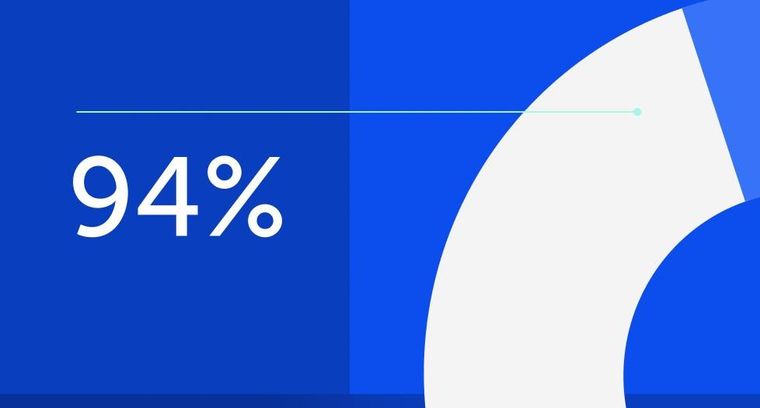
94% of researchers rate our articles as excellent or good
Learn more about the work of our research integrity team to safeguard the quality of each article we publish.
Find out more
ORIGINAL RESEARCH article
Front. Chem., 09 May 2022
Sec. Green and Sustainable Chemistry
Volume 10 - 2022 | https://doi.org/10.3389/fchem.2022.900482
This article is part of the Research TopicSustainable Catalytic Production of Bio-Based Heteroatom-Containing Compounds - Volume IIView all 21 articles
In this study, a novel sulfonic acid-modified catalyst for MOFs (UIO-66-SO3H) was synthesized using chlorosulfonic acid as a sulfonating reagent and first used as efficient heterogeneous catalysts for the one-pot conversion of fructose into biofuel 5-ethoxymethylfurfural (EMF) in a cosolvent free system. The physicochemical properties of this catalyst were characterized by Fourier transform infrared spectroscopy (FT-IR), transmission electron microscopy (TEM), and powder X-ray diffraction (XRD). The characterization demonstrated that the sulfonic acid group was successfully grafted onto the MOF material and did not cause significant changes to its morphology and structure. Furthermore, the effects of catalyst acid amount, reaction temperature, reaction time, and catalyst dosage on reaction results were investigated. The results showed that the conversion of fructose was 99.7% within 1 h at 140°C, while the EMF yield reached 80.4%. This work provides a viable strategy by application of sulfonic acid-based MOFs for the efficient synthesis of potential liquid fuel EMF from renewable biomass.
As the only renewable carbon resource with extensive distribution and abundant reserves in nature, biomass has become the most attractive potential energy to replace fossil fuels (Hu et al., 2015; Tang et al., 2019; Guo D. et al., 2020), especially in recent years, with the shortage of fossil resources and the environmental problems brought by the applications, the development of new methods for the conversion of biomass and its platform molecules into fuels and chemicals is a current priority (Liu et al., 2016; Venkata Mohan et al., 2016).
The products gained from biomass conversion mainly include polyols, furans, organic acids and their ester derivatives, short-chain alkanes, and other basic platform chemicals and new fuels. The large-scale utilization of these biomass conversion products will play a significant leading role in sustainable and healthy socio-economic development (Hommes et al., 2019; He 2021; Hoang and Pham, 2021). The acid-catalyzed conversion of biomass to biomass platform molecule 5-hydroxymethylfurfural (HMF) has been previously reported in some research and progress (Zhao et al., 2007; Guo H. et al., 2020; Liu et al., 2021; Yin et al., 2021), and its derivative 5-ethoxymethylfurfural (EMF) is also a potential fuel or fuel additive with excellent properties such as high energy density and low toxicity, with energy density up to 8.7 kWh/L, even higher than ethanol (6.1 kWh/L), and has good oxidation properties when used as a fuel (Chen et al., 2019a; Guo D. et al., 2020). Currently, the main synthesis pathway of EMF is based on biomass sugar compounds (e.g., glucose, fructose, and cellulose), which are converted to EMF in an ethanol system through acid catalysis (Chen et al., 2019b; Dai et al., 2019; Zhang et al., 2020). Among them, fructose exists in large quantities in the free state in the pulp of fruits and honey and is a common biomass raw material. As a reaction substrate for the generation of EMF, unlike glucose and cellulose, fructose has a strong crystal structure, is easily soluble in conventional organic solvents, and has a higher yield than glucose and cellulose (Zhang et al., 2019). Meanwhile, the acidic catalyst has a crucial role in the synthesis of the EMF reaction, making the conversion process more complete and efficient. The catalysts reported in previous studies are zeolite catalysts, sulfonic acid-type solid acid catalysts, and heteropolyacids (Li et al., 2016; Liu et al., 2016; Kumari et al., 2019; Xiang et al., 2021). In particular, sulfonic acid-type catalysts have high protonic acid strength, which is beneficial for the synthesis of EMF. For instance, Zhang et al (2018) reported sulfonated organic hyper-cross-linked polymer (HCP)-based carbohydrate catalysts to yield 78.9% EMF, 15.4% HMF, and 4.6% ethyl levulinate (EL) in an ethanol-dimethyl sulfoxide system with fructose as feedstock. Yan et al (2022) found sulfonic acid-based annealed functionally biobased carbon microspheres loading polytetrafluoroethylene (A-BCMSs-SO3H@PTFE) catalyst applied to the acid-catalyzed synthesis of liquid EMF. The yield of EMF could reach more than 36.6% after 72 h of the acid-catalyzed reaction. Manjunathan et al (2021) used the sulfonated hydrophobic mesoporous organic polymer (MOP-SO3H) in the production of EMF by the one-pot method using fructose in an ethanol solvent. The conversion of fructose was 99.3%, and the EMF yield reached 72.2% at 100°C for 5 h. These results show that loading acid-functionalized sulfonic acid groups onto the carrier can produce sulfonic acid-based solid acid catalysts to improve the efficiency of fructose conversion to EMF (Nakajima and Hara 2012). Therefore, finding a suitable, modified as well as stable performance carrier is a prerequisite for successful catalyst preparation.
Metal–organic framework (MOF) materials, developed rapidly in the last two decades, have a large ordered specific surface area, adjustable pore size, customizable functions, and a large number of active junctions (Herbst and Janiak 2017; Lv et al., 2019). As a new type of MOFs, UIO-66 has great stability and can exist stably in an acidic environment, and UIO-66 is a hydrophobic material with the molecular formula [Zr6(OH)4O4(BDC)6], which is a Zr6-cluster as the central structural unit and 12 terephthalic acid (H2BDC) as the ligand to form a three-dimensional topology of metal–organic backbone materials with the structure shown in Figure 1 (Guillerm et al., 2012; Olorunyomi et al., 2021). Also, UIO-66 is a hydrophobic material which is well suited for the conversion of biobased feedstock (Lv et al., 2019; Oozeerally et al., 2021). It also inherits the characteristics of ordinary MOF materials such as large specific surface area, adjustable pore size, and easy modification, which is a good catalyst supporter.
FIGURE 1. Structure of UIO-66 (Guillerm et al., 2012; Olorunyomi et al., 2021). Gray and red balls represent carbon and oxygen atoms, respectively. Zirconium octahedral is in green. Hydrogen atoms are omitted for clarity.
Based on the aforementioned details, in this study, we report a sulfonic acid-functionalized UIO-66-type MOFs (UIO-66-SO3H) prepared under mild conditions. The catalytic performance of these catalysts was investigated for selective preparation of EMF from fructose in the one-pot strategy. Also, the effects of reaction temperature, reaction time, catalyst dosage, and acid amount on the yield of EMF were also evaluated. In addition, the morphology and physicochemical properties of the catalysts were characterized and discussed.
Terephthalic acid was purchased from Aladdin Industrial Corporation. 5-Hydroxymethylfurfural (HMF, 98%), 5-ethoxymethylfurfural (EMF, 97%), ethyl levulinate (EL, 99%), and zirconium octahydrate oxychloride were purchased from Macklin Biochemical Co., Ltd. Ethanol (≥99.8%), glacial acetic acid, N, N-dimethylformamide (DMF), and methylene chloride were obtained from Sinopharm Chemical Reagent Co., Ltd. Chlorosulfonic acid was purchased from Shanghai Jiuding Chemical Reagent Co., Ltd.
In a 100-ml round-bottom flask, 4.8 g ZrOCl2·8H2O (15 mmol), 2.4 g terephthalic acid (15 mmol), 50 ml DMF, and 6 ml acetic acid was mixed, and the mixture was then treated with ultrasound for 30 min. After that, the resultant mixture was kept under constant stirring at 120°C for 24 h, and the obtained white precipitate was washed with methanol and DMF several times. After drying, the mashy white solids were calcined in a muffle furnace to give UIO-66 (Ragon et al., 2014).
The as-prepared UIO-66 (1 g) was dispersed in 40 ml CH2Cl2 and stirred at 0°C for 30 min. Under vigorous magnetic stirring, ClSO3H x mL in 10 ml CH2Cl2 was slowly added to the mixture. After the reaction continued for 4 h, the produced solid was filtered and washed with ethanol and then with deionized water and dried in a vacuum oven. The prepared catalysts are designated as UIO-66-SO3H-x (x = 0.3, 0.5, 0.8, and 1.0 ml), and x represents the volume of chlorosulfonic acid added.
The catalytic upgrading of fructose was carried out in a 50-ml autoclave with a Teflon liner. Typically, the Teflon liner was loaded with fructose (1 mmol, 0.180 g), ethanol (5 ml) as the reactant and solvent, and the UIO-66-SO3H catalyst. Then, the reaction mixture was heated to the prescribed temperature for a desired reaction time with stirring. After finishing the reaction, the reactor was given an ice bath for rapid cooling to room temperature.
Then, the mixture products were diluted and filtered using a 0.22-μm syringe filter. The fructose and the formed products were analyzed using an Agilent 1260 HPLC chromatograph equipped with refractive index and UV detectors. The external standard method was used to analyze the substrate conversion rate and selectivity of each product, and the calculation equation is as follows:
The FT-IR spectra of the metal–organic framework material UIO-66 and catalyst UIO-66-SO3H are shown in Figure 2, in which 1,585 cm−1 is the benzene ring skeleton stretching vibration peak; 1,504 cm−1 and 1,401 cm−1 are the absorption peaks corresponding to the structural unit (Zr6O4)(OH)4(CO2)n formed by coordination of terephthalic acid with zirconium nodes (Zaboon et al., 2018). The fingerprint region 745 cm−1 is the out-of-plane bending vibration of C-H; an absorption peak can be seen at 554 cm−1, which is the Zr-O bond stretching vibration peak in the metal–organic skeleton. In the IR curve of UIO-66-SO3H, 1,283 cm−1 and 1,090 cm−1 belong to the O=S=O symmetric stretching vibration peak and S-O stretching vibration peak, respectively; 628 cm−1 is the stretching vibration peak of the C-S bond (Chen T.-F. et al., 2019). Thus, the presence of sulfonic acid groups in the UIO-66-SO3H catalyst was confirmed, indicating the successful preparation of sulfonic acid-functionalized materials.
The XRD spectra of UIO-66 and UIO-66-SO3H are shown in Figure 3, in which the XRD spectrum of UIO-66 is consistent with that in the literature. Some XRD peaks of the UIO-66-SO3H catalyst were shifted, and the intensity of the peaks was significantly weakened compared with the UIO-66 sample, which is similar to the literature report. This was because during the introduction of strong Brønsted acidity into UIO-66, the chlorosulphonic acid impaired its crystallinity as well as the stability of the framework (Hu et al., 2016).
Figure 4 presents the transmission electron microscopy images of the samples, from which it can be observed that the morphology of both materials is similar and consist of particles of relatively uniform size. However, they are also in a more agglomerated state, which can be attributed to the particle size of the samples being around 50 nm, resulting in strong surface forces and agglomeration. The result of transmission electron microscopy revealed that the morphological characteristics of the UIO-66 material were hardly affected by the presence of the sulfonic acid group during the preparation of the catalyst.
In this study, ethanol was used as the reactant and reaction medium, and the reaction was carried out in the order of fructose dehydration to produce HMF and ethyl alcohol etherification to produce EMF, and the ring-opening by-product EL was also generated (Figure 5).
For the sulfonation of the prepared metal–organic framework UIO-66, the same mass of UIO-66 added with different amounts of the sulfonating agent resulted in different acid amounts of UIO-66-SO3H catalysts as listed in Table 1. The UIO-66 (1 g) added with the volume of chlorosulfonic acid increased from 0.3 to 1.0 ml, and the acid amount of the catalytic material showed a roughly increasing trend. The highest acid amount of the catalyst was measured at 0.8 ml of chlorosulfonic acid, and the best catalytic effect was achieved with UIO-66-SO3H showing a maximum acid amount of 1.46 mmol/g. To some extent, the acid amount of the catalyst increased with increasing concentration of the sulfonating agent. However, due to the gradual increase of the spatial site resistance and the damage to the catalyst structure caused by the addition of too much chlorosulfonic acid, further increase of the sulfonating agent did not increase the amount of acid, while the sulfonated materials were all effective in catalyzing the conversion of fructose to EMF. Accordingly, the effect of the acid amount of the catalyst on the catalytic activity is reflected in Figure 6. If the catalyst material was used without sulfonic acid groups, the target product is almost absent in the reaction solution, while the sulfonated materials were all effective in catalyzing the conversion of fructose to EMF. Thus, it is evident that the acidity of the catalyst is crucial for this reaction. With the increase of the acid amount, the conversion of fructose gradually increased, and the yield of EMF also showed an increasing trend, and it can be concluded that the acid amount is closely related to the synthesis of the target product EMF yield. Based on the aforementioned conclusions, the effect of factors such as reaction temperature, time, and catalyst amount on fructose alcoholysis of UIO-66-SO3H was further investigated systematically in this article.
FIGURE 6. Results of alcoholysis of fructose catalyzed by UIO-66-SO3H with different acidity. Reaction conditions: 1 mmol fructose, 5 ml ethanol, 30 mg catalyst, 1 h, and 140°C.
The effect of reaction temperature in the range of 110–150°C on the conversion of fructose to EMF was investigated using UIO-66-SO3H-0.8 as the catalyst, and the results are shown in Figure 7. As the reaction temperature was 110°C, the yield of both EMF and HMF was low, and a trace amount of EL was detected. With the increase in the reaction temperature, the fructose was gradually converted from 88.7% to complete conversion, indicating that the dehydration of fructose required higher temperature, and the etherification of HMF was easier than the dehydration of fructose into HMF. The yield of EMF increased significantly from 9.6 to 80.4% with the increase from 110 to 140°C, the yield of the intermediate product HMF decreased, and the byproduct EL showed a gradual increase. However, when the temperature was increased to 150°C, the yield of the target product EMF decreased due to the ring opening of the side reaction EMF, which might be due to the instability of EMF at higher reaction temperatures under acidic conditions, resulting in the generation of more ring-opening product EL. The increase in temperature was favorable to the conversion of fructose, and too high temperature promoted the decomposition of EMF into other products. Therefore, the optimal reaction temperature for this experiment was 140°C.
FIGURE 7. Effect of reaction temperature on the alcoholysis of fructose. Reaction conditions: 1 mmol fructose, 5 ml ethanol, 30 mg catalyst, and 1 h.
Figure 8 shows the effect of the reaction time of UIO-66-SO3H-0.8 as a catalyst on the synthesis of EMF from fructose in the ethanol system at a reaction temperature of 140°C. It can be seen that the target product EMF yield increased relatively fast at the beginning of the reaction stage, reaching 69% within 30 min, and as the reaction time continued to extend, the yield of EMF reached the best yield of 80.4% at 1 h, followed by a slight decrease in the yield of EMF. The content of HMF gradually decreased with the extension of time and was gradually converted into EMF, while the yield of EL gradually increased. This result indicates that fructose conversion is a typical continuous reaction in the ethanol reaction system. The longer reaction time allowed the conversion of EMF to its byproduct EL. Since the long time was not favorable for the production of EMF, a reaction time of 1 h was chosen for the subsequent reaction.
FIGURE 8. Effect of reaction time on the alcoholysis of fructose. Reaction conditions: 1 mmol fructose, 5 ml ethanol, 30 mg catalyst, and 140°C.
Under the conditions of 180 mg of substrate, 140°C of reaction temperature, and 1 h of reaction time, the effects of catalyst dosages of 10 mg, 20 mg, 30 mg, 40 mg, and 50 mg on the conversion of fructose to synthesize EMF were investigated. As seen in Figure 9, under the condition of 10 mg catalyst dosage, the fructose was almost completely converted, and the EMF yield was 36.1%. It can be observed in the figures that the increase of catalyst dosage from 10 to 20 mg increased the yield of EMF by about double, which was 72.5%. The highest EMF yield was obtained when the catalyst dosage was 30 mg, while the yield decreased slightly when the catalyst dosage was 40 mg and more. Therefore, 30 mg was chosen as the best catalyst dosage.
FIGURE 9. Effect of catalyst dosage on the alcoholysis of fructose. Reaction conditions: 1 mmol fructose, 5 ml ethanol, 1 h, and 140°C.
In addition, the possible mechanism for catalyzed conversion of fructose to EMF is shown in Figure 10. Fructose is dehydrated, dehydrogenated, and dehydrated to produce HMF under acidic conditions. Then, the acid site on the catalyst surface protonated with hydroxyl oxygen in HMF to form carbocation, followed by ethanol attack on carbocation, and finally formed EMF after proton migration (Amarasekara et al., 2008; Ohara et al., 2010; Yan et al., 2022).
FIGURE 10. Possible reaction mechanism for the conversion of fructose to EMF in ethanol catalyzed by UIO-66-SO3H(Amarasekara et al., 2008; Ohara et al., 2010; Yan et al., 2022).
The literature related to the one-step conversion of fructose to EMF is presented in Table 2, which compares the catalytic activity of the catalyst prepared in this study, UIO-66-SO3H, with other catalysts. For example, Dai et al. (2019) reported a solid organocatalyst, double hydrogen-bonded sulfonated polymer catalyst (D-SPC), to convert fructose to EMF in 18 h at 140°C with a maximum yield of 68.8%. Manjunathan et al., (2021) found a catalyst consisting of divinylbenzene (DVB) and sodium p-styrenesulfonate (SPSS) copolymerized with a sulfonated hydrophobic mesoporous organic polymer (MOP-SO3H) catalyst, which was able to achieve an EMF yield of 72.2% under the conditions of a bulk catalyst-catalyzed reaction. Bharath et al., (2021) immobilized the ultra-small Pd–Ru nanoparticles on the surface of 2D MXene nanosheets to produce a catalyst (Pd-Ru/MXene), which was reacted at 120°C for 2.5 h and was able to obtain a final EMF yield of 82%. Compared with the previous two catalysts, the catalyst prepared in this study has good catalytic effect. Meanwhile, the UIO-66-SO3H catalyst catalyzed the conversion of fructose to EMF in this experiment under lower conditions that were easily satisfied, and the reaction time was greatly reduced to achieve high catalytic effect.
TABLE 2. Catalytic performance of UIO-66-SO3H compared to reported solid acid catalysts for the EMF synthesis from fructose.
In summary, we prepared the MOF material UIO-66 and functionalized it using chlorosulfonic acid under mild conditions to produce a series of sulfonic acid-functionalized MOF-based solid catalysts (UIO-66-SO3H). The catalyst was characterized, and the corresponding results showed that the sulphonic acid group was successfully bonded to the benzene ring on the metal–organic framework. In addition, the acid amount of the catalyst could be adjusted by changing the amount of chlorosulfonic acid, and the highest acidity of the catalyst reached 1.46 mmol/g. The as-prepared heterogeneous catalyst UIO-66-SO3H showed efficient catalytic performance for the one-pot conversion of fructose to biofuel EMF under cosolvent-free conditions. The effect of different reaction conditions on the catalytic reaction was systematically investigated, and the EMF yield of 80.4% could be obtained under the optimum reaction conditions. Compared with those previously reported, the catalyst prepared in this work has the advantages of easy preparation, mild reaction conditions, and a good catalytic effect. It is expected that our catalytic system can be further applied for the efficient production of biofuel EMF in industrial applications.
The original contributions presented in the study are included in the article/Supplementary Material, further inquiries can be directed to the corresponding authors.
KZ: investigation, methodology, and writing–original draft preparation. YX: data curation and formal analysis. XS: validation. LC: methodology and visualization. JX: conceptualization, project administration, and writing–review and editing. XL: conceptualization, project administration, funding acquisition, and supervision.
The authors gratefully acknowledge the financial support of the National Natural Science Foundation of China (21606082), Scientific Research Fund of Hunan Provincial Education Department (20B364), and Science and Technology Planning Project of Hunan Province (2018TP1017, 2021RC1003).
The authors declare that the research was conducted in the absence of any commercial or financial relationships that could be construed as a potential conflict of interest.
All claims expressed in this article are solely those of the authors and do not necessarily represent those of their affiliated organizations, or those of the publisher, the editors, and the reviewers. Any product that may be evaluated in this article, or claim that may be made by its manufacturer, is not guaranteed or endorsed by the publisher.
Amarasekara, A. S., Williams, L. D., and Ebede, C. C. (2008). Mechanism of the Dehydration of D-Fructose to 5-hydroxymethylfurfural in Dimethyl Sulfoxide at 150°C: an NMR Study. Carbohydr. Res. 343, 3021–3024. doi:10.1016/j.carres.2008.09.008
Bharath, G., Rambabu, K., Hai, A., Morajkar, P. P., Salkar, A. V., Hasan, S. W., et al. (2021). Highly Selective Etherification of Fructose and 5‐hydroxymethylfurfural over a Novel Pd‐Ru/MXene Catalyst for Sustainable Liquid Fuel Production. Int. J. Energ. Res. 45, 14680–14691. doi:10.1002/er.6743
Chen, B., Xu, G., Chang, C., Zheng, Z., Wang, D., Zhang, S., et al. (2019b). Efficient One-Pot Production of Biofuel 5-Ethoxymethylfurfural from Corn Stover: Optimization and Kinetics. Energy Fuels 33, 4310–4321. doi:10.1021/acs.energyfuels.9b00357
Chen, B., Xu, G., Zheng, Z., Wang, D., Zou, C., and Chang, C. (2019a). Efficient Conversion of Corn stover into 5-ethoxymethylfurfural Catalyzed by Zeolite USY in Ethanol/THF Medium. Ind. Crops Prod. 129, 503–511. doi:10.1016/j.indcrop.2018.12.027
Chen, T.-F., Han, S.-Y., Wang, Z.-P., Gao, H., Wang, L.-Y., Deng, Y.-H., et al. (2019c). Modified UiO-66 Frameworks with Methylthio, Thiol and Sulfonic Acid Function Groups: The Structure and Visible-Light-Driven Photocatalytic Property Study. Appl. Catal. B: Environ. 259, 118047. doi:10.1016/j.apcatb.2019.118047
Dai, J., Liu, Z., Hu, Y., Liu, S., Chen, L., Qi, T., et al. (2019). Adjusting the Acidity of Sulfonated Organocatalyst for the One-Pot Production of 5-ethoxymethylfurfural from Fructose. Catal. Sci. Technol. 9, 483–492. doi:10.1039/c8cy02010h
Guillerm, V., Ragon, F., Dan-Hardi, M., Devic, T., Vishnuvarthan, M., Campo, B., et al. (2012). A Series of Isoreticular, Highly Stable, Porous Zirconium Oxide Based Metal-Organic Frameworks. Angew. Chem. Int. Ed. 51, 9267–9271. doi:10.1002/anie.201204806
Guo, D., Liu, X., Cheng, F., Zhao, W., Wen, S., Xiang, Y., et al. (2020a). Selective Hydrogenolysis of 5-hydroxymethylfurfural to Produce Biofuel 2, 5-dimethylfuran over Ni/ZSM-5 Catalysts. Fuel 274, 117853. doi:10.1016/j.fuel.2020.117853
Guo, H., Duereh, A., Su, Y., Hensen, E. J. M., Qi, X., and Smith, R. L. (2020b). Mechanistic Role of Protonated Polar Additives in Ethanol for Selective Transformation of Biomass-Related Compounds. Appl. Catal. B: Environ. 264, 118509. doi:10.1016/j.apcatb.2019.118509
He, X. (2021). A Novel Hybrid Digestion-Gasification Process Integrated with Membranes for Efficient Conversion of Biomass to Bio-Alcohols. Green. Energ. Environ. 6, 15–21. doi:10.1016/j.gee.2020.04.003
Herbst, A., and Janiak, C. (2017). MOF Catalysts in Biomass Upgrading towards Value-Added fine Chemicals. CrystEngComm 19, 4092–4117. doi:10.1039/c6ce01782g
Hoang, A.T, and Pham, V. (2021). 2-Methylfuran (MF) as a Potential Biofuel: A Thorough Review on the Production Pathway from Biomass, Combustion Progress, and Application in Engines. Renew. Sustain. Energ. Rev. 148, 111265. doi:10.1016/j.rser.2021.111265
Hommes, A., Heeres, H. J., and Yue, J. (2019). Catalytic Transformation of Biomass Derivatives to Value‐Added Chemicals and Fuels in Continuous Flow Microreactors. ChemCatChem 11, 4671–4708. doi:10.1002/cctc.201900807
Hu, L., Tang, X., Wu, Z., Lin, L., Xu, J., Xu, N., et al. (2015). Magnetic Lignin-Derived Carbonaceous Catalyst for the Dehydration of Fructose into 5-hydroxymethylfurfural in Dimethylsulfoxide. Chem. Eng. J. 263, 299–308. doi:10.1016/j.cej.2014.11.044
Hu, Z., Lin, J., Ogiwara, N., Rodriguez, A., Peng, Y., Wang, Y., et al. (2016). A pH-Responsive Phase Transformation of a Sulfonated Metal-Organic Framework from Amorphous to Crystalline for Efficient CO2capture. CrystEngComm 18, 2803–2807. doi:10.1039/c6ce00369a
Kumari, P. K., Rao, B. S., Dhana Lakshmi, D., Sai Paramesh, N. R., Sumana, C., and Lingaiah, N. (2019). Tungstophosphoric Acid Supported on Mesoporouus Niobiumoxophosphate: an Efficient Solid Acid Catalyst for Etherification of 5-hydroxymethylfurfural to 5-ethoxymethylfurfural. Catal. Today 325, 53–60. doi:10.1016/j.cattod.2018.06.047
Li, H., Saravanamurugan, S., Yang, S., and Riisager, A. (2016). Direct Transformation of Carbohydrates to the Biofuel 5-ethoxymethylfurfural by Solid Acid Catalysts. Green. Chem. 18, 726–734. doi:10.1039/c5gc01043h
Liu, S., Zheng, W., Wen, X., Fang, Z., Li, H., Li, C., et al. (2022). Molecular Design and Experimental Study of Cellulose Conversion to 5-hydroxymethylfurfural Catalyzed by Different Ratios of Brønsted/Lewis Acid Ionic Liquids. Carbohydr. Polym. 278, 118936. doi:10.1016/j.carbpol.2021.118936
Liu, X., Li, H., Pan, H., Zhang, H., Huang, S., Yang, K., et al. (2016). Efficient Catalytic Conversion of Carbohydrates into 5-ethoxymethylfurfural over MIL-101-Based Sulfated Porous Coordination Polymers. J. Energ. Chem. 25, 523–530. doi:10.1016/j.jechem.2016.01.015
Lv, S.-W., Liu, J.-M., Li, C.-Y., Ma, H., Wang, Z.-H., Zhao, N., et al. (2019). Fabrication of Fe3O4@UiO-66-So3h Core-Shell Functional Adsorbents for Highly Selective and Efficient Removal of Organic Dyes. New J. Chem. 43, 7770–7777. doi:10.1039/c9nj01324e
Manjunathan, P., Upare, P. P., Lee, M., and Hwang, D. W. (2021). One-pot Fructose Conversion into 5-ethoxymethylfurfural Using a Sulfonated Hydrophobic Mesoporous Organic Polymer as a Highly Active and Stable Heterogeneous Catalyst. Catal. Sci. Technol. 11, 5816–5826. doi:10.1039/d1cy00883h
Nakajima, K., and Hara, M. (2012). Amorphous Carbon with SO3H Groups as a Solid Brønsted Acid Catalyst. ACS Catal. 2, 1296–1304. doi:10.1021/cs300103k
Ohara, M., Takagaki, A., Nishimura, S., and Ebitani, K. (2010). Syntheses of 5-hydroxymethylfurfural and Levoglucosan by Selective Dehydration of Glucose Using Solid Acid and Base Catalysts. Appl. Catal. A: Gen. 383, 149–155. doi:10.1016/j.apcata.2010.05.040
Olorunyomi, J. F., Liu, T., Ho, C.-K., Li, C.-Y. V., and Chan, K.-Y. (2021). Imparting UiO-66 with Fast Cation Exchange Property via Sulfonating Organic Linkers for Selective Adsorption. Separat. Purif. Technol. 260, 118219. doi:10.1016/j.seppur.2020.118219
Oozeerally, R., Burnett, D. L., Chamberlain, T. W., Kashtiban, R. J., Huband, S., Walton, R. I., et al. (2021). Systematic Modification of UiO‐66 Metal‐Organic Frameworks for Glucose Conversion into 5‐Hydroxymethyl Furfural in Water. ChemCatChem 13, 2517–2529. doi:10.1002/cctc.202001989
Ragon, F., Horcajada, P., Chevreau, H., Hwang, Y. K., Lee, U.-H., Miller, S. R., et al. (2014). In Situ energy-dispersive X-ray Diffraction for the Synthesis Optimization and Scale-Up of the Porous Zirconium Terephthalate UiO-66. Inorg. Chem. 53, 2491–2500. doi:10.1021/ic402514n
Tang, Y., Cheng, Y., Xu, H., Wang, Y., Ke, L., Huang, X., et al. (2019). Binary Oxide Nanofiber Bundle Supported Keggin-type Phosphotungstic Acid for the Synthesis of 5-hydroxymethylfurfural. Catal. Commun. 123, 96–99. doi:10.1016/j.catcom.2019.02.015
Venkata Mohan, S., Nikhil, G. N., Chiranjeevi, P., Nagendranatha Reddy, C., Rohit, M. V., Kumar, A. N., et al. (2016). Waste Biorefinery Models towards Sustainable Circular Bioeconomy: Critical Review and Future Perspectives. Bioresour. Technol. 215, 2–12. doi:10.1016/j.biortech.2016.03.130
Xiang, Y., Wen, S., Tian, Y., Zhao, K., Guo, D., Cheng, F., et al. (2021). Efficient Synthesis of 5-ethoxymethylfurfural from Biomass-Derived 5-hydroxymethylfurfural over Sulfonated Organic Polymer Catalyst. RSC Adv. 11, 3585–3595. doi:10.1039/d0ra10307a
Yan, Y., Guo, H., Li, K., and Yan, L. (2022). Fabrication of Supported Acid Catalytic Composite Fibers by a Simple and Low-Cost Method and Their Application on the Synthesis of Liquid Biofuel 5-ethoxymethylfurfural. Green. Energ. Environ. 7, 165–171. doi:10.1016/j.gee.2020.06.028
Yin, Y., Ma, C. H., Li, W., and Liu, S. X. (2021). Solvent System and Conversion Mechanism of 5 over Line Hydroxymethylfurfural Preparation from Glucose. Prog. Chem. 33, 1856–1873. doi:10.7536/pc200852
Zaboon, S., Abid, H. R., Yao, Z., Gubner, R., Wang, S., and Barifcani, A. (2018). Removal of Monoethylene Glycol from Wastewater by Using Zr-Metal Organic Frameworks. J. Colloid Interf. Sci. 523, 75–85. doi:10.1016/j.jcis.2018.03.084
Zhang, J., Dong, K., Luo, W., and Guan, H. (2018). Catalytic Upgrading of Carbohydrates into 5-ethoxymethylfurfural Using SO3H Functionalized Hyper-Cross-Linked Polymer Based Carbonaceous Materials. Fuel 234, 664–673. doi:10.1016/j.fuel.2018.07.060
Zhang, L., Tian, L., Xi, G., Sun, R., and Zhao, X. (2020). Catalytic Valorization of Expired Fructan-Rich Food into the Biofuel 5-Ethoxymethylfurfural via a Restaurant Food Waste-Derived Carbonaceous Solid Acid. Waste Biomass Valor. 11, 6223–6233. doi:10.1007/s12649-019-00904-6
Zhang, Y., Li, B., Wei, Y., Yan, C., Meng, M., and Yan, Y. (2019). Direct Synthesis of Metal-Organic Frameworks Catalysts with Tunable Acid-Base Strength for Glucose Dehydration to 5-hydroxymethylfurfural. J. Taiwan Inst. Chem. Eng. 96, 93–103. doi:10.1016/j.jtice.2018.12.020
Keywords: biomass, fructose, 5-ethoxymethylfurfural, catalysis, one-step conversion
Citation: Zhao K, Xiang Y, Sun X, Chen L, Xiao J and Liu X (2022) Highly Efficient One-Step Conversion of Fructose to Biofuel 5-Ethoxymethylfurfural Using a UIO-66-SO3H Catalyst. Front. Chem. 10:900482. doi: 10.3389/fchem.2022.900482
Received: 20 March 2022; Accepted: 11 April 2022;
Published: 09 May 2022.
Edited by:
Hu Li, Guizhou University, ChinaCopyright © 2022 Zhao, Xiang, Sun, Chen, Xiao and Liu. This is an open-access article distributed under the terms of the Creative Commons Attribution License (CC BY). The use, distribution or reproduction in other forums is permitted, provided the original author(s) and the copyright owner(s) are credited and that the original publication in this journal is cited, in accordance with accepted academic practice. No use, distribution or reproduction is permitted which does not comply with these terms.
*Correspondence: Jiafu Xiao, eGlhb2ppYWZ1MTk5MEAxNjMuY29t; Xianxiang Liu, bHh4QGh1bm51LmVkdS5jbg==
Disclaimer: All claims expressed in this article are solely those of the authors and do not necessarily represent those of their affiliated organizations, or those of the publisher, the editors and the reviewers. Any product that may be evaluated in this article or claim that may be made by its manufacturer is not guaranteed or endorsed by the publisher.
Research integrity at Frontiers
Learn more about the work of our research integrity team to safeguard the quality of each article we publish.