- 1Shaanxi Province Key Laboratory of Bio-resources, QinLing-Bashan Mountains Bioresources Comprehensive Development C. I. C., Qinba State Key Laboratory of Biological Resources and Ecological Environment, College of Bioscience and Bioengineering, Shaanxi University of Technology, Hanzhong, China
- 2Department of Pharmacology, Faculty of Veterinary Medicine, Cairo University, Giza, Egypt
- 3Department of Medical Pharmacology, Faculty of Medicine, Atatürk University, Erzurum, Turkey
Graphene and its derivatives have been a burning issue in the last 10 years. Although many reviews described its application in electrochemical detection, few were focused on food detection. Herein, we reviewed the recent progress in applying graphene and composite materials in food detection during the past 10 years. We pay attention to food coloring materials, pesticides, antibiotics, heavy metal ion residues, and other common hazards. The advantages of graphene composites in electrochemical detection are described in detail. The differences between electrochemical detection involving graphene and traditional inherent food detection are analyzed and compared in depth. The results proved that electrochemical food detection based on graphene composites is more beneficial. The current defects and deficiencies in graphene composite modified electrode development are discussed, and the application prospects and direction of graphene in future food detection are forecasted.
Graphene and its Derivatives
Graphene is a single-atom 2D layer crystal atom with sp2 hybridization of carbon atoms, with a thickness of only 0.34 nm (Figure 1). The inner carbon atoms are arranged in a honeycomb lattice structure. According to different application requirements, the mechanical peeling method, SiC epitaxial growth method, Hummers’ method, and chemical vapor deposition (CVD) method are commonly used in carbon fabrication. The advantages and disadvantages of the methods mentioned previously vary. Graphene has remarkable physical and chemical properties such as high mechanical strength, excellent thermal and electrical conductivity, and a high specific surface area (theoretically, 2,630 m2/g for single-layer graphene) (Alwarappan et al., 2009; Balandin, 2011; Neto et al., 2009; Wang et al., 2009). It has significant application prospects in numerous fields, including the food industry, material manufacturing, energy, chemical industry, biological science, and medical medicine delivery.
Graphene oxide (GO), an oxygen-containing derivative of graphene, belongs to a branch of graphene research. Generally, graphene oxide is a material obtained by the multistep chemical or thermal reduction of graphene (Figure 2) (Dikin et al., 2007; Stankovich et al., 2007). Chemically synthesized GO is suitable for mass production, and the GO surface contains abundant oxygen-containing groups, which gives it greater thickness than graphene. Due to these functional groups, the GO surface is easily modified, with a fast electron transfer rate and good hydrophilic and biocompatibility properties (Dreyer et al., 2010; Liu et al., 2010; Zhou et al., 2009). The abundant oxygen-containing groups (such as -OH and -COOH) provide GO with strong hydrophilic ability. It can be dispersed in water or other organic solvents to form a stable suspension. It is also easy to modify GO through covalent or noncovalent interactions with organic small-molecule polymers (Wang et al., 2011). Recently, GO-based materials have gained increasing interest due to their excellent attributes.
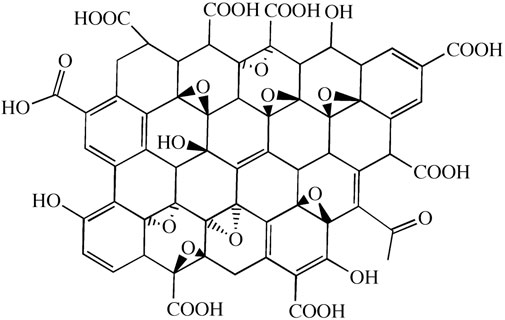
FIGURE 2. Structure of graphene oxide (Zhou et al., 2011).
Reduced graphene oxide (rGO) is an ideal support material for metal nanoparticles, which can be produced at a low cost and large scale. rGO sheets possess higher conductivity than GO sheets due to restoring the conjugated network in the rGO sheets. The oxygen-containing functional groups on rGO render it with an extremely high specific surface area, superior electronic conductivity, excellent mechanical strength, and elasticity (Sahoo et al., 2012; Kong et al., 2009). rGO is considered a promising material in the fabrication of electrochemical biosensors (Zhang L. et al., 2012; Kuila et al., 2011; Lu et al., 2012).
Graphene quantum dots (GQDs) are attractive nanomaterials consisting of a monolayer, or a few layers, of graphene with excellent and unique properties. Unlike graphene sheets, GQDs are 0D graphene segments that exhibit bandgap responsible for their unique optical and electrical properties. Due to their small size, GQDs display a quantum effect when produced using carbon-rich precursors such as fullerene, glucose, graphite, graphene oxide (GO), CNTs, and carbon fibers (CFs). Two main GQD synthesis methods are followed, namely, top–down and bottom–up methods, as shown in Figure 3 (Zhu et al., 2017). Such techniques are too complicated for the synthesis of conventional semiconductor quantum dots.
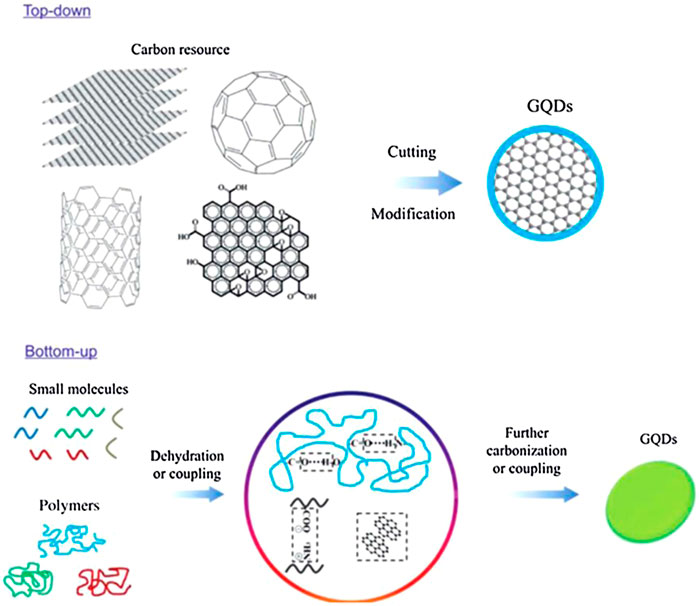
FIGURE 3. Two approaches to synthesizing GQDs: the “top-down” splitting from large molecules and “bottom–up” from small molecules (Zhu et al., 2017).
Preparation of Graphene and Its Derivative
Mechanical Peeling Method
Graphene sheets are removed directly from the surface of large graphite crystals by mechanical force and transferred to carriers such as silica. Monolayer graphene sheets can be obtained on substrates such as silica. This is the first known method to synthesize graphene using a top–down technique, by which layered graphene is split into several layered graphene sheets through repeated peeling. The obtained flakes varied greatly in size and thickness, and the product size could not be controlled. Therefore, it could not meet the industrial requirements of graphene. Entani et al. developed and modified these methods by synthesizing graphene on a SiO2 substrate. Their research showed that the resulting graphene structure was more homogenous than when using other methods.
Epitaxy Growth Method
Under high vapor pressure, high temperature (usually >1400°C), and ultra-high vacuum pressure (usually <106 Pa), silica atoms are volatilized, and the residual carbon atoms are arranged on the SiC surface to form graphene layers throughout the structure.
This method can result in large amounts of high-quality single graphene, but the SiC crystal is expensive, and the transfer of the produced graphene is challenging (Dreyer et al., 2010). Therefore, this method is mainly used to prepare graphene devices with SiC as the substrate.
Hummers’ Method
The key idea of this method is forcibly damaging π and introducing oxygen-containing functional groups made of O and C atoms, such as COOH, -OH, and C–O–C. Subsequently, graphene experiences chemical, thermal, or electrochemical reduction to remove the oxygen-containing functional groups to obtain reduced graphene oxide (reduced graphene oxide, rGO) (Zhou et al., 2009; Zhou et al., 2011). Currently, the most used chemical oxidation method is Hummers’ method. In this method, inorganic strong protic acids (such as concentrated sulfuric acid, concentrated HNO3, or a mixture) are used to treat the raw graphite. Small molecules of a strong acid are inserted in between the graphite layers, followed by strong oxidants such as KMnO4 and KCIO4 (Lee et al., 2016). Finally, hydrogen peroxide is used to remove any excess KMnO4. After filtration, diluted hydrochloric acid and ultra-pure water are used to clean the products and obtain GO (Dreyer et al., 2010). The degree of graphite oxidation depends on the selected method and reaction conditions. This method is currently the most commonly used in laboratory GO preparation, but it often fails to obtain high-quality graphene (Liu et al., 2010).
Chemical Vapor Deposition
The graphene prepared via CVD is of high quality and is expected to meet the application requirements of transparent conductive films. It is widely used in the large-scale industrial preparation of semiconductor materials for thin-film transistors.
Application of Graphene in Food Detection
As a branch of chemistry, electrochemical analysis has been verified by many practical and theoretical experiments. It is an analytical method to determine the composition and content of an object by directly measuring different electrical signal parameters, such as the current potential conductance, according to the electrochemical characteristics of different substances. Electrochemical detection methods have the advantages of low cost, small working space, fast speed, high sensitivity, and high accuracy when compared with other food detection methods. Therefore, the research, application, and promotion of electrochemical detection in food quality and related areas can play an essential role in promoting future food quality detection and standards. As an eminent factor in materials science in the 21st century, graphene has been widely used according to its excellent properties (Novoselov et al., 2012). Applying single graphene or graphene-based composite materials in the chemical modification of electrodes also shows significant development and research value. In addition, graphene and some of its derivatives, including graphene oxide (GO) and reduced graphene oxide (rGO), have been applied to detect substances in different food samples. These include colorants in food and drinks (He et al., 2018a; Qiu et al., 2016), heavy metal ions (Xia et al., 2015), antibiotic residues (Liu et al., 2017), dopamine, rutin from human samples (Yang and Li, 2014; He et al., 2018b; He et al., 2019a), pesticide residues (Liu et al., 2011), and 4-nitrophenol (He et al., 2019b) from environmental contaminants. GO and rGO showed great promise in developing chemically modified electrodes (Shao et al., 2010).
Detection of Food Coloring
The synthetic pigments that commonly appear in food are azo pigments (tartrazine, Allura Red, amaranth, carmine, and sunset Yellow). Azo dyes account for more than half of the global synthetic dye production. Azo pigments exhibit excellent color performance, and desired colors can be obtained by adjusting the type and proportion of the azo components, creating a wide variety of colors. The excessive intake of azo dyes may be harmful to human health. For example, sunset yellow and tartrazine contain groups such as azo and aromatic rings in their structure, which can induce allergies, asthma, migraines, and cancers (Somasundaram, 2014; Ye et al., 2013). Existing analytical methods for the detection of azo pigments mainly involve high-performance liquid chromatography (HPLC–MS) (Qiu et al., 2016), capillary electrophoresis (Zhao et al., 2014), surface-enhanced Raman spectroscopy (SERS) sensors (Xie et al., 2012), fluorescence (Yuan et al., 2016), and thin-layer chromatography (Soponar et al., 2008). Some of these methods are complex and do not meet economic, efficient, and convenient detection demands. Presently, electrochemical analysis methods using modified electrodes have been used to detect food additives such as citron yellow and sunset yellow as they are convenient, fast, sensitive, and highly selective (Table 1) (Qiu et al., 2016; Gan et al., 2013a; Wang and Zhao, 2015a; Wang and Zhao, 2015b).
Gan et al. used a carbon paste electrode, modified by graphene and mesoporous TiO2, to develop a rapid and low-cost method without any sample pre-treatment required to detect sunset yellow and tartrazine yellow colorants, with the detection limits of 6.0 and 8.0 nM(Gan et al., 2013b), respectively. Ding et al. (2019) studied electrochemically reduced graphene oxide (ERGO) nanoflake-decorated MnO2 nanorods (MnO2 NRs) with a modified glassy carbon electrode (GCE) surface. When compared with standard GCE, a well-defined sunset yellow (SY) oxidation peak was observed at the MnO2 NR-ERGO/GCE. It had a detection limit of 2.0 nM and a good linear response to the SY in the ranges of 0.01–2.0 µM, 2.0–10.0 µM, and 10.0–100.0 µM. This method was applied to detect SY in soft drinks and obtained satisfactory results (Ding et al., 2019). Vatandost et al. used natural tea extracts to obtain modified rGO on a carbon paste electrode surface (rGO/CPE). Since rGO/CPE has a large surface area, it has a strong enhancement effect on the electrochemical oxidation of SY, with a wide linear response range of 0.05–10 M and a detection limit of 27 nM (Elham et al., 2020). Wang et al. used a graphene nanometer material (GNM)–modified electrode, which exhibited a significantly enhanced electrochemical amaranth signal. It showed a wider linear response range from 5.0 × 10–9 to 1.0 × 10–6 M. It also possessed a low detection limit of 7.0 × 10–10 M at a signal-to-noise ratio of 3 (Wang et al., 2018). Palanisamy et al. fabricated graphene/β-CD/PtNP-modified electrodes through platinum nanoparticles (PtNPs) decorated with graphene-β-cyclodextrin (graphene/β-CD)–modified electrodes. Studies on the electrode cyclic voltammetry determination of different modified electrodes on the electrochemical behavior of Sudan I indicated that the electric catalytic activity of graphene/β-CD/PtNP-modified electrodes on Sudan I was higher than that of other modified electrodes. With a detection linear response range of 0.005–68.68 µM and a detection limit of 1.6 nM, the sensor was used for monitoring Sudan I–adulterated food samples (chili powder, chili sauce, and tomato sauce) and achieved satisfactory results in practical tests (Palanisamy et al., 2017).
Detection of Heavy Metals in Food
The excessive toxic heavy metal content is hazardous to human health, causing illnesses such as renal dysfunction, cancer, cardiovascular, and cerebrovascular diseases (Kadıoğlu et al., 2010). With industrial development, the frequent pollution accidents in the environment make food unsafe, and excessive toxic heavy metals have become one of the most severe threats to human health. Therefore, it is essential to develop a rapid, sensitive, and simple method for detecting heavy metal ions using analytical techniques, such as atomic fluorescence spectroscopy, inductively coupled plasma optical emission spectrometry (Ozbek and Akman, 2016), and induced plasma-atomic emission spectrometry (Feist and Mikula, 2014).
Graphene and its derivatives with fast electron transmission power, strong adsorption performance, and easy chemical modification are used for the electrochemical detection of heavy metals (Table 1) (Li et al., 2013). This operating instrument has attracted wide attention due to its low cost, small footprint, fast detection speed, high sensitivity, and high accuracy (Aragay and Merkoci, 2012; Zhu et al., 2014). GO possesses abundant active functional groups (such as carboxyl, hydroxyl, and epoxy), making it an excellent adsorbent for environmental applications. Despite the excellent properties of GO, its high hydrophilic nature, extensive agglomeration, and difficulty in separation from treated wastewater are considered drawbacks. In addition, the oxygen atom in the carboxyl group is categorized as a hard ligand group with less affinity for pollutants such as heavy metals (Chen et al., 2015; Zhao et al., 2016; Cui et al., 2015b). Consequently, the modification of GO through the introduction of other functional groups is the subject of ongoing research. The detection and adsorption of heavy metals were altered by modifying GO by introducing organic functional groups from multifunctional materials (Hu et al., 2015). This functionalization enhances the excellent properties of GO and increases its adsorption capacity for the removal of heavy metal ions (Gul et al., 2016).
Although zinc is one of the most important essential trace elements, a lack in the human body will lead to various diseases. However, excessive zinc intake is toxic and harmful to the body, causing various adverse reactions. Therefore, it is of great significance to determine the sensitivity of zinc in different food matrices (Feist and Mikula, 2014). Kazemi et al. proposed an innovative method for the selective extraction and determination of zinc. A novel zinc-imprinted polymer was synthesized by the co-precipitation of graphene oxide/magnetic chitosan nanocomposites. After optimizing the extraction process’s technical parameters, the adsorbent’s maximum adsorption capacity was 71.4 mg g−1, and the detection limit was 0.09 μg L−1 (Kazemi et al., 2017).
During the past decade, many researchers have combined other materials to form new graphene-based nanomaterials that can be used to detect heavy metal concentrations (Table 1). Palisoc et al. prepared graphene/gold nanoparticles (AuNPs)/hexamine mineral acid [Ru(NH3)6] 3+/Nafion via the drop coating method on a glassy carbon electrode (GCE), which successfully determined trace amounts of Pb(II), Cu(I), Cu(II), Sn(IV), and Hg(II) in canned food samples. Pb2+, Cd2+, and Cu2+ exhibited the highest sensitivity by anodic stripping voltammetry (ASV). There was an excellent linear relationship between heavy metal concentration and peak current, and the detection limit of Pb2+ was 0.74 ppb, that of Cd2+ was 37 ppb, and that of Cu2+ was 945 ppb (Palisoc et al., 2019). Wang et al. improved the extraction efficiency of Pb(II) in food samples through the synergistic effect of the BGO membrane (solid extraction) and organic solvent (liquid extraction). After optimizing the BGO membrane composition parameters, pH, elution agent types, elution time, sample volume, and other experimental conditions showed no obvious influences on different competitive ions. Under the optimal experimental conditions, the minimum detection limit was 0.84 μg L−1 and the precision was 4.65%. The method has been successfully verified based on the analysis of detecting Pb(II) added to food samples. Karthik used electron microscopy (HR-SEM), transmission electron microscopy (TEM), X-ray diffraction (XRD), and UV–Vis-NIR Fourier-transform infrared spectroscopy (FT-IR) to analyze cobalt-doped zinc oxide/reduced GO (Co: ZnO/RGO) nanorods, obtained by chemical co-precipitation (Wang J. et al., 2018). It was found that the electrode prepared with Co: ZnO/RGO nanorods had good sensitivity to Cd(II) and Pb(II) ions with excellent electrocatalytic oxidation performance, and the detection limit was 0.94 g/L (Cd(II)) and 0.83 g/L (Pb(II)), with concentrations ranging from 10 to 90 g/L (Karthik and Thambidurai, 2017).
Detection of Food Pesticides
Organophosphate pesticides (OPs) have been widely used in agricultural production due to their wide control range, low cost, and high insecticidal efficiency. Organophosphates are neurotoxins that inhibit acetylcholinesterase activity, causing acetylcholine accumulation and neurotoxicity (Gu et al., 2012). In the European Union, it is already banned from being used on crops and is only allowed under strict limitations. Taking the organophosphate insecticide phoxim as an example (Chao and Chen, 2014), it is mainly used to prevent underground pests, particularly the Lepidoptera pests affecting peanuts, vegetables, and other crops. Various methods have been developed to identify phoxim, such as high-performance liquid chromatography (HPLC) (Hamscher et al., 2007; Liang et al., 2005; Liang et al., 2006; Liu et al., 2009; Lv et al., 2009), liquid chromatography (Guo et al., 2005; Lee et al., 2010), gas chromatography (Qu et al., 1997), near-infrared spectroscopy (Gu et al., 2012; Shen et al., 2009), and spectrophotometry (Ni et al., 2007). These methods require expensive equipment, large amounts of organic solvents, or are time-consuming. Electrochemical sensors have attracted increasing attention in recent years due to their convenience, speed, high sensitivity, and selectivity (Table 2) (Huang et al., 2011; Jin et al., 2011; Keawkim et al., 2013; Wang et al., 2014). Using graphene-modified glassy carbon electrodes, Chao and Chen established an electrochemical method to directly determine phoxim traces in vegetables, meat, and egg samples. The Gr/GCE combined with linear scanning voltammetry (LSV) was successfully applied to determine phoxim in food samples such as cauliflower, lamb, and quail eggs. This method has a very sensitive nanomolar detection limit for phoxim, providing an important detection tool (Chao and Chen, 2014). Mani et al. reported an effective electrochemical sensor using NbC@Mo nanocomposite for various pesticides (i.e., fenitrothion). This study proved that NbC@Mo holds a higher electrochemical active area and improved electrocatalytic property toward FTN. A DPV-sensing platform displayed eminent electroanalytical parameters, such as a wide linear range (0.01–1889 µM) and low detection limit (0.15 nM) (Govindasamy et al., 2018). Furthermore, Mani et al. described a reproducible and reliable screen-printed carbon electrode (SPCE) modified with graphene oxide nanoribbons (GONRs) for sensitive determination of methyl parathion. The sensor exhibited two linear ranges: (1) 100 nM–100 μM, with a sensitivity of 1.804 μAμM−1 cm2, and (2) 100–2,500 μM, with a sensitivity of 0.8587 μAμM−1 cm2. The detection limit was 0.5 nM (S/N = 3). The method successfully determined methyl parathion in ugli and tomato fruits, beetroot, and broccoli, indicating its practical applicability (Govindasamy et al., 2017a).
In the early 1950s, methylcarbamate was introduced as a pesticide and is still used today (Wong et al., 2014). This is one of the most toxic carbamate insecticides used to control insects on various field crops, including potatoes, corn, carrots, and soybeans. Methylcarbamate is a systemic pesticide absorbed through plant roots and distributed to their organs, where the pesticides accumulate over time (Otieno et al., 2010). Classical analytical techniques such as gas chromatography (GC), high-pressure liquid chromatography (HPLC), and mass spectrometry (MS) are known to be sensitive and standardized techniques for the detection of carbofuran (Filho et al., 2010; Vera-Avila et al., 2012; Atrache et al., 2013). Electrochemical sensors, specifically chemically modified electrodes, have become of interest to researchers due to their portability, simplicity, minimal cost, and short analysis time (Table 2) (Wong et al., 2013; Zen et al., 2003). Wong et al. (2014) developed a technique for the sensitive and selective analysis of carbofuran pesticides based on a carbon paste electrode modified with hemin complex and graphene oxide. The selective detection of carbofuran was confirmed, and when the sensor was applied to food samples, the recovery results were close to 100%, similar to the HPLC method (Wong et al., 2014).
Methyl paraoxon (MOX) is a highly toxic organophosphate pesticide. It has been recently reported that MOX can enter the human body through ingestion, inhalation, or dermal penetration. Due to its high nondegradability, it can bind to fruits and vegetables. When consumed, it can impose sub-chronic and chronic diseases by inhibiting acetylcholinesterase in human metabolism. Umamaheswari et al. reported the detection of non-enzymatic electrochemical sensors based on the 3D porous phase graphene oxide sheet–encapsulated chalcopyrite (GOS@CuFeS2) nanocomposite. As-synthesized GOS@CuFeS2 nanocomposite film screen-printed carbon-modified electrode (SPCE) displays excellent electrocatalytic ability toward MOX. Under optimized working conditions, the modified electrode provides a linear response range from 0.073 to 801.5 µM. The detection limit was obtained as 4.5 nM. The sensor displayed outstanding sensitivity at 17.97 μA μM−1 cm−2 35. The GOS@CuFeS2 nanocomposite–modified electrode shows greater real-time practicality in actual vegetable samples (Rajaji et al., 2019a).
Detection of Food Toxins
One of the most harmful food contaminants is aflatoxin (AFT) (Conradt et al., 2015). Aflatoxin mainly exists in corn, soybean, peanut, grain, wheat, and other agricultural products and seriously affects food safety. AFB1 is classified as a group I carcinogenic compound according to the International Agency for Research on Cancer (IARC) (Liu et al., 2006). Bhardwaj et al. deposited GQDs via chemical synthesis on indium tin oxide (ITO)–coated glass substrates through electrophoretic deposition. The AFB1 monoclonal antibody was covalently fixed on the deposition electrode GQDs/ITO (Table 3). The detection limits of the standard and contaminated samples were 0.03 ng ml−1 and 0.05 ng g−1, respectively, which falls below the maximum acceptable limit stipulated by the European Union. This suggests that this method possesses a potential application value in detecting AFB1 in food (Bhardwaj et al., 2018). Srivastava et al. studied the fabrication of a highly sensitive label-free biosensor based on a graphene oxide platform to detect aflatoxin B1 (AFB1). Electrochemical impedance spectroscopy (EIS) detected the AFB1 concentration range. The impedimetric sensing response of immunoelectrodes as a function of AFB1 concentration demonstrated a wide linear detection range (0.5–5 ng/ml), high sensitivity (639 Ω ng−1 ml), improved detection limit (0.23 ng ml−1), and good stability (5 weeks) in label-free detection (Srivastava et al., 2014). Pumera described the application of graphene in biosensors used as biomolecular labels, including a bio-field effect transistor (Pumera, 2011). Reduced GO (rGO) is a promising electrochemical biosensor material due to its biocompatibility and the presence of oxygen-containing functional groups (especially carboxyl groups) (Pumera, 2010; Zhang C. et al., 2012; Kuila et al., 2011; Lu et al., 2012). Srivastava et al. (2013) synthesized chemically active rGO and deposited it onto an indium tin oxide (ITO)–coated glass substrate via electrophoretic deposition. The sensing results of the anti-AFB1/RGO/ITO––based immunoelectrode obtained as a function of aflatoxin concentration showed high sensitivity (68 mA ng−1 ml cm2) and an improved detection limit (0.12 ng ml−1) (Srivastava S Kumar et al., 2013).
Maltol, chemically known as 3-hydroxy-2-methyl-4H-pyran-4-one, is a natural flavor enhancer widely used in food (cake, beer, and drinks). Maltol is banned in children’s food by both the FDA and China’s national food safety standards because of its potential to harm health and is also banned in Europe (Ma et al., 2014). Therefore, the detection of maltol in food is crucial. Ma et al. (2014) set up a rapid method of detecting maltol, where the electrochemical behavior of maltol at the modified electrode was studied by cyclic voltammetry (Table 3). The method performed well in terms of linearity (r = 0.9981 and 0.9955), recovery (97.99.3%), reproducibility (relative standard deviation 3.1%, n = 6), and stability. Subsequently, it has been successfully applied in maltol analysis in various foods (Ma et al., 2014). Gan’s study proposed an ideal cheap voltammetric method to determine maltol in complex food matrices. SnO2@C@Go nanocomposite is a novel electrochemical reinforced material used to prepare maltol in food electrochemical detection platforms, with an excellent linear range (0.08–10 µM) and low detection limit (12 nM). This detection method extends the application range of semiconductor materials and sheds some light on the fusion of electrochemical technology and analytical methods (Gan et al., 2017).
For highly carcinogenic nitrites (Absalan et al., 2015), it is essential to develop a simple method to detect and monitor their levels in drinking water, cured food, and environmental systems (Wang et al., 2017; Schierenbeck and Smith, 2017). Zhao et al. (2017) synthesized a Pd/Fe3O4/polyDOPA/RGO composite material using a green method, and its electrocatalytic activity on nitrite oxidation was excellent (Table 3). A modified glassy carbon electrode measured the nitrite, and the amperometric response results demonstrated a wide linear range of 2.5–6470 µM and a low detection limit of 0.5 µM. Moreover, the sensor could effectively monitor the change in the nitrite content in the rapid decay of cabbage. In addition, the monitoring results showed that the nitrite content reached its peak within 1 day of corrosion and decreased to a lower level after 3 days, which was consistent with the ion chromatography trend (Zhao et al., 2017).
Acrylamide (2-propenamide) is found in carbohydrate-rich food cooked under high temperatures. Nodeh et al. (2018) successfully cleaned and measured acrylamide in various foods using a Fe3O4@G-TEOS-MTMOS RP-MSPE method and compared it with previous TEOS-MTMOS d-SPE and other published works. The Fe3O4@G-TEOS-MTMOS RPMSPE method showed low LODs (0.061–2.89 μg kg−1) and high relative recovery (82.70–105.97%) (Table 3) (Nodeh et al., 2018).
Rajaji et al. (2020) synthesized a super-active electrocatalyst of Bi2Te3@g-C3N4 BNs to quantify food toxic chemicals in meat samples. As modified, the Bi2Te3/g-C3N4 BN-modified electrode exhibits excellent electrochemical activity toward food toxic ractopamine (RAC) with high-sensitive (L.R: 0.015–456.4) and nanomolar detection limit (LOD: 1.77 nM) (Rajaji et al., 2020).
Govindasamy et al. (2019) developed a sensitive electrochemical (voltammetric; DPV) sensor for the determination of coccidiostat drug (roxarsone) based on the use of an SPCE (screen-printed carbon electrode) modified with tungsten disulfide nanosheets (WS2 NSs). Features including (a) a wider linear range (0.05–490 μM), (b) a nanomolar detection limit (0.03 μM), and (c) high sensitivity (29 μA μM−1 cm−2) have been recorded. It also yields high accuracy and good recovery (Govindasamy et al., 2019).
Detection of Food Antibiotics
Abuse of antibiotics will lead to excessive amounts in livestock, poultry, meat, and eggs. Excessive drug and antibiotic residue in livestock and poultry products threatens public health and restricts the breeding industry’s sustainable development (Hao et al., 2014; Liu et al., 2017). There are significant differences between countries and species regarding the maximum residue limits of some veterinary drugs. Developing a reliable, highly sensitive detection method for antibiotics is conducive to better food quality supervision and guaranteed human food safety and benefits the country’s export trade in agricultural products. The SP–HPLC–FLD method of E-spun GO/pancma-NF proposed by Weng et al. has been successfully applied to TC analysis in chicken tissue samples (Table 3). The adsorption of tetracycline antibiotics (TCs) in chicken samples was studied by electrospun graphene oxide–doped poly (acrylonitrile-co-maleic acid) nanofibers (E-spun-GO-PANCMA-NFs), as a new adsorbent for solid-phase extraction (SPE). The results showed good linearity in the range of 5–500 ng/ml, the correlation coefficient was higher than 0.9990, the detection limit was 20.4–44.8 μg/kg, and the quantitation limit was 69.7–115.5 μg/kg. The recovery rate of TCs added to the chicken muscle samples was 84.7–106.3%, and that of RSDs was 0.4–4.5% (Weng et al., 2019). Rajaji and Chen prepared a chloramphenicol (CAP) amperometric sensor using Eu2O3 NPS-@rGO. The sensor can be used in honey and fresh milk samples, with a high recovery rate. It possesses high sensitivity and repeatability and can detect concentrations as low as 1.32 nM (Rajaji et al., 2019b). Main and Chen prepared a chloramphenicol (CAP) amperometric sensor using MoS2/f MWCNT nanocomposite. The sensor can be used in milk, honey, and powdered milk, with a high recovery rate. Under optimized working conditions, the nanocomposite film–modified electrode responds linearly to CAP in the concentration range of 0.08–1392 μM. The detection limit was obtained as 0.015 μM (±0.003). The electrode has a high level of selectivity in the large excess concentrations of interfering species. In addition, the modified electrode offers satisfactory repeatability, reproducibility, and stability (Govindasamy et al., 2017b). Vinoth et al. developed an almond-like structured SrMoO4 embedded on sulfur-doped–graphitic carbon nitride composites (SrMoO4/SGCN) using green methods for the electrochemical detection of CAP. The sensor can be used in river water samples, urine, and human blood serum with a high recovery rate. SrMoO4/SGCN/GCE impedance shows a lower resistance charge transfer (Rct), which helps favor the superior electrochemical detection of CAP. The SrMoO4/SGCN/GCE exhibits an ultralow detection limit of 1.5 nM for an extensive concentration range of 0.005 1316.8 M and high sensitivity is 9.619 AM/cm2 using the amperometric method (Vinoth et al., 2021). Govindasamy et al.developed a novel core-shell Bi2S3@GCN electrode material–modified SPCE using green methods for a highly sensitive and selective electrocatalytic detection of antibiotics. Under the optimal conditions of electrochemical analysis, the CPL sensor exhibited responses directly proportional to concentrations (a toxic chemical) over a range of 0.02 374.4 μM, with a nanomolar detection limit of 1.2 nM (signal-to-noise ratio S/N = 3) (Govindasamy et al., 2021). Wei’s study provides an effective tool for the selective and rapid detection of SM2 in food. A three-dimensional molecularly imprinted polymer (MIP) array electrochemical sensor was used to detect sulfadiazine (SM2) residues in food. Under optimized conditions, a wide linear range of 0.2–1000 ng/ml and a detection limit of 0.169 ng/ml (S/N = 3) were obtained. The recovery rate of the sensor is 92.3–102.23%, and the relative standard deviation is 2.27–4.10% (Wei et al., 2019). He and Wang developed a simple and sensitive tool for detecting fluoroquinolone residues in animal-derived foods. An MG–DSPE–HPLC method was used to extract seven types of FQ animal–derived food. The extraction method exhibited a high adsorption capacity (6800 ng) and enrichment coefficient (6879 times) for seven fluoroquinolones. The absorbent can be reused at least 40 times, the detection limit was within the range of 0.05–0.3 ng/g, and the recovery rate of the test samples (milk, chicken muscle, and egg) was 82.4–108.5% (He et al., 2017).
Detection of Other Food Additives
Rajaji et al. developed a rapid detection of the feed additive drug (salbutamol) using bismuth telluride (Bi2Te3) decorated graphitic carbon nitride (GCN) nanostructures as a modified electrode for electrochemical sensing. A nanomolar limit of detection (1.36 nM) was calculated in a 0.05-M phosphate buffer (PB) supporting electrolyte (pH 7.0) using differential pulse voltammetry. The linear dynamic ranges concerning salbutamol concentration were 0.01 892.5 μM, and the sensor’s sensitivity was 36.277 μA μM−1cm2(Rajaji et al., 2021).
Umamaheswari et al. synthesized zinc sulfide nanospheres (ZnS NPs) encapsulated on reduced graphene oxide (RGO) hybrid by an ultrasonic bath (50 kHz/60 W). As-prepared ZnS NPs@RGO hybrid was applied toward the electrochemical determination of caffeic acid (CA) in various food samples. The sensor can be used in red wine and soft drink samples with a high recovery rate. The proposed electrochemical caffeic acid sensor produces a wide linear range of 0.015–671.7 µM with a nanomolar level detection limit (3.29 nM) (Vinoth et al., 2021).
Disadvantages
Graphene is an excellent new 2D material with an extensive application range (Yu et al., 2017; Zhang et al., 2014; Dresselhaus and Araujo, 2010). However, some disadvantages do exist. For example, the electrode material is limited by some application aspects when the graphene is separated from the dispersion liquid. Due to the influence of van der Waals forces, it tends to aggregate and accumulate during the drying process. This reduces the specific surface area. In addition, graphene hydrophobicity also limits the adsorption effect when detecting heavy metal ions in food. However, irreversible aggregation and repetition of graphene sheets limit their application on modified detection electrodes (Kim et al., 2010; Fan et al., 2010). Although multiple studies have shown that graphene has good biocompatibility, others have found that graphene possesses some biotoxicity. Graphene has a small particle size and quickly penetrates human skin, where it can interact with biological macromolecules such as proteins, lipids, and nucleic acids, generating certain biotoxicity (Singh et al., 2009; Makarucha et al., 2011). Consequently, graphene biosafety should be the focus of significant attention.
At present, the biodegradation of graphene materials mainly focuses on enzyme degradation. During body metabolic processes, the biological effect of hydrogen peroxide during substance oxidative decomposition is combined with active enzymes in the body to achieve the biodegradation of graphene materials. Differences in graphene (and its derivatives) structure, properties, and composition affect their degradation behavior. Using polyphase atom doping and surface functionalization, the physical and chemical properties of the materials are changed to affect the degradation process to regulate the enzymatic degradation of graphene materials. Some methods of obtaining graphene cannot meet research specifications due to dangerous pollution levels and low purity. Therefore, the development of green and environmentally friendly preparation methods is needed.
Prospects
Based on existing electrochemical detection models, adding modified functional graphene-like materials to develop new, more reliable, rapid, and accurate detection methods and improving detection stability and limits has always been an essential topic in food detection. Although the commercialization of graphene materials is still in its infancy, the related long-term safety and environmental issues still need to be addressed. The physical and chemical properties of graphene have a decisive influence on biological outcomes, such as the effects of size, shape, surface charge, chemical composition, and surface modification on the toxicity of biofilm, which are still unknown. Furthermore, the active mechanisms are still unclear. Research on the mechanisms and biology of graphene interaction is still in its infancy at home and abroad. The physiological and biochemical processes caused by graphene’s impact on organisms have not been thoroughly studied. Therefore, it is urgent to study further the toxic effects and functional mechanisms of graphene biofilms.
Author Contributions
JP: conceptualization, methodology, and review and editing. TR: visualization, methodology, resources, and writing—review and editing. YH: visualization, methodology, and resources. RC: investigation. WJ: investigation. SS: investigation. JW: visualization, methodology, and resources. ZL: visualization, methodology, and resources. YL: conceptualization. AMA: Validation, revising, and writing–review and editing.
Funding
This study was funded by a special support plan for high-level talents in Shaanxi Province (For JP), foreign expert project of the Ministry of science and technology (G2021041001, DL2021041001 and QN2021041001), and Research Project of Shaanxi Provincial Department of Science and Technology (2021JZY003).
Conflict of Interest
The authors declare that the research was conducted in the absence of any commercial or financial relationships that could be construed as a potential conflict of interest.
Publisher’s Note
All claims expressed in this article are solely those of the authors and do not necessarily represent those of their affiliated organizations, or those of the publisher, the editors, and the reviewers. Any product that may be evaluated in this article, or claim that may be made by its manufacturer, is not guaranteed or endorsed by the publisher.
References
Absalan, G., Akhond, M., Bananejad, A., and Ershadifar, H. (2015). Highly Sensitive Determination of Nitrite Using a Carbon Ionic Liquid Electrode Modified with Fe3O4 Magnetic Nanoparticle. J. Iran. Chem. Soc. 12, 1293–1301. doi:10.1007/s13738-015-0594-z
Alwarappan, S., Erdem, A., Liu, C., and Li, C.-Z. (2009). Probing the Electrochemical Properties of Graphene Nanosheets for Biosensing Applications. J. Phys. Chem. C 113, 8853–8857. doi:10.1021/jp9010313
Aragay, G., and Merkoçi, A. (2012). Nanomaterials Application in Electrochemical Detection of Heavy Metals. Electrochimica Acta 84, 49–61. doi:10.1016/j.electacta.2012.04.044
Bahar, S., and Karami, F. (2015). Amino-functionalized Fe3O4-Graphene Oxide Nanocomposite as Magnetic Solid-phase Extraction Adsorbent Combined with Flame Atomic Absorption Spectrometry for Copper Analysis in Food Samples. J. Iran. Chem. Soc. 12 (12), 2213–2220. doi:10.1007/s13738-015-0699-4
Balandin, A. A. (2011). Thermal Properties of Graphene and Nanostructured Carbon Materials. Nat. Mater 10, 569–581. doi:10.1038/nmat3064
Behbahani, M., Tapeh, N. A. G., Mahyari, M., Pourali, A. R., Amin, B. G., and Shaabani, A. (2014). Monitoring of Trace Amounts of Heavy Metals in Different Food and Water Samples by Flame Atomic Absorption Spectrophotometer after Preconcentration by Amine-Functionalized Graphene Nanosheet. Environ. Monit. Assess. 186 (11), 7245–7257. doi:10.1007/s10661-014-3924-1
Bhardwaj, H., Singh, C., Kotnala, R. K., and Sumana, G. (2018). Graphene Quantum Dots-Based Nano-Biointerface Platform for Food Toxin Detection. Anal. Bioanal. Chem. 410 (28), 7313–7323. doi:10.1007/s00216-018-1341-y
Chao, M., and Chen, M. (2014). Electrochemical Determination of Phoxim in Food Samples Employing a Graphene-Modified Glassy Carbon Electrode. Food Anal. Methods 7 (9), 1729–1736. doi:10.1007/s12161-014-9813-y
Chen, J. H., Xing, H. T., Sun, X., Su, Z. B., Huang, Y. H., Weng, W., et al. (2015). Highly Effective Removal of Cu(II) by Triethylenetetramine-Magnetic Reduced Graphene Oxide Composite. Appl. Surf. Sci. 356, 355–363. doi:10.1016/j.apsusc.2015.08.076
Conradt, D., Schätzle, M. A., Haas, J., Townsend, C. A., and Müller, M. (2015). New Insights into the Conversion of Versicolorin A in the Biosynthesis of Aflatoxin B1. J. Am. Chem. Soc. 137 (34), 10867–10869. doi:10.1021/jacs.5b06770
Cui, L., Wang, Y., Gao, L., Hu, L., Yan, L., Wei, Q., et al. (2015b). EDTA Functionalized Magnetic Graphene Oxide for Removal of Pb(II), Hg(II) and Cu(II) in Water Treatment: Adsorption Mechanism and Separation Property. Chem. Eng. J. 281, 1–10. doi:10.1016/j.cej.2015.06.043
Dikin, D. A., Stankovich, S., Zimney, E. J., Piner, R. D., Dommett, G. H. B., Evmenenko, G., et al. (2007). Preparation and Characterization of Graphene Oxide Paper. Nature 448, 457–460. doi:10.1038/nature06016
Ding, Z., Deng, P., Wu, Y., Tian, Y., Li, G., Liu, J., et al. (2019). A Novel Modified Electrode for Detection of the Food Colorant Sunset Yellow Based on Nanohybrid of MnO₂ Nanorods-Decorated Electrochemically Reduced Graphene Oxide. MOLECULES 24 (6). doi:10.3390/molecules24061178
Dresselhaus, M. S., and Araujo, P. T. (2010). Perspectives on the 2010 Nobel Prize in Physics for Graphene. ACS Nano 4 (11), 6297–6302.
Dreyer, D. R., Park, S., Bielawski, C. W., and Ruoff, R. S. (2010). The Chemistry of Graphene Oxide. Chem. Soc. Rev. 39, 228–240. doi:10.1039/b917103g
Elham, V., Azade, G-H., Fereshteh, C., Shahram, N. R., and Seyed-Ahmad, S. (2020). Green Tea Extract Assisted Green Synthesis of Reduced Graphene Oxide: Application for Highly Sensitive Electrochemical Detection of Sunset Yellow in Food Products. Food Chem. X, 100085.
Fan, W., He, M., Wu, X., Chen, B., and Hu, B. (2015). Graphene Oxide/polyethyleneglycol Composite Coated Stir Bar for Sorptive Extraction of Fluoroquinolones from Chicken Muscle and Liver. J. Chromatogr. A 1418, 36–44. doi:10.1016/j.chroma.2015.09.052
Fan, Z. J., Yan, J., Zhi, L. J., Zhang, Q., Wei, T., Feng, J., et al. (2010). A Three-Dimensional Carbon Nanotube/graphene Sandwich and its Application as Electrode in Supercapacitors. Adv. Mat. 22 (33), 3723–3728. doi:10.1002/adma.201001029
Feist, B., and Mikula, B. (2014). Preconcentration of Heavy Metals on Activated Carbon and Their Determination in Fruits by Inductively Coupled Plasma Optical Emission Spectrometry. Food Chem. 147, 302–306. doi:10.1016/j.foodchem.2013.10.002
Feist, B., and Sitko, R. (2019). Fast and Sensitive Determination of Heavy Metal Ions as Batophenanthroline Chelates in Food and Water Samples after Dispersive Micro-solid Phase Extraction Using Graphene Oxide as Sorbent. Microchem. J. 147 (147), 30–36. doi:10.1016/j.microc.2019.03.013
Feminus, J. J., and Deepa, P. N. (2019). Electrochemical Sensor Based on Composite of Reduced Graphene and Poly-Glutamic Acid for Selective and Sensitive Detection of Lead. J. Of Mater. Science-Materials Electron. 30 (16), 15553–15562. doi:10.1007/s10854-019-01932-7
Filho, A. M., dos Santos, F. N., and Pereira, P. A. d. P. (2010). Development, Validation and Application of a Method Based on DI-SPME and GC-MS for Determination of Pesticides of Different Chemical Groups in Surface and Groundwater Samples. Microchem. J. 96, 139–145. doi:10.1016/j.microc.2010.02.018
Gan, T., Sun, J., Meng, W., Song, L., and Zhang, Y. (2013a). Electrochemical Sensor Based on Graphene and Mesoporous TiO2 for the Simultaneous Determination of Trace Colourants in Food. Food Chem. 141, 3731–3737. doi:10.1016/j.foodchem.2013.06.084
Gan, T., Sun, J., Wu, Q., Jing, Q., and Yu, S. (2013b). Graphene Decorated with Nickel Nanoparticles as a Sensitive Substrate for Simultaneous Determination of Sunset Yellow and Tartrazine in Food Samples. Electroanalysis 25 (6), 1505–1512. doi:10.1002/elan.201300008
Gan, T., Sun, J., Yu, M., Wang, K., Lv, Z., and Liu, Y. (2017). Amplified Electrochemical Determination of Maltol in Food Based on Graphene Oxide-Wrapped Tin Oxide@carbon Nanospheres. Food Chem. 214, 82–89. doi:10.1016/j.foodchem.2016.07.054
Govindasamy, M., Wang, S. F., Jothiramalingam, R., Noora Ibrahim, S., and Al-Lohedan, H. A. (2019). A Screen-Printed Electrode Modified with Tungsten Disulfide Nanosheets for Nanomolar Detection of the Arsenic Drug Roxarsone. Mikrochim. Acta 186 (7). doi:10.1007/s00604-019-3535-1
Govindasamy, M., Chen, S.-M., Mani, V., Devasenathipathy, R., Umamaheswari, R., Joseph Santhanaraj, K., et al. (2017a). Molybdenum Disulfide Nanosheets Coated Multiwalled Carbon Nanotubes Composite for Highly Sensitive Determination of Chloramphenicol in Food Samples Milk, Honey and Powdered Milk. J. colloid interface Sci. 485, 129–136. doi:10.1016/j.jcis.2016.09.029
Govindasamy, M., Rajaji, U., Chen, S.-M., Kumaravel, S., Chen, T.-W., Al-Hemaid, F. M. A., et al. (2018). Detection of Pesticide Residues (Fenitrothion) in Fruit Samples Based on Niobium Carbide@Molybdenum Nanocomposite: An Electrocatalytic Approach. Anal. Chim. Acta 1030, 52–60. doi:10.1016/j.aca.2018.05.044
Govindasamy, M., Umamaheswari, R., Chen, S.-M., Mani, V., and Su, C. (2017b). Graphene Oxide Nanoribbons Film Modified Screen-Printed Carbon Electrode for Real-Time Detection of Methyl Parathion in Food Samples. J. Electrochem. Soc. 164 (9), B403–B408. doi:10.1149/2.0371709jes
Govindasamy, M., Wang, S.-F., Almahri, A., and Rajaji, U. (2021). Effects of Sonochemical Approach and Induced Contraction of Core-Shell Bismuth Sulfide/graphitic Carbon Nitride as an Efficient Electrode Materials for Electrocatalytic Detection of Antibiotic Drug in Foodstuffs. Ultrason. sonochemistry 72, 105445. doi:10.1016/j.ultsonch.2020.105445
Gu, C., Xiang, B., and Xu, J. (2012). Direct Detection of Phoxim in Water by Two-Dimensional Correlation Near-Infrared Spectroscopy Combined with Partial Least Squares Discriminant Analysis. Spectrochimica Acta Part A Mol. Biomol. Spectrosc. 97, 594–599. doi:10.1016/j.saa.2012.06.046
Gul, K., Sohni, S., Waqar, M., Ahmad, F., Norulaini, N. A. N., and A. K., M. O. O. (2016). Functionalization of Magnetic Chitosan with Graphene Oxide for Removal of Cationic and Anionic Dyes from Aqueous Solution. Carbohydr. Polym. 152, 520–531. doi:10.1016/j.carbpol.2016.06.045
Guo, L., Liang, P., Zhang, T., Liu, Y., and Liu, S. (2005). Use of Continuous-Flow Microextraction and Liquid Chromatography for Determination of Phoxim in Water Samples. Chroma 61, 523–526. doi:10.1365/s10337-005-0537-4
Ha, N. R., Jung, I. P., La, I. J., Jung, H. S., and Yoon, M. Y. (2017). Ultra-sensitive Detection of Kanamycin for Food Safety Using a Reduced Graphene Oxide-Based Fluorescent Aptasensor. Sci. Rep. 7 (1), 40305–40310. doi:10.1038/srep40305
Hamscher, G., Priess, B., and Nau, H. (2007). Determination of Phoxim Residues in Eggs by Using High-Performance Liquid Chromatography Diode Array Detection after Treatment of Stocked Housing Facilities for the Poultry Red Mite (Dermanyssus Gallinae). Anal. Chim. Acta 586, 330–335. doi:10.1016/j.aca.2006.09.041
Han, Q., Wang, X., Yang, Z., Zhu, W., Zhou, X., and Jiang, H. (2014). Fe3O4@ rGO Doped Molecularly Imprinted Polymer Membrane Based on Magnetic Field Directed Self-Assembly for the Determination of Amaranth. Talanta 123, 101–108. doi:10.1016/j.talanta.2014.01.060
Hao, H., Cheng, G., Iqbal, Z., Ai, X., Hussain, H. I., Huang, L., et al. (2014). Benefits and Risks of Antimicrobial Use in Food-Producing Animals. Front. Microbiol. 5, 288. doi:10.3389/fmicb.2014.00288
He, Q., Liu, J., Liu, X., Li, G., Chen, D., Deng, P., et al. (2018a). Fabrication of Amine-Modified Magnetite-Electrochemically Reduced Graphene Oxide Nanocomposite Modified Glassy Carbon Electrode for Sensitive Dopamine Determination. Nanomaterials 8 (4), 194. doi:10.3390/nano8040194
He, Q., Liu, J., Liu, X., Xia, Y., Li, G., Deng, P., et al. (2018b). Novel Electrochemical Sensors Based on Cuprous Oxide-Electrochemically Reduced Graphene Oxide Nanocomposites Modified Electrode toward Sensitive Detection of Sunset Yellow. Molecules 23 (9), 2130. doi:10.3390/molecules23092130
He, Q., Tian, Y., Wu, Y., Liu, J., Li, G., Deng, P., et al. (2019a). Facile and Ultrasensitive Determination of 4-nitrophenol Based on Acetylene Black Paste and Graphene Hybrid Electrode. Nanomaterials 9 (3), 429. doi:10.3390/nano9030429
He, Q., Wu, Y., Tian, Y., Li, G., Liu, J., Deng, P., et al. (2019b). Facile Electrochemical Sensor for Nanomolar Rutin Detection Based on Magnetite Nanoparticles and Reduced Graphene Oxide Decorated Electrode. Nanomaterials 9 (1), 115. doi:10.3390/nano9010115
He, X., Wang, G. N., Yang, K., Liu, H. Z., Wu, X. J., and Wang, J. P. (2017). Magnetic Graphene Dispersive Solid Phase Extraction Combining High Performance Liquid Chromatography for Determination of Fluoroquinolones in Foods. Food Chem. 221, 1226–1231. doi:10.1016/j.foodchem.2016.11.035
Hu, X.-j., Jiang, Liu., Liu, Y.-g., ming, G., Wang, G.-m., Wang, H., et al. (2015). Effects of Inorganic Electrolyte Anions on Enrichment of Cu(II) Ions with Aminated Fe3O4/graphene Oxide: Cu(II) Speciation Prediction and Surface Charge Measurement. Chemosphere 127, 35–41. doi:10.1016/j.chemosphere.2015.01.013
Huang, J., Zhang, X., Lin, Q., He, X., Xing, X., Huai, H., et al. (2011). Electrochemical Sensor Based on Imprinted Sol-Gel and Nanomaterials for Sensitive Determination of Bisphenol A. Food control. 22, 786–791. doi:10.1016/j.foodcont.2010.11.017
Jeyapragasam, T., and Saraswathi, R. (2014). Electrochemical Biosensing of Carbofuran Based on Acetylcholinesterase Immobilized onto Iron Oxide-Chitosan Nanocomposite. Sens. Actuators B 191, 681–687. doi:10.1016/j.snb.2013.10.054
Jian, J.-M., Liu, Y.-Y., Zhang, Y.-L., Guo, X.-S., and Cai, Q. (2013). Fast and Sensitive Detection of Pb2+ in Foods Using Disposable Screen-Printed Electrode Modified by Reduced Graphene Oxide. SENSORS 13 (10), 13063–13075. doi:10.3390/s131013063
Jin, W., Yang, G., Wu, L., Wang, Q., Shao, H., Qin, A., et al. (2011). Detecting 5-Morpholino-3-Amino-2-Oxazolidone Residue in Food with Label-free Electrochemical Impedimetric Immunosensor. Food control. 22, 1609–1616. doi:10.1016/j.foodcont.2011.03.017
Karimi, M., Aboufazeli, F., Zhad, H. R. L. Z., Sadeghi, O., and Najafi, E. (2014). Cadmium Ions Determination in Sea Food Samples Using Dipyridyl-Functionalized Graphene Nano-Sheet. Food Anal. Methods 7 (3), 669–675. doi:10.1007/s12161-013-9671-z
Karthik, R., and Thambidurai, S. (2017). Synthesis of Cobalt Doped ZnO/reduced Graphene Oxide Nanorods as Active Material for Heavy Metal Ions Sensor and Antibacterial Activity. J. Of Alloys And Compd. 715, 254–265. doi:10.1016/j.jallcom.2017.04.298
Kazemi, E., Dadfarnia, S., Haji Shabani, A. M., and Ranjbar, M. (2017). Synthesis, Characterization, and Application of a Zn (II)-imprinted Polymer Grafted on Graphene Oxide/magnetic Chitosan Nanocomposite for Selective Extraction of Zinc Ions from Different Food Samples. Food Chem. 237, 921–928. doi:10.1016/j.foodchem.2017.06.053
Keawkim, K., Chuanuwatanakul, S., Chailapakul, O., and Motomizu, S. (2013). Determination of Lead and Cadmium in Rice Samples by Sequential Injection/anodic Stripping Voltammetry Using a Bismuth Film/crown ether/Nafion Modified Screen-Printed Carbon Electrode. Food control. 31, 14–21. doi:10.1016/j.foodcont.2012.09.025
Keramat, A., and Zare-Dorabei, R. (2017). Ultrasound-assisted Dispersive Magnetic Solid Phase Extraction for Preconcentration and Determination of Trace Amount of Hg (II) Ions from Food Samples and Aqueous Solution by Magnetic Graphene Oxide (Fe3O4@GO/2-PTSC): Central Composite Design Optimization. Ultrason. SONOCHEMISTRY 38, 421–429. doi:10.1016/j.ultsonch.2017.03.039
Kim, J., Cote, L. J., Kim, F., Yuan, W., Shull, K. R., and Huang, J. (2010). Graphene Oxide Sheets at Interfaces. J. Am. Chem. Soc. 132 (23), 8180–8186. doi:10.1021/ja102777p
Kong, B.-S., Geng, J., and Jung, H.-T. (2009). Layer-by-layer Assembly of Graphene and Gold Nanoparticles by Vacuum Filtration and Spontaneous Reduction of Gold Ions. Chem. Commun. 16, 2174. doi:10.1039/b821920f
Kuila, T., Bose, S., Khanra, P., Mishra, A. K., Kim, N. H., and Lee, J. H. (2011). Biosens. Bioelectron. 26, 4637–4648. doi:10.1016/j.bios.2011.05.039
Latrous El Atrache, L., Ben Sghaier, R., Bejaoui Kefi, B., Haldys, V., Dachraoui, M., and Tortajada, J. (2013). Factorial Design Optimization of Experimental Variables in Preconcentration of Carbamates Pesticides in Water Samples Using Solid Phase Extraction and Liquid Chromatography-Electrospray-Mass Spectrometry Determination. Talanta 117, 392–398. doi:10.1016/j.talanta.2013.09.032
Lee, J. H., Park, S., Jeong, W. Y., Park, H. J., Kim, H. G., Lee, S.-J., et al. (2010). Simultaneous Determination of Phoxim and its Photo-Transformation Metabolite Residues in Eggs Using Liquid Chromatography Coupled with Electrospray Ionization Tandem Mass Spectrometry. Anal. Chim. Acta 674, 64–70. doi:10.1016/j.aca.2010.06.016
Lee, P. M., Ng, H. W., Lim, J. D., Khun, N. W., Chen, Z., and Liu, E. (2016). Nanostructure Restoration of Thermally Reduced Graphene Oxide Electrode upon Incorporation of Nafion for Detection of Trace Heavy Metals in Aqueous Solution. ELECTROANALYSIS 28, 9 2037–2043. doi:10.1002/elan.201501099
Liang, P., Guo, L., Liu, Y., Liu, S., and Zhang, T.-z. (2005). Application of Liquid-phase Microextraction for the Determination of Phoxim in Water Samples by High Performance Liquid Chromatography with Diode Array Detector. Microchem. J. 80, 19–23. doi:10.1016/j.microc.2004.07.004
Liang, P., Xu, J., Guo, L., and Song, F. (2006). Dynamic Liquid-phase Microextraction with HPLC for the Determination of Phoxim in Water Samples. J. Sep. Sci. 29, 366–370. doi:10.1002/jssc.200500385
Liu, T., Su, H., Qu, X., Ju, P., Cui, L., and Ai, S. (2011). Acetylcholinesterase Biosensor Based on 3-carboxyphenylboronic Acid/reduced Graphene Oxide–Gold Nanocomposites Modified Electrode for Amperometric Detection of Organophosphorus and Carbamate Pesticides. Sens. Actuators B Chem. 160 (1), 1255–1261. doi:10.1016/j.snb.2011.09.059
Liu, X., Steele, J. C., and Meng, X. Z. (2017). Usage, Residue, and Human Health Risk of Antibiotics in Chinese Aquaculture: a Review. Environ. Pollut. 223, 161–169. doi:10.1016/j.envpol.2017.01.003
Liu, Y., Qin, Z., Wu, X., and Jiang, H. (2006). Immune-biosensor for Aflatoxin B1 Based Bio-Electrocatalytic Reaction on Micro-comb Electrode. Biochem. Eng. J. 32, 211–217. doi:10.1016/j.bej.2006.10.003
Liu, Y., Yu, D., Zeng, C., Miao, Z., and Dai, L. (2010). Biocompatible Graphene Oxide-Based Glucose Biosensors. Langmuir 26, 6158–6160. doi:10.1021/la100886x
Lu, Z., Zhang, L., Li, Y. S., and He, N. (2012). Graphene Oxide for Rapid microRNA Detection. Nanoscale 4, 5840–5842. doi:10.1039/c2nr31497e
Luo, X., Pan, J., Pan, K., Yu, Y., Zhong, A., Wei, S., et al. (2015). An Electrochemical Sensor for Hydrazine and Nitrite Based on Graphene–Cobalt Hexacyanoferrate Nanocomposite: Toward Environment and Food Detection. J. Electroanal. Chem. 745, 80–87. doi:10.1016/j.jelechem.2015.03.017
Lv, Z., Gao, L., Gao, H., Hou, Z., and Zhang, B. (2009). J. Food Sci. 74, T37–T41. doi:10.1111/j.1750-3841.2009.01157.x
Ma, J., Zhang, B., Wang, Y. N., Hou, X. F., and He, L. C. (2014). Determination of Flavor Enhancers in Milk Powder by One-step Sample Preparation and Two-Dimensional Liquid Chromatography. J. Sep. Sci. 37, 920–926. doi:10.1002/jssc.201301367
Ma, X., Wang, J., Sun, M., Wang, W., Wu, Q., Wang, C., et al. (2013b). Magnetic Solid-phase Extraction of Neonicotinoid Pesticides from Pear and Tomato Samples Using Graphene Grafted Silica-Coated Fe3O4 as the Magnetic Adsorbent. Anal. Methods 5, 2809–2815. doi:10.1039/c3ay40207j
Ma, X. Y., Chao, M. Y., and Wang, Z. X. (2013a). Electrochemical Determination of Sudan I in Food Samples at Graphene Modified Glassy Carbon Electrode Based on the Enhancement Effect of Sodium Dodecyl Sulphonate. Food Chem. 138, 739–744. doi:10.1016/j.foodchem.2012.11.004
Ma, Y. D., Yu, C., Yu, Y. J., Chen, J., Gao, R. F., and He, J. L. (2019). DNAzyme Assisted Recycling Amplification Method for Ultrasensitive Amperometric Determination of Lead(II) Based on the Use of a Hairpin Assembly on a Composite Prepared from Nitrogen-Doped Graphene, Perylenetetracarboxylic Anhydride, Thionine and Gold Nanoparticles. Microchim. ACTA 186 (10), 677. doi:10.1007/s00604-019-3790-1
Majidi, M. R., Pournaghi-Azar, M. H., Baj, R. F. B., and Naseri, A. (2015). Formation of Graphene Nanoplatelet-like Structures on Carbon-Ceramic Electrode Surface: Application for Simultaneous Determination of Sunset Yellow and Tartrazine in Some Food Samples. Ionics 21 (3), 863–875. doi:10.1007/s11581-014-1223-z
Makarucha, A. J., Todorova, N., and Yarovsky, I. (2011). Nanomaterials in Biological Environment: a Review of Computer Modelling Studies[J]. Eur. Biophysics J. 40 (2), 103–115. doi:10.1007/s00249-010-0651-6
Neto, A. C., Guinea, F., Peres, N., Novoselov, K. S., and Geim, A. K. (2009). The Electronic Properties of Graphene. Rev. Mod. Phys. 81, 109. doi:10.1103/revmodphys.81.109
Nodeh, H. R., Ibrahim, W. A. W., Kamboh, M. A., and Sanagi, M. M. (2018). Magnetic Graphene Sol-Gel Hybrid as Clean-Up Adsorbent for Acrylamide Analysis in Food Samples Prior to GC–MS. Food Chem. 239, 208–216. doi:10.1016/j.foodchem.2017.06.094
Novoselov, K. S., Fal′ko, V. I., Colombo, L., Gellert, P. R., Schwab, M. G., and Kim, K. (2012). A Roadmap for Graphene. Nature 490 (7419), 192–200. doi:10.1038/nature11458
Otieno, P. O., Lalah, J. O., Virani, M., Jondiko, I. O., and Schramm, K.-W. (2010). Carbofuran and its Toxic Metabolites Provide Forensic Evidence for Furadan Exposure in Vultures (Gyps Africanus) in Kenya. Bull. Environ. Contam. Toxicol. 84, 536–544. doi:10.1007/s00128-010-9956-5
Ozbek, N., and Akman, S. (2016). Method Development for the Determination of Calcium, Copper, Magnesium, Manganese, Iron, Potassium, Phosphorus and Zinc in Different Types of Breads by Microwave-Induced Plasma-Atomic Emission Spectrometry. Food Chem. 200, 245–248. doi:10.1016/j.foodchem.2016.01.043
Palanisamy, S., Kokulnathan, T., Chen, S. M., Velusamy, V., and Ramaraj, S. K. (2017). Voltammetric Determination of Sudan I in Food Samples Based on Platinum Nanoparticles Decorated on Graphene-β-Cyclodextrin Modified Electrode. J. Electroanal. Chem. 794, 64–70. doi:10.1016/j.jelechem.2017.03.041
Palisoc, S. T., Bentulan1, J. M. O., and Natividad, M. T. (2019). Determination of Trace Heavy Metals in Canned Food Using Graphene/AuNPs/[Ru(NH3)6] 3+/Nafion Modified Glassy Carbon Electrodes. J. Of Food Meas. And Charact. 13 (1), 169–176. doi:10.1007/s11694-018-9930-1
Pizarro, J., egura, R., Tapia, D., Bollo, S., and Sierra-Rosales, P. (2019). Electroanalytical Determination of Cd(II) and Pb(II) in Bivalve Mollusks Using Electrochemically Reduced Graphene Oxide-Based Electrode. ELECTROANALYSIS 31 (11), 2199–2205. doi:10.1002/elan.201900061
Pourjavid, M. R., Sehat, A. A., Arabieh, M., Yousefifi, S. R., Hosseini, M. H., and Rezaee, M. (2014). Column Solid-phase Extraction and Flflame Atomic Absorption Spectrometric Determination of Manganese(II) and Iron(III) Ions in Water, Food and Biological Samples Using 3-(1-Methyl-1h-Pyrrol-2-Yl)-1h-Pyrazole-5-Carboxylic Acid on Synthesized Graphene Oxide Materials. Sci. Eng. C 35, 370–378. doi:10.1016/j.msec.2013.11.029
Qiu, X., Lu, L., Leng, J., Yu, Y., Wang, W., Jiang, M., et al. (2016). An Enhanced Electrochemical Platform Based on Graphene Oxide and Multi-Walled Carbon Nanotubes Nanocomposite for Sensitive Determination of Sunset Yellow and Tartrazine. Food Chem. 190, 889–895. doi:10.1016/j.foodchem.2015.06.045
Qu, G., Yang, J., Li, J., and Xie, L. (1997). Chin. J. Chromatogr. 15, 252–253. doi:10.1023/a:1007901206431
Rajaji, U., Chen, T,-W., Chinnapaiyan, S., Chen, S-M., and Govindasamy, M. (2020). Two-dimensional Binary Nanosheets (Bi2Te3@ G-C3n4): Application toward the Electrochemical Detection of Food Toxic Chemical. Anal. Chim. Acta 1125, 220–230. doi:10.1016/j.aca.2020.05.033
Rajaji, U., Manavalan, S., Chen, S. M., Govindasamy, M., Chen, T. W., and Maiyalagan, T. (2019a). Microwave-assisted Synthesis of Europium(III) Oxide Decorated Reduced Graphene Oxide Nanocomposite for Detection of Chloramphenicol in Food Samples. Compos. PART B-ENGINEERING 161, 29–36. doi:10.1016/j.compositesb.2018.10.043
Rajaji, U., Murugan, K., Chen, S-M., Govindasamy, M., Chen, T-W., Lin, P. H., et al. (2019b). Graphene Oxide Encapsulated 3D Porous Chalcopyrite (CuFeS2) Nanocomposite as an Emerging Electrocatalyst for Agro-Hazardous (Methyl Paraoxon) Detection in Vegetables. Compos. Part B Eng. 160, 268–276. doi:10.1016/j.compositesb.2018.10.042
Sa’adi, A., and Es’haghi, Z. (2019). Azo-phenol Ligand Surface-Active Magnetic Graphene Oxide Nanosheets as Solid-phase Adsorbents for Extraction of Cadmium in Food Samples. J. Of Food Meas. And Charact. 13 (1), 579–591. doi:10.1007/s11694-018-9971-5
Sahoo, N. G., Pan, Y. Z., Li, L., and Chan, S. H. (2012). Graphene-based Materials for Energy Conversion. Adv. Mater 24, 4203. doi:10.1002/adma.201104971
Samphao, A., Suebsanoh, P., Wongsa1, Y., Pekec, B., Jitchareon, J., and Kalcher, K. (2013). Alkaline Phosphatase Inhibition-Based Amperometric Biosensor for the Detection of Carbofuran. Int. J. Electrochem. Sci. 8, 3254–3264.
Schierenbeck, T. M., and Smith, M. C. (2017). Path to Impact for Autonomous Field-Deployable Chemical Sensors: A Case Study of In Situ Nitrite Sensors. Environ. Sci. Tech. 51, 4755–4771. doi:10.1021/acs.est.6b06171
Shahrebabak, S. M., Saber-Tehrani, M., Faraji, M., Shabanian, M., and Aberoomand-Azar, P. (2019). Simultaneous Magnetic Solid-phase Extraction of Acidic and Basic Pesticides Using Triazine-Based Polymeric Network Modified Magnetic Nanoparticles/Graphene Oxide Nanocomposite in Water and Food Samples. Microchem. J. 146, 630–639. doi:10.1016/j.microc.2019.01.047
Shao, Y., Wang, J., Wu, H., Liu, J., Aksay, I. A., and Lin, Y. (2010). Graphene-based Electrochemical Sensors and Biosensors: a Review. Electroanal. Int. J. Devoted Fundam. Pract. Asp. Electroanal. 22 (10), 1027–1036. doi:10.1002/elan.200900571
Singh, N., Manshian, B., and Gareth, J. S. (2009). Nano Genotoxicology: the DNA Damaging Potential of Engineered Nanomaterials [J]. Biomaterials 30 (23), 3891–3914. doi:10.1016/j.biomaterials.2009.04.009
Somasundaram, S. T. (2014). Biodegradation of Carcinogenic Textile Azo Dyes Using Bacterial Isolates of Mangrove Sediment. J. Coast. Life Med. 2 (2), 154–162. doi:10.12980/jclm.2.201414j2
Soponar, F., Moţ, A. C., and Sârbu, C. (2008). Quantitative Determination of Some Food Dyes Using Digital Processing of Images Obtained by Thin-Layer Chromatography. J. Chromatogr. A 1188 (2), 295–300. doi:10.1016/j.chroma.2008.02.077
Srivastava, S., Ali, M. A., Umrao, S., Parashar, U. K., Srivastava, A., Sumana, G., et al. (2014). Graphene Oxide-Based Biosensor for Food Toxin. Detection 174 (3), 960–970. doi:10.1007/s12010-014-0965-4
Srivastava S Kumar, V., Ali, M. A., Solanki, P. R., Srivastava, A., Sumana, G., Saxena, P. S., et al. (2013). Electrophoretically Deposited Reduced Graphene Oxide Platform for Food Toxin Detection. NANOSCALE 5 (7), 3043–3051. doi:10.1039/c3nr32242d
Stankovich, S., Dikin, D. A., Piner, R. D., Kohlhaas, K. A., Kleinhammes, A., Jia, Y., et al. (2007). Synthesis of Graphene-Based Nanosheets via Chemical Reduction of Exfoliated Graphite Oxide. Carbon 45, 1558–1565. doi:10.1016/j.carbon.2007.02.034
Vera-Avila, L. E., Márquez-Lira, B. P., Villanueva, M., Covarrubias, R., Zelada, G., and Thibert, V. (2012). Determination of Carbofuran in Surface Water and Biological Tissue by Sol-Gel Immunoaffinity Extraction and On-Line preconcentration/HPLC/UV Analysis. Talanta 88, 553–560. doi:10.1016/j.talanta.2011.11.032
Vinoth, S., Govindasamy, M., Wang, S-F., ALOthman, Z. A., Alshgari, R. A., and Ouladsmane, M. (2021). Fabrication of Strontium Molybdate Incorporated with Graphitic Carbon Nitride Composite: High-Sensitive Amperometric Sensing Platform of Food Additive in Foodstuffs. Microchem. J. 167 (2021), 106307.
Wang, C., Li, D., Too, C. O., and Wallace, G. G. (2009). Electrochemical Properties of Graphene Paper Electrodes Used in Lithium Batteries. Chem. Mater. 21, 2604–2606. doi:10.1021/cm900764n
Wang, J., Liu, M. S., Zhang, L., Zhang, T. S., Yue, T. L., Li, Z. H., et al. (2018a). Biomass Reinforced Graphene Oxide Solid/liquid Phase Membrane Extraction for the Measurement of Pb(II) in Food Samples. FOOD Chem. 269, 9–15. doi:10.1016/j.foodchem.2018.06.137
Wang, J., Yang, B., Wang, H., Yang, P., and Du, Y. (2015). Highly Sensitive Electrochemical Determination of Sunset Yellow Based on Gold Nanoparticles/graphene Electrode. Anal. Chim. Acta 893, 41–48. doi:10.1016/j.aca.2015.08.042
Wang, L., Qi, W., Su, R., and He, Z. (2014). Sensitive and Efficient Electrochemical Determination of Kojic Acid in Foodstuffs Based on Graphene-Pt Nanocomposite-Modified Electrode. Food Anal. Methods 7, 109–115. doi:10.1007/s12161-013-9604-x
Wang, M. L., Cui, M. Z., Zhao, M., and Cao, H. L. (2018b). Sensitive Determination of Amaranth in Foods Using Graphene Nanomeshes. J. Electroanal. Chem. 809, 117–124. doi:10.1016/j.jelechem.2017.12.059
Wang, M., and Zhao, J. (2015a). A Facile Method Used for Simultaneous Determination of Ponceau 4R, Allura Red and Tartrazine in Alcoholic Beverages. J. Electrochem. Soc. 162 (6), H321–H327. doi:10.1149/2.0111506jes
Wang, M., and Zhao, J. (2015b). Facile Synthesis of Au Supported on Ionic Liquid Functionalized Reduced Graphene Oxide for Simultaneous Determination of Sunset Yellow and Tartrazine in Drinks. Sens. Actuators B Chem. 216, 578–585. doi:10.1016/j.snb.2015.04.053
Wang, Q., Yu, L., Liu, Y., Lan, L., Lu, R., Zhu, J., et al. (2017). Methods for the Detection and Determination of Nitrite and Nitrate: A Review. Talanta 165, 709–720. doi:10.1016/j.talanta.2016.12.044
Wang, Y. K., Ou, Y. H., Xie, S. Y., Chen, D. M., Wang, X., Pan, Y. H., et al. (2019). Magnetic Graphene Solid-phase Extraction for the Determination of 47 Kinds of Non-steroidal Anti-inflammatory Drug Residues in Animal Food with Liquid Chromatography-Tandem Mass Spectrometry. FOOD Anal. METHODS (12), 1346–1368. doi:10.1007/s12161-019-01440-8
Wang, Y., Li, Z., Wang, J., Li, J., and Lin, Y. (2011). Graphene and Graphene Oxide: Biofunctionalization and Applications in Biotechnology. Trends Biotechnol. 29, 205–212. doi:10.1016/j.tibtech.2011.01.008
Wei, X. B., Zhang, Z. R., Zhang, L. F., and Xu, X. H. (2019). Synthesis of Molecularly Imprinted polymers/NiCo2O4 Nanoneedle Arrays on 3D Graphene Electrode for Determination of Sulfadimidine Residue in Food. J. Mater. Sci. 54 (3), 2066–2078. doi:10.1007/s10853-018-2975-z
Weng, R., Sun, L. S., Jiang, L. P., Li, N., Ruan, G. H., Li, J. P., et al. (2019). Electrospun Graphene Oxide–Doped Nanofiber-Based Solid Phase Extraction Followed by High-Performance Liquid Chromatography for the Determination of Tetracycline Antibiotic Residues in Food Samples. FOOD Anal. METHODS (12), 1594–1603. doi:10.1007/s12161-019-01495-7
Wong, A., Materon, E. M., and Sotomayor, M. D. T. (2014). Development of A Biomimetic Sensor Modified with Hemin and Graphene Oxide for Monitoring of Carbofuran in. FOOD Electrochimica Acta 146, 830–837. doi:10.1016/j.electacta.2014.09.091
Wong, A., de Vasconcelos Lanza, M. R., and Sotomayor, M. D. P. T. (2013). Sensor for Diuron Quantitation Based on the P450 Biomimetic Catalyst Nickel(II) 1,4,8,11,15,18,22,25-Octabutoxy-29h,31h-Phthalocyanine. J. Electroanal. Chem. 690, 83–88. doi:10.1016/j.jelechem.2012.11.007
Xia, B., Chu, M., Wang, S., Wang, W., Yang, S., Liu, C., et al. (2015). Graphene Oxide Amplified Electrochemiluminescence of Graphitic Carbon Nitride and its Application in Ultrasensitive Sensing for Cu2+. Anal. Chim. Acta 891, 113–119. doi:10.1016/j.aca.2015.05.054
Xie, Y., Li, Y., Niu, L., Wang, H., Qian, H., and Yao, W. (2012). A Novel Surface-Enhanced Raman Scattering Sensor to Detect Prohibited Colorants in Food by Graphene/silver Nanocomposite. Talanta 100, 32–37. doi:10.1016/j.talanta.2012.07.080
Yang, Y. J., and Li, W. (2014). CTAB Functionalized Graphene Oxide/multiwalled Carbon Nanotube Composite Modified Electrode for the Simultaneous Determination of Ascorbic Acid, Dopamine, Uric Acid and Nitrite. Biosens. Bioelectron. 56, 300–306. doi:10.1016/j.bios.2014.01.037
Yao, Y. Z., Liu, Y. C., and Yang, Z. S. (2016). Highly Sensitive Electrochemical Sensor for the Food Toxicant Sudan I Based on a Glassy Carbon Electrode Modified with Reduced Graphene Oxide Decorated with Ag-Cu Nanoparticles. Microchim. Acta 183 (12), 3275–3283. doi:10.1007/s00604-016-1977-2
Yavuz, S., Erkal, A., Kariper, I. A., Solak, A. O., Jeon, S., Mülazımoğlu, I. E., et al. (2016). Carbonaceous Materials-12: a Novel Highly Sensitive Graphene Oxide-Based Carbon Electrode: Preparation, Characterization, and Heavy Metal Analysis in Food Samples. Food Anal. Methods 9 (2), 322–331. doi:10.1007/s12161-015-0198-3
Ye, X., Du, Y., Lu, D., and Wang, C. (2013). Fabrication of β-cyclodextrin-coated Poly (Diallyldimethylammonium Chloride)-Functionalized Graphene Composite Film Modified Glassy Carbon-Rotating Disk Electrode and its Application for Simultaneous Electrochemical Determination Colorants of Sunset Yellow and Tartrazine. Anal. Chim. Acta 779, 22–34. doi:10.1016/j.aca.2013.03.061
Yi, W., He, Z., Fei, J., and He, X. (2019). Sensitive Electrochemical Sensor Based on Poly (L-Glutamic Acid)/graphene Oxide Composite Material for Simultaneous Detection of Heavy Metal Ions[J]. RSC Adv. 9 (30), 17325–17334. doi:10.1039/c9ra01891c
Yu, L., Shi, M., Yue, X., and Qu, L. (2016). Detection of Allura Red Based on the Composite of Poly (Diallyldimethylammonium Chloride) Functionalized Graphene and Nickel Nanoparticles Modified Electrode. Sensors Actuators B Chem. 225, 398–404. doi:10.1016/j.snb.2015.11.061
Yu, X., Chen, H. h., Zhang, M., Zhao, Y., and Qu, L. (2017). Graphene-based Smart Materials[J]. Nat. Rev. Mater. 2 (9), 17–046. doi:10.1038/natrevmats.2017.46
Yuan, Y., Zhao, X., Qiao, M., Zhu, J., Liu, S., Yang, J., et al. (2016). Determination of Sunset Yellow in Soft Drinks Based on Fluorescence Quenching of Carbon Dots. Spectrochim. Acta Part A Mol. Biomol. Spectrosc. 167, 106–110. doi:10.1016/j.saa.2016.05.038
Zekavati, R., Safi, S., Hashemi, S. J., Rahmani-Cherati, T., Tabatabaei, M., Mohsenifar, A., et al. (2013). Highly Sensitive FRET-Based Fluorescence Immunoassay for Aflatoxin B1 Using Cadmium Telluride Quantum Dots. Microchim. Acta 180, 1217–1223. doi:10.1007/s00604-013-1047-y
Zen, J.-M., Senthil Kumar, A., and Tsai, D.-M. (2003). Recent Updates of Chemically Modified Electrodes in Analytical Chemistry. Electroanalysis 15, 1073–1087. doi:10.1002/elan.200390130
Zhang, C., Zhang, J., Wang, K., and Dai, Z. (2012b). Acta Chim. Sin. 70, 1008–1012. doi:10.6023/a1108021
Zhang, J., Long, S., Zhang, Z., Chen, N., and Qu, L. Environmentally Responsive Graphene Systems[J], Small, 2014, 10 (11):2 151–152 164.doi:10.1002/smll.201303080
Zhang, L., Zhang, A., Du, D., and Lin, Y. (2012a). Biosensor Based on Prussian Blue Nanocubes/reduced Graphene Oxide Nanocomposite for Detection of Organophosphorus Pesticides. Nanoscale 4, 4674–4679. doi:10.1039/c2nr30976a
Zhao, D., Gao, X., Wu, C., Xie, R., Feng, S., and Chen, C. (2016). Facile Preparation of Amino-Functionalized Graphene Oxide Decorated with Fe3O4 Nanoparticles for the Adsorption of Cr(VI). Appl. Surf. Sci. 384, 1–9. doi:10.1016/j.apsusc.2016.05.022
Zhao, L., Zeng, B., and Zhao, F. (2014). Electrochemical Determination of Tartrazine Using a Molecularly Imprinted Polymer - Multiwalled Carbon Nanotubes - Ionic Liquid Supported Pt Nanoparticles Composite Film Coated Electrode. Electrochimica Acta 146, 611–617. doi:10.1016/j.electacta.2014.08.108
Zhao, Z. Y., Xia, Z. H., Liu, C. Y., Huang, H., and Ye, W. C. (2017). Green Synthesis of Pd/Fe3O4 Composite Based on polyDOPA Functionalized Reduced Graphene Oxide for Electrochemical Detection of Nitrite in Cured Food. ELECTROCHIMICA ACTA 256, 146–154. doi:10.1016/j.electacta.2017.09.185
Zhou, M., Zhai, Y., and Dong, S. (2009). Electrochemical Sensing and Biosensing Platform Based on Chemically Reduced Graphene Oxide. Anal. Chem. 81, 5603–5613. doi:10.1021/ac900136z
Zhou, T. N., Chen, F., Liu, K., Deng, H., Zhang, Q., Feng, J., et al. (2011). A Simple and Efficient Method to Prepare Graphene by Reduction of Graphite Oxide with Sodium Hydrosulfite [J]. Nanotechnology 22 (4), 45704. doi:10.1088/0957-4484/22/4/045704
Zhu, C., Yang, G., Li, H., Du, D., and Lin, Y. (2014). Electrochemical Sensors and Biosensors Based on Nanomaterials and Nanostructures. Anal. Chem. 87, 230–249. doi:10.1021/ac5039863
Keywords: electrochemical detection, graphene derivatives, pigments, pesticides, toxins
Citation: Pei J, Ren T, Huang Y, Chen R, Jin W, Shang S, Wang J, Liu Z, Liang Y and Abd El-Aty AM (2022) Application of Graphene and its Derivatives in Detecting Hazardous Substances in Food: A Comprehensive Review. Front. Chem. 10:894759. doi: 10.3389/fchem.2022.894759
Received: 12 March 2022; Accepted: 04 May 2022;
Published: 01 July 2022.
Edited by:
Cheng Zhong, Tianjin University, ChinaReviewed by:
Mani Govindasamy, National Taipei University of Technology, TaiwanCeren Karaman, Akdeniz University, Turkey
Copyright © 2022 Pei, Ren, Huang, Chen, Jin, Shang, Wang, Liu, Liang and Abd El-Aty. This is an open-access article distributed under the terms of the Creative Commons Attribution License (CC BY). The use, distribution or reproduction in other forums is permitted, provided the original author(s) and the copyright owner(s) are credited and that the original publication in this journal is cited, in accordance with accepted academic practice. No use, distribution or reproduction is permitted which does not comply with these terms.
*Correspondence: Jinjin Pei, amluamlucGVpc2xnQDE2My5jb20=; Yinku Liang, MzA4OTI3MDg0QHFxLmNvbQ==; A. M. Abd El-Aty, YWJkZWxhdHk0NEBob3RtYWlsLmNvbQ==
†These authors share first authorship