- 1School of Civil Engineering, Xi’an University of Architecture and Technology, Xi’an, China
- 2Shaanxi Key Laboratory of Geotechnical and Underground Space Engineering (XAUAT), Xi’an, China
Heavy metal contamination during the rapid urbanization process in recent decades has notably impacted our fragile environments and threatens human health. However, traditional remediation approaches are considered time-consuming and costly, and the effect sometimes does not meet the requirements expected. The present study conducted test tube experiments to reproduce enzyme-induced carbonate precipitation applied to lead remediation under the effects of urease concentration and a calcium source. Furthermore, the speciation and sequence of the carbonate precipitation were simulated using the Visual MINTEQ software package. The results indicated that higher urease concentrations can assure the availability of CO32− during the enzyme-induced carbonate precipitation (EICP) process toward benefiting carbonate precipitation. The calcium source determines the speciation of carbonate precipitation and subsequently the Pb remediation efficiency. The use of CaO results in the dissolution of Pb(OH)2 and, therefore, discharges Pb ions, causing some difficulty in forming the multi-layer structure of carbonate precipitation and degrading Pb remediation. The findings of this study are useful in widening the horizon of applications of the enzyme-induced carbonate precipitation technology to heavy metal remediation.
Introduction
Heavy metal contamination in the soil and underground water seriously threatens surrounding fragile environments and human health (Kim et al., 2001; Mohan et al., 2006; Bai et al., 2017; Chang et al., 2019; Chen et al., 2022a; Hu et al., 2020; Bai et al., 2021; Hu et al., 2021; Liu et al., 2021; Xue et al., 2021; Hu et al., 2022; Wang et al., 2022). Such heavy metal contamination remediation by the traditional soil flushing measure is deemed time-consuming and costly since heavy metal ions are easily adsorbed by soil particles, and intensive industrial activities further aggravate heavy metal contamination (Yang et al., 2014; An et al., 2019; Kumar and Dwivedi, 2019; Bai et al., 2021; Cheng et al., 2021; Duan et al., 2021; Wei et al., 2021; Yuan et al., 2021; Chen et al., 2022b). In the past few years, various remediation measures, including but not limited to physical, chemical, and biological measures, have been proposed to deal with heavy-metal contamination (Dhami et al., 2013; Shashank et al., 2016; Qian et al., 2017; Rahman et al., 2020; Ahenkorah et al., 2021a; Hu et al., 2021; Jiang et al., 2021; and Xue et al., 2022). Amongst the heavy metal contamination remediation measures, enzyme-induced carbonate precipitation (EICP) is an environmentally friendly, efficient, and sustainable measure. Recently, the EICP technology has been widely applied to calcareous sand reinforcement (Ahenkorah et al., 2021b; Chen et al., 2021; Cui et al., 2021; and Meng et al., 2021), while studies on the remediation of contaminations, including organic and inorganic contaminants using the EICP technology, are remarkably limited (Neupane et al., 2013; Putra et al., 2016; and Moghal et al., 2020a). During the EICP process, urease catalyzes urea hydrolysis toward producing CO32−. The obtained CO32− precipitates with a calcium source, resulting in carbonate precipitation (Fisher et al., 2017; and Sun et al., 2021). Eqs 1–4 show the biochemical reactions involving urea hydrolysis driven by urease in the EICP process (Mobley and Hausinger, 1989; Fujita et al., 2010; Achal et al., 2012; Achal et al., 2013; Mitchelf et al., 2013; Mugwar and Harbottle, 2016).
A significant body of research conducted over the last few years has greatly enhanced our understanding of improving the mechanical properties of soil and mitigating dust emissions using the EICP technology. Yuan et al. (2020) studied an improved EICP method by adding organic materials (i.e., skim milk powder and brown sugar) into the urease solution and demonstrated its improvement in the mechanical properties of fine-grained soils. Almajed et al. (2018) conducted a baseline study to evaluate the effect of urease solution components on the precipitated efficiency, and a threshold of the carbonate fraction was further identified through a series of unconfined compression tests. Hamdan and Kavazanjian, (2016) performed wind tunnel tests, and the mitigation of fugitive dust emissions was attained by carbonate precipitation using the EICP technology. Hoang et al., (2019) examined the shearing resistance in sand and silt-sand mixtures using the carbonate precipitation catalyzed by the urease enzyme extracted from plants. These results demonstrate an exciting potential for the use of the EICP technology to achieve soil reinforcement and mitigation of dust emissions.
Recently, it is also attempted as a remediation measure for immobilizing organic and inorganic contaminants. Moghal et al., 2020b evaluated the efficiency of EICP in restricting the migration of heavy metals by using ethylenediaminetetraacetic acid (EDTA) and citric acid as extractants. The results indicated that the retention efficiency of the remediated soil can be improved by 30% in comparison with the unremediated soil. Nam et al. (2016) examined the ability of the Canavalia ensiformis extract to catalyze urea to form carbonate precipitation on heavy metals resulting from abandoned mines and employed X-ray diffraction and scanning electron microscopy (SEM) to confirm the precipitation speciation. The findings of this work suggested that such an extract is effective in immobilizing heavy metals and preventing their diffusion into surrounding environments. Although there are limited studies on the immobilization of heavy metals by the EICP approach, higher concentrations of contaminants (i.e., > than 5 mmol/L in solution or 400 mg/kg in soil) have been neglected (Li et al., 2015; Kang and So, 2016; Zhu et al., 2017). The Pb contamination sites correspond to approximately 20% in all heavy metal–contaminated sites in mainland China, while the highest Pb concentration measured near a smelter in the Fujian province was up to 30,430 mg/kg (Duan et al., 2016). Therefore, an efficient, economical measure to remediate the heavy metal–contaminated sites is of great necessity to protect fragile environments. However, commercial and bacteria-derived sources of the urease enzyme are either expensive or cumbersome (Nafisi et al., 2019; Wu et al., 2020). The objectives of this study are as follows: 1) to investigate the effects of the urease concentration and calcium source on the enzyme-induced carbonate precipitation for high concentration Pb remediation through a series of preliminary test tube experiments using the plant-derived urease enzyme as the catalyst and 2) to identify the speciation and sequence of carbonate precipitation using Visual MINTEQ simulations toward revealing the mechanisms affecting the Pb remediation efficiency.
Materials and Methods
Urease Enzyme Extraction
Canavalia ensiformis was used in this study to prepare the urease enzyme using simple, economical extraction processes, including crushing, sieving, primary centrifugation, and secondary centrifugation (Yuan et al., 2020). The details are as follows: 1) Canavalia ensiformis was crushed using the plant grinding machine, thereby sieving with a mesh sieve (apertures = 0.15 mm); 2) the jack bean and ethanol solution were mixed in a ratio of 1:10 (g: ml), and the mixture solution was centrifuged for 30 min and then stored in the refrigerator for 4 h; 3) after that, the solution was centrifuged again for 1 h, and the precipitation of secondary centrifugation was the urease used in this study. It should be noted that the urease enzyme should be stored in a refrigerator at −20°C prior to its use. The urease enzyme is suggested to be used within 24 h after it is derived.
Urease Activity Tests
At standard conditions (30°C), 1 international unit (IU) of the enzyme activity is the amount of 1.0 μmol catalyzed transformation in 1 min (Mobley and Hausinger, 1989). Furthermore, a modified measure, proposed by Van Paassen (2011), was also used to enhance the accuracy of urease activity measurements. For the test, 1 ml of urease solution was mixed with 9 ml 1.11 mol/L urea, with electrical conductivity (EC), measured at 5, 10, and 15 min after mixing at room temperature. The modified measure can be expressed as follows:
where EC5, EC10, and EC15 are electrical conductivities at 5, 10, and 15 min, respectively. The modified electrical conductivity method yielded the urease activity being 99.8 mM Urea min-1, while the Nessler’s reagent colorimetric method yielded a urease activity of 342.7 U/g. The former reflects the amount of urea hydrolyzed per minute. However, the latter reflects the required amount of enzyme converting 1 μM of the substrate using a specialized unit U/g. For this reason, the two values are not found on the same basis, although the Nessler’s reagent colorimetric method adopted in the present work can describe the urease activity in a more straightforward manner. On the other hand, the NH4+ concentration was measured via a combination of the Nessler’s reagent and spectrophotometer, which aims to describe the degree of urea hydrolysis.
Test Tube Experiments
The effects of urease concentration and the calcium source on Pb remediation were investigated through a series of test tube experiments. Three urease concentrations (3 g/L, 6 g/L, and 9 g/L) and three calcium sources (CaCl2, Ca(CH3COO)2, and CaO) were adopted in the test tube experiments. The calcium source concentration used in the test tube experiments is 0.25 mol/L, while the urea concentration is 0.5 mol/L. Lead contaminant (Pb(NO3)2) concentrations adopted include both the low and high ranges, namely, 5 mmol/L, 10 mmol/L, 30 mmol/L, 40 mmol/L, and 50 mmol/L. The solution applied to the test tube experiments consisted of distilled water, urea, Pb(NO3)2, CaCl2, and the urease enzyme. The preparation of the solution is depicted in Supplementary Figure S1. Catalyzing urea hydrolysis was handled at an indoor temperature of about 26°C and was conducted for a 48-h period, following inoculation. The measurements of pH, NH4+ concentration, and remaining Pb2+ concentration were conducted after 48 h. It is widely accepted that NH4+ and OH− are the products of urea hydrolysis using the EICP technology, and they determine whether comprehensive urea hydrolysis is attained. The degree of urea hydrolysis not only dominates the amount of carbonate precipitation but also reflects the remaining Pb2+ concentration and remediation efficiency. The more the Pb2+ precipitated with the carbonate, the lesser will be the remaining Pb2+ and the higher the remediation efficiency. Prior to the measurement of the NH4+ concentration, the mixed solution was centrifuged and acidified to a pH lower than 2.0 for the sake of NH4+ measurement. Furthermore, the mixed solution was diluted 100–500 times, allowing the measurement to be undertaken. It was worth noting that all the precipitations were separated from the solution through the vacuum filtration method at the end of the tests and dried at 30°C for 3 days before being subjected to mass measurement (Keykha et al., 2018). All aforementioned tests had three biological replicates with coefficient of variance (COV) < 10%.
Visual MINTEQ Simulation
Given that the test tube experiments are not capable of providing the speciation and sequence of carbonate precipitation, it was simulated using the Visual MINTEQ software package. Here, urea hydrolysis was modeled as the initial condition about NH4+ and CO32− with a stoichiometric ratio of 2:1 (Gat et al., 2017). The simulation for the evolution of the precipitation speciation was determined by the degrees of urea hydrolysis that require the inputs of NH4+ and CO32− concentrations toward differentiating the abiotic precipitation from the biotic precipitation that directly plays roles in urea hydrolysis (Xue et al., 2022).
Results
Relationship of Urease Concentration versus Lead Remediation Efficiency
It is widely accepted that ammonium (NH4+) and hydroxide (OH−) ions are the products of urea hydrolysis using the EICP technology (Eqs 1–3), and therefore, the NH4+ concentration and pH are considered appropriate in determining whether comprehensive urea hydrolysis is attained. Its measurements were conducted during the EICP process. The variations of NH4+ concentration and pH when subjected to no urease and urease at concentrations ranging from 3 g/L to 9 g/L are shown in Figures 1A,B. Prior to introducing urease, the pH is always lower than 6 and appears insensitive to the concentration of Pb(NO3)2. However, the pH increases and surpasses 6 while catalyzing urea hydrolysis using the urease enzyme. Furthermore, the pH is generally increased with the increase of the urease concentration, and for urease at 3 g/L, there is a decrease when subjected to Pb(NO3)2 at 30 mmol/L (see Figure 1A). On the other hand, the urease is also on the way to catalyze urea hydrolysis toward producing NH4+ (see Figure 1B). Furthermore, the NH4+ concentration is generally decreased with the increase of the Pb(NO3)2 concentration. A significant drop in the Pb(NO3)2 concentration is also present when Pb(NO3)2 is at 30 mmol/L. Such a drop is not significant when subjected to higher urease concentrations (e.g. > 3 g/L). The variations of the measured precipitation mass when subjected to urease at 3 g/L, 6 g/L, and 9 g/L are shown in Figure 1C. It is clear that the precipitation mass is increased with the increase of the urease concentration and its maximum being 0.14 g, resulting from the urease at 9 g/L, presents when the Pb(NO3)2 concentration is at 50 mmol/L. Furthermore, the produced precipitation mass is generally increased with the increase of the Pb(NO3)2 concentration.
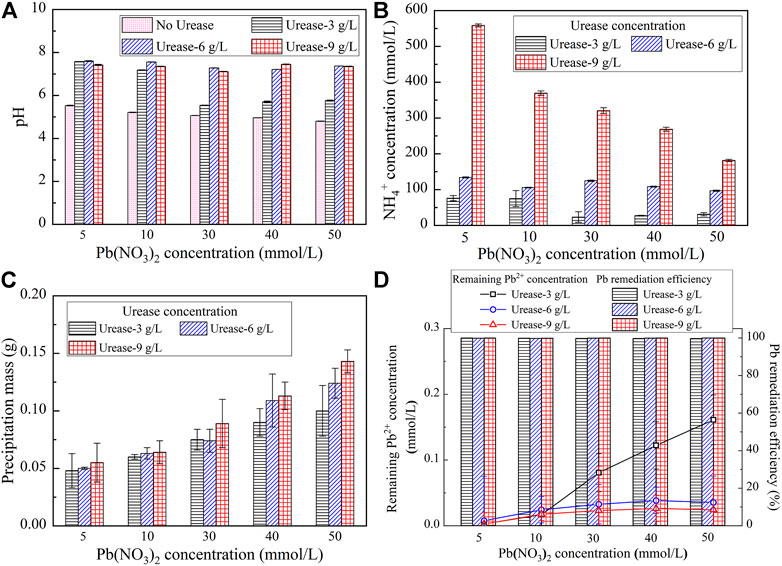
FIGURE 1. (A) Relationship of pH versus Pb(NO3)2 concentration; (B) Relationship of the NH4+ concentration versus Pb(NO3)2 concentration; (C) Relationship of the precipitation mass versus Pb(NO3)2 concentration; and (D) Relationship of the Pb2+ concentration and Pb remediation efficiency versus Pb(NO3)2 concentration.
To assess the effectiveness of Pb remediation, a remediation efficiency defined as a ratio of the remaining Pb2+ concentration to the initial Pb2+ concentration was used in the present study. The lower the remaining Pb2+ concentration, the higher is the Pb2+ remediation efficiency. The variations of the remaining Pb2+ concentration and Pb remediation efficiency when subjected to urease at 3 g/L, 6 g/L, and 9 g/L, respectively, are shown in Figure 1D. The relationship of the remaining Pb2+ concentration versus Pb(NO3)2 concentration for the urease at 3 g/L lies above that for the urease at 6 g/L which is underlaid by that for the urease at 9 g/L. These results show that the higher the urease concentration, the lower is the remaining Pb2+ concentration. By measuring the remaining Pb ion concentration, the remediation efficiency against different urease concentrations is measured to be higher than 99.5%. Its maximum presents when the urease is at 9 g/L and reaches approximately 100%.
Relationship of Calcium Source Versus Lead Remediation Efficiency
Given that the effect of the calcium source is considered crucial in improving Pb remediation, three calcium sources, including calcium chloride (CaCl2), calcium acetate (Ca(CH3COO)2), and calcium oxide (CaO), were taken into account in the test tube experiments. Despite the Pb remediation efficiency being approximately 100% at 9 g/L, an efficiency being higher than 99.5% was derived at 3 g/L. The efficiency of 3 g/L was, therefore, adopted in the following analysis for the sake of economy. The variations of pH and NH4+ concentrations when subjected to CaCl2, Ca(CH3COO)2, and CaO are shown in Figure 2. The pH, while catalyzing urea hydrolysis using urease, is always in excess of that prior to the urea hydrolysis except the case of calcium oxide (see Figures 2A–C). Furthermore, the pH is decreased with the increase of the Pb(NO3)2 concentration. Moreover, for the same Pb(NO3)2 concentration, the NH4+ concentration is the highest when subjected to Ca(CH3COO)2 (see Figure 2D). The NH4+ concentration is the lowest when subjected to CaO.
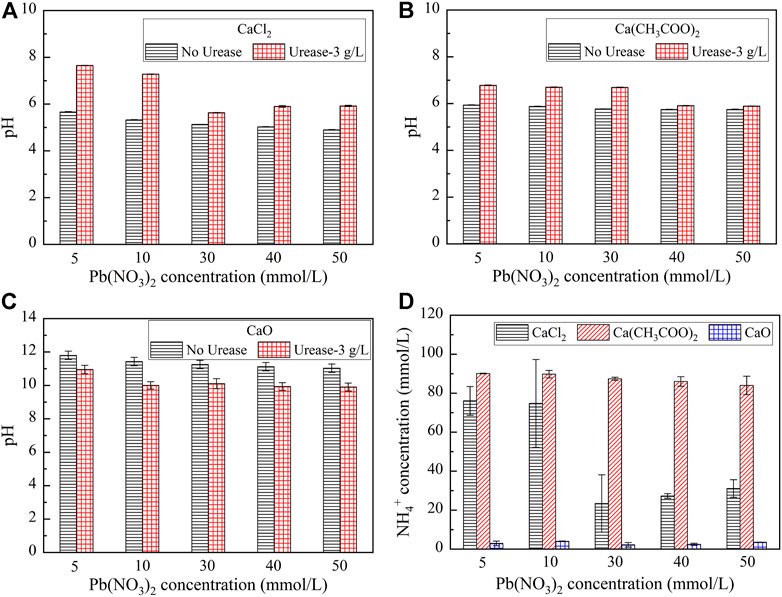
FIGURE 2. Relationship of pH and NH4+ concentrations versus the Pb(NO3)2 concentration considering different calcium sources: (A) CaCl2; (B) Ca(CH3COO)2; (C) CaO; and (D) Relationship of the NH4+ concentration versus Pb(NO3)2 concentration.
The variations of the measured carbonate precipitation mass when subjected to CaCl2, Ca(CH3COO)2, and CaO are shown in Figure 3A. The results indicate that the carbonate precipitation mass, when subjected to CaO, is much greater than that subjected to CaCl2 and Ca(CH3COO)2, and its maximum reaches 0.23 g. Furthermore, the precipitation mass is generally increased with the increase in the Pb(NO3)2 concentration. The variations of the remaining Pb2+ concentration and Pb remediation efficiency, when subjected to CaCl2, Ca(CH3COO)2, and CaO, are shown in Figure 3B. The relationship of the remaining Pb2+ concentration versus Pb(NO3)2 concentration, when subjected to Ca(CH3COO)2 and CaO, lies above that subjected to CaCl2. The Pb remediation efficiency, when subjected to different calcium sources, performs very well and is generally in excess of 70%. The best remediation efficiency measures at about 100%.
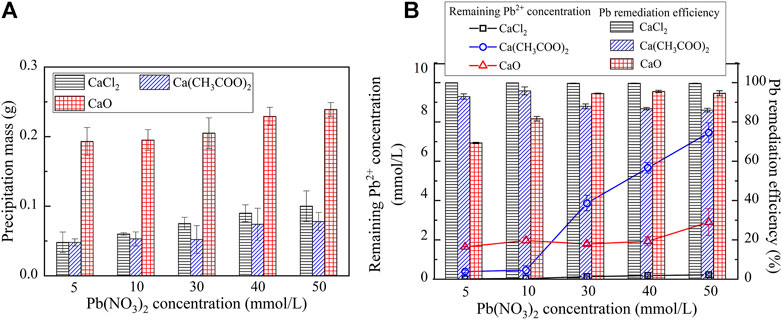
FIGURE 3. (A) Relationship of the precipitation mass versus Pb(NO3)2 concentration and (B) Relationship of the Pb2+ concentration and remediation efficiency versus Pb(NO3)2 concentration.
Visual MINTEQ Simulation
Carbonate precipitations, including abiotic precipitation and biotic precipitation, in the test tube experiments, were reproduced using the Visual MINTEQ software package, investigating its speciation during the EICP process. Understanding the speciation of precipitations is considered of great necessity for revealing the mechanisms affecting Pb remediation. The reproduced carbonate precipitations, when subjected to urease at 3 g/L, 6 g/L, and 9 g/L, are shown in Figure 4. There are four precipitation speciations present, including PbCl2, hydrocerrusite (Pb3(CO3)2(OH)2), lead carbonate (PbCO3), and calcium carbonate (CaCO3) (Eqs 4, 6, 7, 8). PbCl2 is categorized as abiotic precipitation since it is formed prior to introducing urease, while Pb3(CO3)2(OH)2, PbCO3, and CaCO3 are, therefore, classed as biotic precipitation. Furthermore, accompanied with the increase of the urease concentration, the precipitation speciation starts transforming. For example, PbCl2 is transformed to Pb3(CO3)2(OH)2 in the first place when the urease concentration is increased from 3 g/L to 6 g/L (see Figures 4A–C). Subsequently, Pb3(CO3)2(OH)2 is transformed to PbCO3 when the urease concentration is increased from 6 g/L to 9 g/L. Moreover, except CaCO3 the concentrations of PbCl2, PbCO3, and Pb3(CO3)2(OH)2 are increased with the increase in the Pb(NO3)2 concentration. The simulated results indicate that the remediation efficiency against different urease concentrations performs very well and in excess of 99% on average, which is in line with the experimental results (see Figures 1D, 4D).
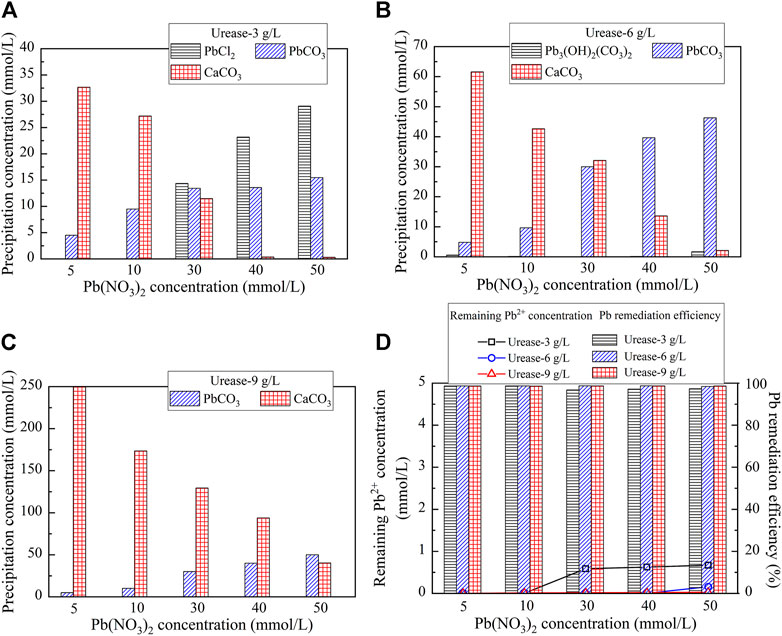
FIGURE 4. Visual MINTEQ simulation under different urease concentrations: (A) 3 g/L; (B) 6 g/L; (C) 9 g/L; and (D) remaining Pb2+ concentration and remediation efficiency.
On the other hand, the reproduced carbonate precipitations when subjected to CaCl2, Ca(CH3COO)2, and CaO are shown in Figure 5. Unlike the previous simulation, there are six speciations of carbonate precipitation, including PbCl2, Pb(OH)2, Pb3(CO3)2(OH)2, PbCO3, Ca(OH)2, and CaCO3, when subjected to the effect of the calcium source (Eqs 5–10). PbCl2, Pb(OH)2, and Ca(OH)2 are precipitated before catalyzing urea hydrolysis and are therefore classed as abiotic precipitations, while CaCO3, Pb3(CO3)2(OH)2, and PbCO3 are categorized as biotic precipitations. Furthermore, except CaCO3 and Ca(OH)2, the concentrations of PbCl2, Pb(OH)2, Pb3(CO3)2(OH)2, and PbCO3 are increased with the increase in the concentration of Pb(NO3)2 (see Figures 5A–C). Last but not least, the remediation efficiency against different calcium sources surpasses 90% (see Figure 5D). There appears a discrepancy between the simulated results and the experimental ones (see Figures 3B, 5D).
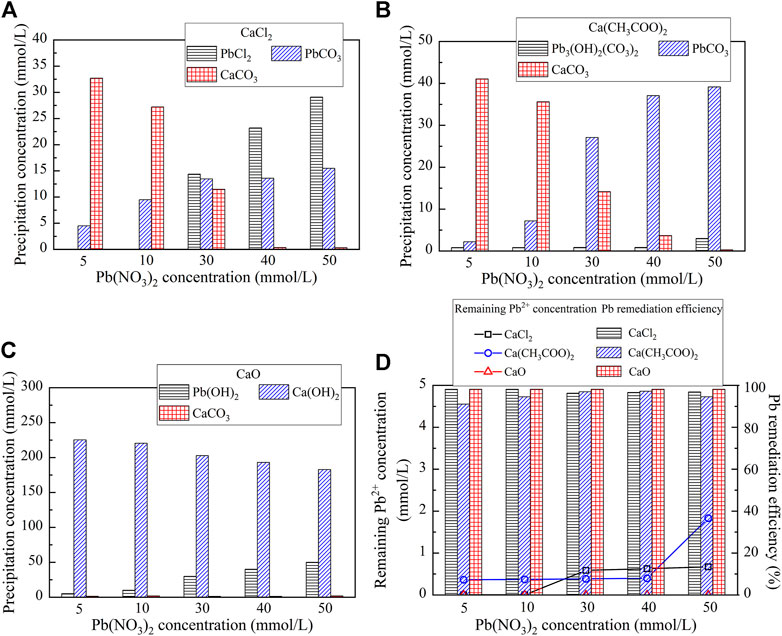
FIGURE 5. Visual MINTEQ simulation under different calcium sources: (A) CaCl2; (B) Ca(CH3COO)2; (C) CaO; and (D) remaining Pb2+ concentration and remediation efficiency.
Discussion
Effect of Urease Concentration
It can be seen from Figure 1A that the pH values for urease at 9 g/L are always higher than those for urease at 3 g/L, indicating a more comprehensive urea hydrolysis. The pH, when subjected to urease at 6 g/L and 9 g/L, respectively, shows a small change when the concentration of Pb(NO3)2 is increased from 5 mmol/L to 50 mmol/L. In contrast, when subjected to urease at 3 g/L, there appears a decrease in the pH of Pb(NO3)2 at 30 mmol/L. The lower the urease concentration, the more significant is the effect of Pb ions. A similar decrease can also be seen in Figure 1B; when subjected to urease at 9 g/L and 6 g/L, respectively, the NH4+ concentration generally decreases with the increase in the Pb(NO3)2 concentration. However, when subjected to urease at 3 g/L, there appears a NH4+ concentration drop in Pb(NO3)2 at 30 mmol/L. These results indicate that the effect of Pb ions can depress urea hydrolysis and becomes more significant when subjected to lower urease concentrations. In the present work, Pb(NO3)2 at or higher than 30 mmol/L starts depressing urea hydrolysis when subjected to urease at 3 g/L. Urease is essentially protein, and heavy metal ions can badly inactivate it by reacting with its sulfhydryl group (Shaw and Raval, 1961; Chung et al., 2020). Given that the NH4+ concentration reflects whether comprehensive urea hydrolysis is attained, enhancing our understanding of how the urease activity, when subjected to the effect of Pb ions, affects the production of NH4+ is considered of great necessity. For this reason, the relation of the urease activity and NH4+ concentration versus time is explored, as shown in Supplementary Figure S2. It can be observed that the degradation of the urease activity, when subjected to the Pb ions, is not instantaneous (Yuan et al., 2020) and can be further divided into three phases, including the rapid degradation phase, gentle degradation phase, and limited degradation phase, according to the change of the NH4+ concentration. During the rapid degradation phase, the Pb ions cause the urease activity to decrease very quickly, while the NH4+ concentration is increased over time, indicating that urease has not been inactivated yet. The urease activity decreases progressively during the gentle degradation phase where the increase of the NH4+ concentration due to the reduced urease activity is not as significant as before. In the limited degradation phase, the NH4+ concentration shows a small change, indicating that urease has already been inactivated.
It is well acknowledged that the degradation of the urease activity is accompanied by carbonate precipitation, and the speciation of carbonate precipitation determines whether a fairly good Pb remediation is attained. The following content aims to address how the speciation of carbonate precipitation affects the Pb remediation efficiency. The simulated results indicate that the speciation of carbonate precipitation, when subjected to the urease concentration at 9 g/L, includes PbCO3 and CaCO3, and they are classed as biotic precipitations. The biotic precipitations are relatively stable compared to the abiotic ones, according to their solubility product (termed Ksp hereafter). Their transformation is, therefore, not going to happen (Jiang et al., 2019; Xue et al., 2022). Provided that higher urease concentrations ensure the availability of CO32−, a fairly satisfactory Pb remediation can be expected. In contrast, the speciation of carbonate precipitation under the urease concentration at 3 g/L comprises PbCl2, CaCO3, and PbCO3. Two of them (CaCO3 and PbCO3) are categorized as biotic precipitations and PbCl2 as an abiotic one. The Pb remediation efficiencies under 3 g/L and 9 g/L urease concentrations are approximately 100%, although the associated precipitation speciation differs from each other. Given that Ksp (PbCl2) being 1.6 × 10–6 is greater than Ksp (PbCO3) being 7.4 × 10–14, PbCl2 is more likely to be dissolved and converted to other chemical substances compared with PbCO3. Thus, PbCO3 is deemed as a desirable precipitation compound rather than PbCl2. To summarize, the effect of Pb ions depresses urea hydrolysis and becomes more significant when subjected to lower urease concentrations. Higher urease concentrations ensure the availability of CO32−, contributing to a formation of a relatively stable biotic precipitation.
Effect of Calcium Source
As the calcium source can affect the speciation of carbonate precipitation, measurements of pH and NH4+ concentrations can benefit us in enhancing our understanding of the effect of the calcium source on Pb remediation (Jiang et al., 2019). For a given Pb(NO3)2 concentration, the pH is measured the highest when subjected to CaO, followed by CaCl2 (see Figures 2A,C), while the pH is measured the lowest when subjected to Ca(CH3COO)2 (see Figure 2B). The highest pH derived from CaO appears to indicate the comprehensive urea hydrolysis. In fact, while using CaO as the calcium source, the CaO reacts with H2O to form Ca(OH)2 toward elevating the value of pH. This is in line with the measurements of pH. The pH reaches a value as high as 12 (see Figure 2C). Notwithstanding this fact, such a high pH depresses the urease activity, resulting in a reduction of the NH4+ concentration (Mobley and Hausinger, 1989; Neupane et al., 2013; and Almajed et al., 2018) (see Figure 2D). The measured results of the NH4+ concentration also confirm this statement. On the other hand, the NH4+ concentration for a given Pb(NO3)2 concentration is measured the highest under Ca(CH3COO)2, followed by CaCl2. As a result, the calcium source depresses the urease activity by influencing the surrounding pH, resulting in a reduction of the NH4+ concentration.
The speciation of carbonate precipitation is also explored here to extend the interpretation of the experimental and simulated results. The simulated results indicate that the speciation of the carbonate precipitation, when subjected to the calcium source of CaO, includes Pb(OH)2, Ca(OH)2, and CaCO3 where the former two are classed as abiotic precipitations. Ca(OH)2 is formed before Pb(OH)2 due to the addition sequence of calcium sources. Given that Ksp (Ca(OH)2) being 5.5 × 10–6 is greater than Ksp (Pb(OH)2) being 1.2 × 10–15, Ca(OH)2 tends to transform to Pb(OH)2. However, Pb(OH)2 is deemed soluble when exposed to alkaline environments (Edwards et al., 1992; Baltpurvins et al., 1996; Vítková et al., 2009; and Wang et al., 2022). When Pb(OH)2 is just formed following the aforesaid transformation, and it is also on the way to dissolve, thereby releasing the Pb ions into the solution. This also means a degradation of the Pb remediation. Furthermore, the speciation of carbonate precipitation under the calcium source of Ca(CH3COO)2 comprises Pb3(CO3)2(OH)2, PbCO3, and CaCO3, and they are classed as biotic precipitations. Under the calcium source of CaCl2, two of them (CaCO3 and PbCO3) are categorized as biotic precipitations and PbCl2 as an abiotic one. The abiotic precipitation compensates for the deficiency of carbonate precipitation caused by the lack of CO32-. Therefore, the formation of biotic and abiotic precipitation results in the highest Pb remediation efficiency although the NH4+ concentration is not the highest under the calcium source of CaCl2.
Generally, the higher the precipitation mass, the higher is the remediation efficiency. However, this sometimes causes a misleading interference while assessing the Pb remediation efficiency. In the present work, there appears a discrepancy of Pb remediation between the experimental and simulated results (see Figures 3B, 5D). Despite this fact, the highest precipitation mass against different Pb(NO3)2 concentrations is derived using CaO, and the highest remediation efficiency is, however, derived using CaCl2. In light of this, the Pb remediation is not only determined by the precipitation mass but also by the speciation of precipitation. As discussed, the dissolution of Pb(OH)2, while using CaO, releases free Pb ions and subsequently degrades Pb remediation, which has been neglected in the simulation. This is considered as the main cause leading to the discrepancy between the experimental and simulated results.
Mechanisms Affecting Lead Remediation
The change of the NH4+ concentration confirms that the Pb ions notably depress the urease activity, and the effect of the Pb ions becomes more significant when subjected to lower urease concentrations (see Figure 6A). In these circumstances, the availability of CO32− cannot be consistently assured during the EICP process, thereby degrading Pb remediation. These results indicate that higher urease concentrations not only counterbalance the effect of the Pb ions but also assure the availability of CO32− toward securing a formation of the relatively stable biotic precipitation. On the other hand, the highest pH is derived using CaO, most likely because of the elevation of pH by Ca(OH)2. The urease activity is depressed accordingly, and when exposed to alkaline environments, the dissolution of Pb(OH)2 releases Pb ions to degrade Pb remediation (see Figure 6B). The highest Pb remediation is attained using CaCl2 where the pH is measured in the second place. In short, a combination of higher urease concentrations and an appropriate calcium source may be used to prevent the degradation of Pb remediation.
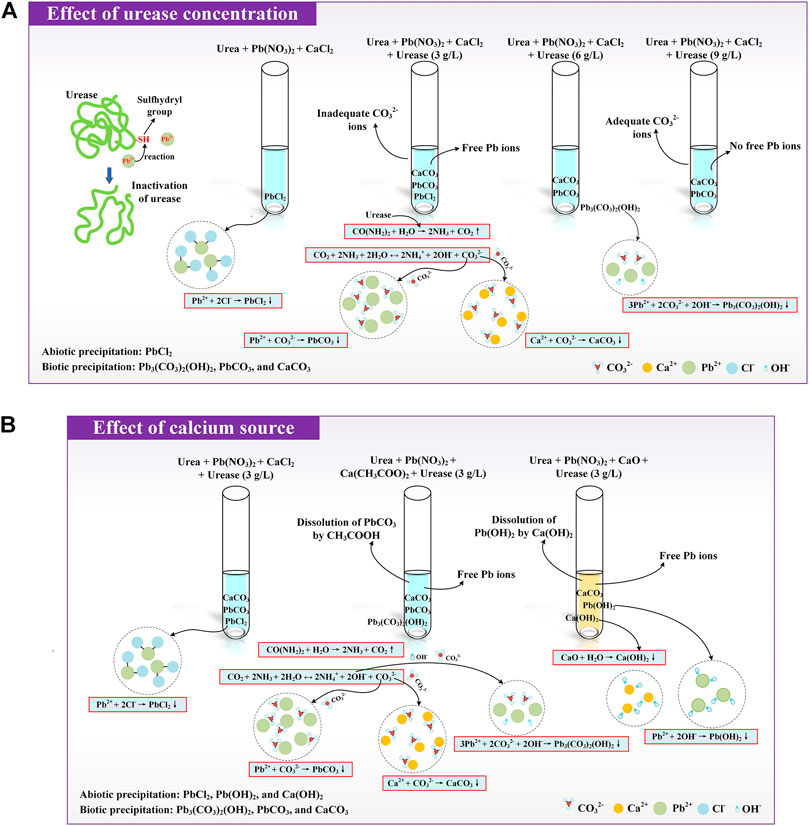
FIGURE 6. (A) Schematic illustration of the effect of urease concentration on Pb remediation and (B) Schematic illustration of the effect of the calcium source on Pb remediation.
In addition to the effects of the urease concentration and calcium source, the precipitation mass also has implications on Pb remediation. The precipitation mass under the urease concentration at 9 g/L is higher than that under the urease concentration at 3 g/L, making the discrepancy in Pb remediation efficiency negligible. Meanwhile, the highest precipitation mass against different calcium sources is attained using CaO, and the highest Pb remediation is, however, attained using CaCl2. These results confirm that Pb remediation is not only determined by the precipitation mass but also by other influencing factors (e.g., speciation and sequence of carbonate precipitation). When subjected to the urease concentration at 3 g/L, PbCl2 first precipitates to form the inner layer of a multi-layer structure, followed by PbCO3 and CaCO3 to form its outer layer (see Figures 7A–C). The multi-layer structure prevents the migration of Pb ions, although a small number of free Pb ions is released as a result of the inadequacy of CO32−. In contrast, the multi-layer structure of carbonate precipitation under urease at 9 g/L can be recognized as PbCO3 and CaCO3 forming the inner and outer layers, respectively, thereby preventing the migration of Pb ions. On the other hand, Pb3(CO3)2(OH)2, when subjected to Ca(CH3COO)2, precipitates in the first place, followed by PbCO3 and CaCO3 (see Figures 7D–F). When subjected to CaO, the carbonate precipitation sequence can be sorted as Ca(OH)2, Pb(OH)2, and CaCO3. However, Pb(OH)2 is dissolved in alkaline conditions resulting from Ca(OH)2, and Pb ions are released, degrading Pb remediation (Edwards et al., 1992; Baltpurvins et al., 1996; Vítková et al., 2009). Moreover, PbCl2 under CaCl2 first precipitates, followed by PbCO3 and CaCO3, meaning that a multi-layer structure of carbonate precipitation is developed, and PbCl2 forms the inner layer, covered by the two outer layers, including PbCO3 and CaCO3. The multi-layer structure of the carbonate precipitation encapsulates the Pb ions and causes some difficulty for the Pb ions to migrate, stabilizing the Pb ions and preventing the degradation of Pb remediation. The multi-layer structure of carbonate precipitation, when subjected to CaO, would have been more effective in stabilizing the Pb ions if the dissolution of Pb(OH)2 had not happened.
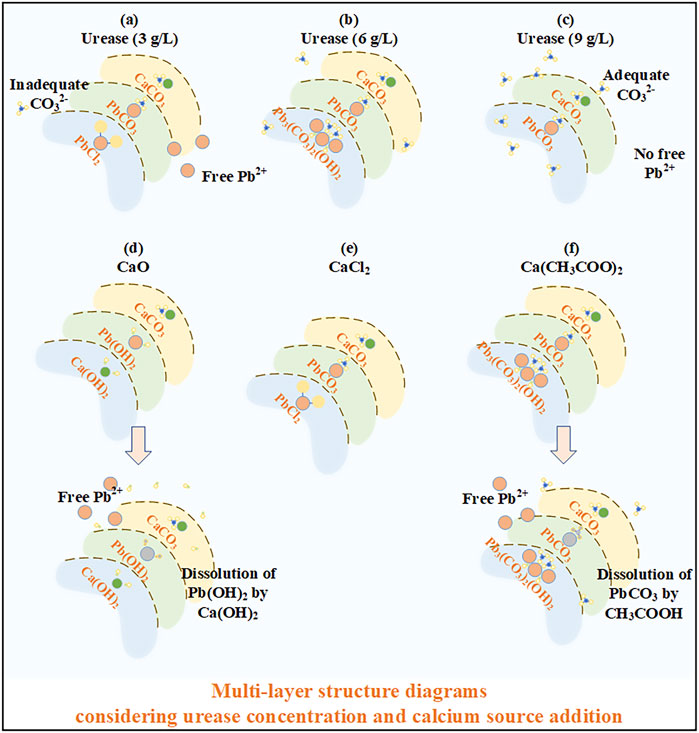
FIGURE 7. Schematic illustration of the multi-layer structure of carbonate precipitation: (A) urease at 3 g/L; (B) urease at 6 g/L; (C) urease at 9 g/L; (D) CaO; (E) CaCl2; and (F) Ca(CH3COO)2.
On the whole, Pb ions depress the urease activity, and the effect of Pb ions turns into a more pronounced contributor when subjected to lower urease concentrations. Higher urease concentrations can consistently assure the availability of CO32− during the EICP process. The use of a calcium source can affect the speciation of carbonate precipitation. In some cases, an inappropriate calcium source can cause difficulty in developing the multi-layer structure of carbonate precipitation, thereby degrading Pb remediation. The use of higher urease concentrations and an appropriate calcium source can prevent the degradation of Pb remediation. Furthermore, Pb remediation is not only determined by the precipitation mass but also by other influencing factors. The precipitation sequence plays a role in the formation of the multi-layer structure of carbonate precipitation. The multi-layer structure capsulizes Pb ions and, therefore, prevents their migration, securing the Pb remediation efficiency. The robustness of the multi-layer structure of carbonate precipitation cannot be quantitatively assessed by a micro-structural analysis but by the speciation analysis of Pb2+. Details about the results of the micro-structural and speciation analyses are not within the scope of the present work and would be discussed in another article.
Conclusion
This article has investigated the effects of the urease concentration and a calcium source on Pb remediation. The speciation and sequence of carbonate precipitation have been explored to highlight the mechanisms leading to the degradation of Pb remediation. Based on the results and discussion, some main conclusions can be drawn as follows:
1) The NH4+ concentration presents good correspondence with the urease concentration. As indicated by the NH4+ concentration, the Pb ions depress the urease activity, and the effect of Pb ions becomes more significant when provided with lower urease concentrations. Higher urease concentrations can assure the availability of CO32− during the EICP process toward benefiting carbonate precipitation.
2) The calcium source, in fact, determines the speciation of carbonate precipitation and subsequently the Pb remediation efficiency. The Pb remediation efficiency is not only determined by the precipitation mass but also by the other influencing factors (e.g., precipitation sequence). The dissolution of Pb(OH)2, when subjected to CaO, has been neglected in the Visual MINTEQ simulation, causing a discrepancy of Pb remediation between the experimental and simulated results. In the present study, the highest Pb remediation efficiency is attained using CaCl2. The abiotic precipitation compensates for the deficiency of carbonate precipitation caused by the lack of CO32−.
3) The use of CaO results in the dissolution of Pb(OH)2 and, therefore, releases Pb ions, causing some difficulty in forming the multi-layer structure of carbonate precipitation and degrading Pb remediation. Given that PbCl2 is precipitated first, followed by PbCO3 and CaCO3, when subjected to CaCl2, the multi-layer structure of carbonate precipitation capsulizes the Pb ions and prevents their migration, securing Pb remediation. The present work highlights the exciting potential of applying the EICP technology to Pb ion removal. Further work of stabilizing Pb ions in the contaminated sites by the EICP technology is ongoing and would be discussed in another article.
Data Availability Statement
The original contributions presented in the study are included in the article/Supplementary Material, further inquiries can be directed to the corresponding author.
Author Contributions
LW: data curation, formal analysis, validation, software, and writing—original draft. W-CC: conceptualization, methodology, writing—review and editing, supervision, and funding acquisition. Z-FX: writing—review and editing. WH: writing—review and editing.
Funding
This study is based upon work supported by the Shaanxi Educational Department under Grant No. 2020TD-005 through the innovative ability support scheme.
Conflict of Interest
The authors declare that the research was conducted in the absence of any commercial or financial relationships that could be construed as a potential conflict of interest.
Publisher’s Note
All claims expressed in this article are solely those of the authors and do not necessarily represent those of their affiliated organizations, or those of the publisher, the editors, and the reviewers. Any product that may be evaluated in this article, or claim that may be made by its manufacturer, is not guaranteed or endorsed by the publisher.
Supplementary Material
The Supplementary Material for this article can be found online at: https://www.frontiersin.org/articles/10.3389/fchem.2022.892090/full#supplementary-material
References
Achal, V., Mukerjee, A., and Sudhakara Reddy, M. (2013). Biogenic Treatment Improves the Durability and Remediates the Cracks of concrete Structures. Construction Building Mater. 48, 1–5. doi:10.1016/j.conbuildmat.2013.06.061
Achal, V., Pan, X., Fu, Q., and Zhang, D. (2012). Biomineralization Based Remediation of As(III) Contaminated Soil by Sporosarcina Ginsengisoli. J. Hazard. Mater. 201, 178–184. doi:10.1016/j.jhazmat.2011.11.067
Ahenkorah, I., Rahman, M. M., Karim, M. R., and Beecham, S. (2021a). Optimisation of Chemical Constituents on Enzyme-Induced Carbonate Precipitation in Test-Tube and Soil. Geotechnical Res. 5 (3), 1–19. doi:10.1680/jgere.21.00006
Ahenkorah, I., Rahman, M. M., Karim, M. R., Beecham, S., and Saint, C. (2021b). A Review of Enzyme Induced Carbonate Precipitation (EICP): The Role of Enzyme Kinetics. Sustain. Chem. 2, 92–114. doi:10.3390/suschem2010007
Almajed, A., Khodadadi Tirkolaei, H., and Kavazanjian, E. (2018). Baseline Investigation on Enzyme-Induced Calcium Carbonate Precipitation. J. Geotech. Geoenviron. Eng. 144 (11), 04018081. doi:10.1061/(asce)gt.1943-5606.0001973
An, Q., Deng, S., Xu, J., Nan, H., Li, Z., and Song, J. L. (2019). Simultaneous Reduction of Nitrate and Cr(VI) by Pseudomonas aeruginosa Strain G12 in Wastewater. Ecotoxicol Environ. Saf. 191, 110001. doi:10.1016/j.ecoenv.2019.110001
Bai, B., Long, F., Rao, D. Y., and Xu, T. (2017). The Effect of Temperature on the Seepage Transport of Suspended Prticles in a Porous Medium. Hydrol Process 31 2, 382–393. doi:10.1002/hyp.11034
Bai, B., Nie, Q. K., Zhang, Y. K., Wang, X. L., and Xu, W. (2021). Cotransport of Heavy Metals and SiO2 Particles at Different Temperatures by Seepage. J. Hydrol. 597, 125771. doi:10.1016/j.jhydrol.2020.125771
Bai, X. D., Cheng, W. C., and Li, G. (2021). A Comparative Study of Different Machine Learning Algorithms in Predicting EPB Shield Behavior: A Case Study at Xi’an Metro, China. Acta geotechnica 16 12, 4061–4080. doi:10.1007/s11440-021-01383-7
Baltpurvins, K. A., Burns, R. C., Lawrance, G. A., and Stuart, A. D. (1996). Use of the Solubility Domain Approach for the Modeling of the Hydroxide Precipitation of Heavy Metals from Wastewater. Environ. Sci. Technol. 30, 1493–1499. doi:10.1021/es950421u
Chang, J., Deng, S., Liang, Y., and Chen, J. (2019). Cr(VI) Removal Performance from Aqueous Solution by Pseudomonas Sp. Strain DC-B3 Isolated from Mine Soil: Characterization of Both Cr(VI) Bioreduction and Total Cr Biosorption Processes. Environ. Sci. Pollut. Res. 26 (27), 28135–28145. doi:10.1007/s11356-019-06017-w
Chen, L., Beiyuan, J., Hu, W., Zhang, Z., Duan, C., Cui, Q., et al. (2022a). Phytoremediation of Potentially Toxic Elements (PTEs) Contaminated Soils Using Alfalfa (Medicago Sativa L.): A Comprehensive Review. Chemosphere 293, 133577. doi:10.1016/j.chemosphere.2022.133577
Chen, L., Wang, J., Beiyuan, J., Guo, X., Wu, H., and Fang, L. (2022b). Environmental and Health Risk Assessment of Potentially Toxic Trace Elements in Soils Near Uranium (U) Mines: A Global Meta-Analysis. Sci. Total Environ. 816, 151556. doi:10.1016/j.scitotenv.2021.151556
Chen, Y., Gao, Y., Ng, C. W. W., and Guo, H. (2021). Bio-improved Hydraulic Properties of Sand Treated by Soybean Urease Induced Carbonate Precipitation and its Application Part 1: Water Retention Ability. Transportation Geotechnics 27, 100489. doi:10.1016/j.trgeo.2020.100489
Cheng, W. C., Duan, Z., Xue, Z. F., and Wang, L. (2021). Sandbox Modelling of Interactions of Landslide Deposit with Terrace Sediments Aided by Field Observation. Bulletin of Engineering Geology and the Environment 80, 100489. doi:10.1007/s10064-021-02144-2
Chung, H., Kim, S. H., and Nam, K. (2020). Inhibition of Urea Hydrolysis by Free Cu Concentration of Soil Solution in Microbially Induced Calcium Carbonate Precipitation. Sci. Total Environ. 740, 3711–3731. doi:10.1007/s10064-021-02144-2
Cui, M.-J., Lai, H.-J., Hoang, T., and Chu, J. (2021). One-phase-low-pH Enzyme Induced Carbonate Precipitation (EICP) Method for Soil Improvement. Acta Geotech. 16, 481–489. doi:10.1007/s11440-020-01043-2
Dhami, N. K., Reddy, M. S., and Mukherjee, A. (2013). Biomineralization of Calcium Carbonates and Their Engineered Applications: a Review. Front. Microbiol. 4, 314. doi:10.3389/fmicb.2013.00314
Duan, Q., Lee, J., Liu, Y., Chen, H., and Hu, H. (2016). Distribution of Heavy Metal Pollution in Surface Soil Samples in China: A Graphical Review. Bull. Environ. Contam. Toxicol. 97 (3), 303–309. doi:10.1007/s00128-016-1857-9
Duan, Z., Cheng, W. C., Peng, J. B., and Tang, H. (2021). Interactions of Landslide Deposit with Terrace Sediments: Perspectives from Velocity of Deposit Movement and Apparent Friction Angle. Engineering Geology 280, 105913. doi:10.1016/j.enggeo.2020.105913
Edwards, R., Gillard, R. D., Williams, P. A., and Pollard, A. M. (1992). Studies of Secondary mineral Formation in the PbO-H2o-HC1 System. Mineral. Mag. 56, 53–65. doi:10.1180/minmag.1992.056.382.07
Fisher, K. A., Yarwood, S. A., and James, B. R. (2017). Soil Urease Activity and Bacterial ureC Gene Copy Numbers: Effect of pH. Geoderma 285, 1–8. doi:10.1016/j.geoderma.2016.09.012
Fujita, Y., Taylor, J. L., Wendt, L. M., Reed, D. W., and Smith, R. W. (2010). Evaluating the Potential of Native Ureolytic Microbes to Remediate a 90Sr Contaminated Environment. Environ. Sci. Technol. 44, 7652–7658. doi:10.1021/es101752p
Gat, D., Ronen, Z., and Tsesarsky, M. (2017). Long-term Sustainability of Microbial-Induced CaCO3 Precipitation in Aqueous media. Chemosphere 184, 524–531. doi:10.1016/j.chemosphere.2017.06.015
Hamdan, N. E. K., and Kavazanjian, E. (2016). Enzyme-induced Carbonate mineral Precipitation for Fugitive Dust Control. Géotechnique 66 (7), 546–555. doi:10.1680/jgeot.15.p.168
Hoang, T., Alleman, J., Cetin, B., Ikuma, K., and Choi, S.-G. (2019). Sand and Silty-Sand Soil Stabilization Using Bacterial Enzyme-Induced Calcite Precipitation (BEICP). Can. Geotech. J. 56, 808–822. doi:10.1139/cgj-2018-0191
Hu, W., Cheng, W.-C., Wen, S., and Yuan, K. (2021). Revealing the Enhancement and Degradation Mechanisms Affecting the Performance of Carbonate Precipitation in EICP Process. Front. Bioeng. Biotechnol. 9, 750258. doi:10.3389/fbioe.2021.750258
Hu, X. F., Huang, X. R., Zhao, H. H., Liu, F. H., Wang, L., Zhao, X., et al. (2020). Possibility of Using Modified Fly Ash and Organic Fertilizers for Remediation of Heavy-Metal-Contaminated Soils. J. Clean. Prod. 284 (7), 124713. doi:10.1016/j.jclepro.2020.124713
Hu, W., Cheng, W. C., Wen, S., and Mizanur Rahman, N. (2021). Effects of Chemical Contamination on Microscale Structural Characteristics of Intact Loess and Resultant Macroscale Mechanical Properties. Catena 203, 105361. doi:10.1016/j.catena.2021.105361
Hu, W., Cheng, W. C., Wang, L., and Xue, Z. F. (2022). Micro-Structural Characteristics Deterioration of Intact Loess Under Acid and Saline Solutions and Resultant Macro-Mechanical Properties. Soil and Tillage Research 220, 105382. doi:10.1016/j.still.2022.105382
Jiang, N.-J., Liu, R., Du, Y.-J., and Bi, Y.-Z. (2019). Microbial Induced Carbonate Precipitation for Immobilizing Pb Contaminants: Toxic Effects on Bacterial Activity and Immobilization Efficiency. Sci. Total Environ. 672, 722–731. doi:10.1016/j.scitotenv.2019.03.294
Jiang, N. J., Wang, Y. J., Chu, J., Kawasaki, S., Tang, C. S., Cheng, L., et al. (2021). Bio-mediated Soil Improvement: An Introspection into Processes, Materials, Characterization and Applications. Soil Use Manage. 38, 12736. doi:10.1111/sum.12736
Kang, C.-H., and So, J.-S. (2016). Heavy Metal and Antibiotic Resistance of Ureolytic Bacteria and Their Immobilization of Heavy Metals. Ecol. Eng. 97, 304–312. doi:10.1016/j.ecoleng.2016.10.016
Keykha, H. A., Asadi, A., Huat, B. B. K., and Kawasaki, S. (2018). Laboratory Conditions Formaximal Calcium Carbonate Precipitation Induced by Sporosarcina Pasteurii and Sporosarcina Aquimarina Bacteria. Environ. Geotechnics 6 (8), 1–20. doi:10.1680/jenge.16.00009
Kim, S. O., Moon, S. H., and Kim, K. W. (2001). Removal of Heavy Metals from Soils Using Enhanced Electrokinetic Soil Processing. Water Air Soil Pollut. 125 (1), 259–272. doi:10.1023/a:1005283001877
Kumar, V., and Dwivedi, S. K. (2019). Hexavalent Chromium Stress Response, Reduction Capability and Bioremediation Potential of Trichoderma Sp. Isolated from Electroplating Wastewater. Ecotoxicology Environ. Saf. 185, 109734. doi:10.1016/j.ecoenv.2019.109734
Li, M., Fu, Q.-L., Zhang, Q., Achal, V., and Kawasaki, S. (2015). Bio-grout Based on Microbially Induced Sand Solidification by Means of Asparaginase Activity. Sci. Rep. 5, 16128. doi:10.1038/srep16128
Liu, B., Xie, Y.-H., Tang, C.-S., Pan, X.-H., Jiang, N.-J., Singh, D. N., et al. (2021). Bio-mediated Method for Improving Surface Erosion Resistance of Clayey Soils. Eng. Geology. 293, 106295. doi:10.1016/j.enggeo.2021.106295
Meng, H., Shu, S., Gao, Y., Yan, B., and He, J. (2021). Multiple-phase Enzyme-Induced Carbonate Precipitation (EICP) Method for Soil Improvement. Eng. Geology. 294, 106374. doi:10.1016/j.enggeo.2021.106374
Mobley, H. L., and Hausinger, R. P. (1989). Microbial Ureases: Significance, Regulation, and Molecular Characterization. Microbiol. Rev. 53 (1), 85–108. doi:10.1128/mr.53.1.85-108.1989
Moghal, A. A. B., Lateef, M. A., Mohammed, S. A. S., Ahmad, M., Usman, A. R. A., and Almajed, A. (2020b). Heavy Metal Immobilization Studies and Enhancement in Geotechnical Properties of Cohesive Soils by EICP Technique. Appl. Sci. 10 (7568), app10217568. doi:10.3390/app10217568
Moghal, A. A. B., Lateef, M. A., Mohammed, S. A. S., Lemboye, K., Chittoori, B. C. S., and Almajed, A. (2020a). Efficacy of Enzymatically Induced Calcium Carbonate Precipitation in the Retention of Heavy Metal Ions. Sustainability 12 (7019), su12177019. doi:10.3390/su12177019
Mohan, S. V., Kisa, T., Ohkuma, T., Kanaly, R. A., and Shimizu, Y. (2006). Bioremediation Technologies for Treatment of PAH-Contaminated Soil and Strategies to Enhance Process Efficiency. Rev. Environ. Sci. Biotechnol. 5 (4), 347–374. doi:10.1007/s11157-006-0004-1
Mugwar, A. J., and Harbottle, M. J. (2016). Toxicity Effects on Metal Sequestration by Microbially-Induced Carbonate Precipitation. J. Hazard. Mater. 314, 237–248. doi:10.1016/j.jhazmat.2016.04.039
Nafisi, A., Safavizadeh, S., and Montoya, B. M. (2019). Influence of Microbe and Enzyme-Induced Treatments on Cemented Sand Shear Response. J. Geotech. Geoenviron. Eng. 145 (9), 06019008. doi:10.1061/(asce)gt.1943-5606.0002111
Nam, I.-H., Roh, S.-B., Park, M.-J., Chon, C.-M., Kim, J.-G., Jeong, S.-W., et al. (2016). Immobilization of Heavy Metal Contaminated Mine Wastes Using Canavalia Ensiformis Extract. Catena 136, 53–58. doi:10.1016/j.catena.2015.07.019
Neupane, D., Yasuhara, H., Kinoshita, N., and Unno, T. (2013). Applicability of Enzymatic Calcium Carbonate Precipitation as a Soil-Strengthening Technique. J. Geotech. Geoenviron. Eng. 139 (12), 2201–2211. doi:10.1061/(asce)gt.1943-5606.0000959
Phillips, A. J., Gerlach, R., Lauchnor, E., Mitchell, A. C., Cunningham, A. B., and Spangler, L. (2013). Engineered Applications of Ureolytic Biomineralization: a Review. Biofouling 29, 715–733. doi:10.1080/08927014.2013.796550
Putra, H., Yasuhara, H., Kinoshita, N., Neupane, D., and Lu, C. W. (2016). Effect of Magnesium as Substitute Material in Enzyme-Mediated Calcite Precipitation for Soil-Improvement Technique. Front. Bioeng. Biotechnol. 4, 37–38. doi:10.3389/fbioe.2016.00037
Qian, X., Fang, C., Huang, M., and Achal, V. (2017). Characterization of Fungal-Mediated Carbonate Precipitation in the Biomineralization of Chromate and lead from an Aqueous Solution and Soil. J. Clean. Prod. 164 (15), 198–208. doi:10.1016/j.jclepro.2017.06.195
Rahman, M. M., Hora, R. N., Ahenkorah, I., Beecham, S., Karim, M. R., and Iqbal, A. (2020). State-of-the-art Review of Microbial-Induced Calcite Precipitation and its Sustainability in Engineering Applications. Sustainability 12 (6281), su12156281. doi:10.3390/su12156281
Shashank, B. S., Sharma, S., Sowmya, S., Latha, R. A., Meenu, P. S., and Singh, D. N. (2016). State-of-the-art on Geotechnical Engineering Perspective on Bio-Mediated Processes. Environ. Earth Sci. 75 (3), 270. doi:10.1007/s12665-015-5071-6
Shaw, W. H. R., and Raval, D. N. (1961). The Inhibition of Urease by Metal Ions at pH 8.9. J. Am. Chem. Soc. 83 (15), 3184–3187. doi:10.1021/ja01476a004
Sun, X., Miao, L., Wang, H., Yuan, J., and Fan, G. (2021). Enhanced Rainfall Erosion Durability of Enzymatically Induced Carbonate Precipitation for Dust Control. Sci. Total Environ. 791, 148369. doi:10.1016/j.scitotenv.2021.148369
Van Paassen, L. A. (2011). Bio-mediated Ground Improvement: From Laboratory experiment to Pilot Applications. Dallas, Texas, United States: ASCE, 4099–4108.
Vítková, M., Ettler, V., Sebek, O., Mihaljeviˇc, M., Grygar, T., and Rohovec, J. (2009). The pH-dependent Leaching of Inorganic Contaminants from Secondary lead Smelter Fly Ash. J. Hazard. Mater. 167, 427–433. doi:10.1016/j.jhazmat.2008.12.136
Wang, L., Cheng, W.-C., and Xue, Z.-F. (2022). The Effect of Calcium Source on Pb and Cu Remediation Using Enzyme-Induced Carbonate Precipitation. Front. Bioeng. Biotechnol. 10, 849631. doi:10.3389/fbioe.2022.849631
Wang, L., Cheng, W. C., and Xue, Z. F. (2022). Investigating microscale structural characteristics and resultant macroscale mechanical properties of loess exposed to alkaline and saline environments. B Eng. Geol environ. 81, 146. doi:10.1007/s10064-022-02640-z
Wei, W., Shao, Z. S., Qiao, R. J., Chen, W. W., Zhang, P. J., and Cheng, J. X. (2021). Workability and Mechanical Properties of Microwave Heating for Recovering High Quality Aggregate From Concrete. Construction and Building Materials 276, 122237. doi:10.1016/j.conbuildmat.2020.122237
Wu, M., Hu, X., Zhang, Q., Zhao, Y., Sun, J., Cheng, W., et al. (2020). Preparation and Performance Evaluation of Environment-Friendly Biological Dust Suppressant. J. Clean. Prod. 273, 123162. doi:10.1016/j.jclepro.2020.123162
Xue, Z.-F., Cheng, W.-C., Wang, L., and Hu, W. (2022). Effects of Bacterial Inoculation and Calcium Source on Microbial-Induced Carbonate Precipitation for lead Remediation. J. Hazard. Mater. 426, 128090. doi:10.1016/j.jhazmat.2021.128090
Xue, Z. F., Cheng, W. C., Wang, L., and Song, G. Y. (2021). Improvement of the Shearing Behaviour of Loess Using Recycled Straw Fiber Reinforcement. KSCE J. Civ. Eng. 25 9, 3319–3335. doi:10.1007/s12205-021-2263-3
Yang, Y. Y., Wu, H. L., and Du, Y. J. (2014). Strength and Leaching Characteristics of Heavy Metal Contaminated Soils Solidified by Cement. J. Residuals Sci. Techn. 11 (3), 71–98.
Yuan, H., Ren, G., Liu, K., Zheng, W., and Zhao, Z. (2020). Experimental Study of EICP Combined with Organic Materials for silt Improvement in the Yellow River Flood Area. Appl. Sci. 10, 7678. doi:10.3390/app10217678
Yuan, Y., Shao, Z. S., Qiao, R. J., Fei, X. S., Cheng, J. X., and Wei, W. (2021). Fracture Behavior of Concrete Coarse Aggregates Under Microwave Irradiation Influenced by Mineral Components. Construction and Building Materials 286, 122944. doi:10.1016/j.conbuildmat.2021.122944
Keywords: urease enzyme, carbonate precipitation, heavy metal, urease concentration, calcium source
Citation: Wang L, Cheng W-C, Xue Z-F and Hu W (2022) Effects of the Urease Concentration and Calcium Source on Enzyme-Induced Carbonate Precipitation for Lead Remediation. Front. Chem. 10:892090. doi: 10.3389/fchem.2022.892090
Received: 08 March 2022; Accepted: 25 March 2022;
Published: 27 April 2022.
Edited by:
Florent Allais, AgroParisTech Institut des Sciences et Industries du Vivant et de L’environnement, FranceReviewed by:
Isaac Ahenkorah, University of South Australia, AustraliaWei Li, Nanjing University, China
Copyright © 2022 Wang, Cheng, Xue and Hu. This is an open-access article distributed under the terms of the Creative Commons Attribution License (CC BY). The use, distribution or reproduction in other forums is permitted, provided the original author(s) and the copyright owner(s) are credited and that the original publication in this journal is cited, in accordance with accepted academic practice. No use, distribution or reproduction is permitted which does not comply with these terms.
*Correspondence: Wen-Chieh Cheng, dy1jLmNoZW5nQHhhdWF0LmVkdS5jbg==