, A., Kim, S., Han, J. W., and Lee, J. (2019). Cu-Pd alloy Nanoparticles as Highly Selective Catalysts for Efficient Electrochemical Reduction of CO2 to CO. Appl. Catal. B: Environ. 246, 82–88. doi:10.1016/j.apcatb.2019.01.021
Nichols, A. W., and Machan, C. W. (2019). Secondary-Sphere Effects in Molecular Electrocatalytic CO2 Reduction. Front. Chem. 7, 397. doi:10.3389/fchem.2019.00397
Qiao, J., Liu, Y., Hong, F., and Zhang, J. (2014). A Review of Catalysts for the Electroreduction of Carbon Dioxide to Produce Low-Carbon Fuels. Chem. Soc. Rev. 43, 631–675. doi:10.1039/C3CS60323G
Sharifian, R., Wagterveld, R. M., Digdaya, I. A., Xiang, C., and Vermaas, D. A. (2021). Electrochemical Carbon Dioxide Capture to Close the Carbon Cycle. Energy Environ. Sci. 14, 781–814. doi:10.1039/D0EE03382K
Sun, X. (2021). Achieving Selective and Efficient Electrocatalytic Activity for CO2 Reduction on N-Doped Graphene. Front. Chem. 9, 734460. doi:10.3389/fchem.2021.734460
Vasileff, A., Xu, C., Jiao, Y., Zheng, Y., and Qiao, S.-Z. (2018). Surface and Interface Engineering in Copper-Based Bimetallic Materials for Selective CO2 Electroreduction. Chem 4, 1809–1831. doi:10.1016/j.chempr.2018.05.001
Wei, S., Jiang, X., He, C., Wang, S., Hu, Q., Chai, X., et al. (2022). Construction of Single-Atom Copper Sites with Low Coordination Number for Efficient CO2 Electroreduction to CH4. J. Mater. Chem. A. 10, 6187–6192. doi:10.1039/D1TA08494A
Xie, L., Yu, X., Wang, S., Wei, S., Hu, Q., Chai, X., et al. (2021). A Multiscale Strategy to Construct Cobalt Nanoparticles Confined within Hierarchical Carbon Nanofibers for Efficient CO 2 Electroreduction. Small 18, 2104958. doi:10.1002/smll.202104958
Yang, H., Wu, Y., Li, G., Lin, Q., Hu, Q., Zhang, Q., et al. (2019). Scalable Production of Efficient Single-Atom Copper Decorated Carbon Membranes for CO2 Electroreduction to Methanol. J. Am. Chem. Soc. 141, 12717–12723. doi:10.1021/jacs.9b04907
Yang, H., Lin, Q., Wu, Y., Li, G., Hu, Q., Chai, X., et al. (2020a). Highly Efficient Utilization of Single Atoms via Constructing 3D and Free-Standing Electrodes for CO2 Reduction with Ultrahigh Current Density. Nano Energy 70, 104454. doi:10.1016/j.nanoen.2020.104454
Yang, H., Yu, X., Shao, J., Liao, J., Li, G., Hu, Q., et al. (2020b). In Situ encapsulated and Well Dispersed Co3O4 Nanoparticles as Efficient and Stable Electrocatalysts for High-Performance CO2 Reduction. J. Mater. Chem. A. 8, 15675–15680. doi:10.1039/d0ta03770b
Yang, N., Chen, D., Cui, P., Lu, T., Liu, H., Hu, C., et al. (2021). Heterogeneous Nanocomposites Consisting of Pt 3 Co alloy Particles and CoP 2 Nanorods towards High‐efficiency Methanol Electro‐oxidation. SmartMat 2, 234–245. doi:10.1002/smm2.1032
Yu, D., Gao, L., Sun, T., Guo, J., Yuan, Y., Zhang, J., et al. (2021). Strain-Stabilized Metastable Face-Centered Tetragonal Gold Overlayer for Efficient CO2 Electroreduction. Nano Lett. 21, 1003–1010. doi:10.1021/acs.nanolett.0c04051
Zhang, S., Kang, P., and Meyer, T. J. (2014). Nanostructured Tin Catalysts for Selective Electrochemical Reduction of Carbon Dioxide to Formate. J. Am. Chem. Soc. 136 (5), 1734–1737. doi:10.1021/ja4113885
Zhang, J., Sewell, C. D., Huang, H., and Lin, Z. (2021). Closing the Anthropogenic Chemical Carbon Cycle toward a Sustainable Future via CO 2 Valorization. Adv. Energ. Mater. 11, 2102767. doi:10.1002/aenm.202102767
Keywords: bimetallic catalysts, copper–cobalt bimetal, carbon nanofibers, CO2 reduction, Electrocatalysis
Citation: He C, Wang S, Jiang X, Hu Q, Yang H and He C (2022) Bimetallic Cobalt–Copper Nanoparticle-Decorated Hollow Carbon Nanofibers for Efficient CO2 Electroreduction. Front. Chem. 10:904241. doi: 10.3389/fchem.2022.904241
Received: 25 March 2022; Accepted: 04 April 2022;
Published: 29 April 2022.
Edited by:
Jie Zeng, University of Science and Technology of China, ChinaReviewed by:
Zhigang Geng, University of Science and Technology of China, ChinaCopyright © 2022 He, Wang, Jiang, Hu, Yang and He. This is an open-access article distributed under the terms of the Creative Commons Attribution License (CC BY). The use, distribution or reproduction in other forums is permitted, provided the original author(s) and the copyright owner(s) are credited and that the original publication in this journal is cited, in accordance with accepted academic practice. No use, distribution or reproduction is permitted which does not comply with these terms.
*Correspondence: Hengpan Yang, aHB5YW5nQHN6dS5lZHUuY24=; Chuanxin He, aGVjeEBzenUuZWR1LmNu
Disclaimer: All claims expressed in this article are solely those of the authors and do not necessarily represent those of their affiliated organizations, or those of the publisher, the editors and the reviewers. Any product that may be evaluated in this article or claim that may be made by its manufacturer is not guaranteed or endorsed by the publisher.
Research integrity at Frontiers
Learn more about the work of our research integrity team to safeguard the quality of each article we publish.