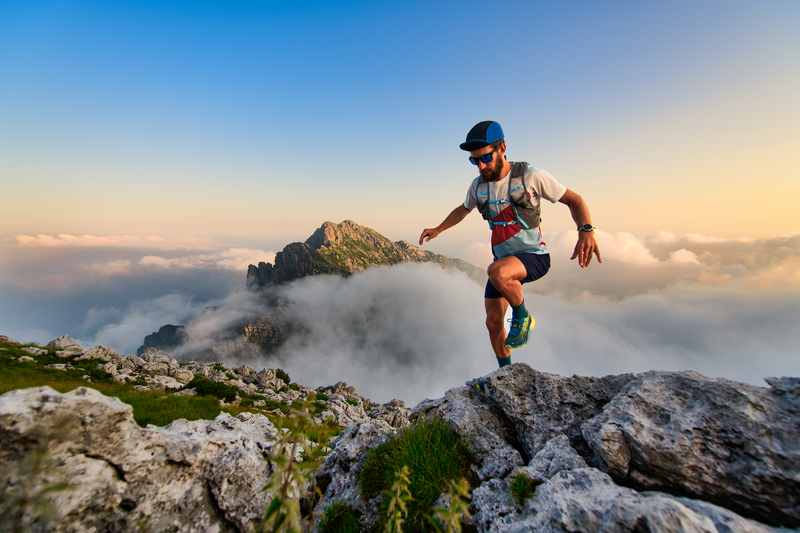
95% of researchers rate our articles as excellent or good
Learn more about the work of our research integrity team to safeguard the quality of each article we publish.
Find out more
ORIGINAL RESEARCH article
Front. Chem. , 03 May 2022
Sec. Supramolecular Chemistry
Volume 10 - 2022 | https://doi.org/10.3389/fchem.2022.885939
This article is part of the Research Topic Advances in Mechanically Bonded Molecules View all 9 articles
Ring-over-ring slippage and ring-through-ring penetration are important processes in the construction of ring-in-ring multiple interlocked architectures. We have successfully observed “ring-over-ring deslipping” on the rotaxane axle by exploiting the dynamic covalent nature of imine bonds in imine-bridged heterorotaxanes R1 and R2 with two macrocycles of different ring sizes on the axle. When the imine bridges of R1 were cleaved, a hydrolyzed hetero[4]rotaxane [4]R1′ was formed as an intermediate under dynamic equilibrium, and the larger 38-membered macrocycle M was deslipped over the 24-membered ring (24C8 or DB24C8) to dissociate into a [3]rotaxane [3]R3 and a macrocycle M. The time dependent NMR measurement and the determined thermodynamic parameters revealed that the rate-limiting step of the deslipping process was attributed to steric hindrance between two rings and reduced mobility of M due to proximity to the crown ether, which was bound to the anilinium on the axle molecule.
Cyclic molecules are chemical species that have attracted the interest of chemists due to their topology, restricted flexibility, and internal cavity (Forgan et al., 2011). The interaction and relative mobility, such as threading, slipping, and shuttling motion between ring and linear molecules, have been extensively studied by rotaxane chemistry (Amabilino and Stoddart, 1995; Kay et al., 2007; Xue et al., 2015). On the other hand, the interactions and motions between cyclic molecules have been investigated with regard to stacking (Grave and Schlüter, 2002) and “ring-in-ring” assembly (Cantrill et al., 2005; Kawase et al., 2007; Klosterman et al., 2016), catenation and pirouetting motion (Evans and Beer, 2014), while “ring-over-ring” slippage has been rarely investigated (Schweez et al., 2016; Zhu et al., 2018) (Figure 1). For example, the rings of main-chain [n]rotaxanes, which have multiple rings on the axle (Harada et al., 2009; Fang et al., 2010), usually do not slip past each other, but translate together. This limitation in mobility is the cause of sequence isomers in hetero[n]rotaxanes (Fuller et al., 2010; Neal and Goldup, 2014; Wang et al., 2018) and has been applied to the development of new rotaxane construction methods, such as cascade stoppering based on integrative threading of rings (Jiang et al., 2008; Rao et al., 2017). On the other hand, the first “ring-through-ring” rotaxane was reported by Loeb, where it was revealed that a [24]crown-8 ring (24C8) could pass through a [42]crown-8 ring but not a [30]crown-8 one on the axle (Zhu et al., 2018). This “ring-through-ring” slipping has opened a new gate to extend the mobility range between components inherent in rotaxane.
We have developed imine-bridged rotaxanes in which the mobility of the rings can be switched reversibly by turning on/off the imine bridges between the aniline ring and the axle of the imine-bridged rotaxane (Kawai et al., 2006; Umehara et al., 2008; Sugino et al., 2012). We predicted that if additional smaller rings were placed on the axle of this imine-bridged rotaxane, the dynamic covalent bond between the central aniline macrocycle and the axle would act as a gate for the shuttling motion of the smaller rings, allowing gating control (Chatterjee et al., 2006; Erbas-Cakmak et al., 2015; Borsley et al., 2021) of the position and mobility of rings (Figure 2). To use this control method, the following requirements must be achieved: 1) the incorporation of an additional small ring into the imine-bridged rotaxane by using different interactions; 2) the ability of the larger aniline macrocycle to pass over the small ring (or the small ring to pass through the larger macrocycle); and 3) the stability and reversibility of the imine bridging site in the heterorotaxane for keeping the aniline ring connected to the axle and releasing its mobility. As a part of our efforts toward this target, we have successfully observed “ring-over-ring deslipping” from the rotaxane axle, where the large macrocycle surmounts the small ring, by controlling the imine bridging of a hetero[4]rotaxane with two types of macrocycles with different ring sizes.
FIGURE 2. Gating control of ring position and mobility in hetero[n]rotaxane using a combination of imine-bridging and “ring-over-ring” slippage.
To observe this ring-over-ring slipping, we designed imine-bridged heterorotaxanes R1 and R2 as shown in Scheme 1. The key points are as follows: 1) the large aniline macrocycle (38-membered ring) is imine-bridged to the starting station to prevent it from falling off the end of the axle; 2) two crown ethers (24-membered rings) are located on the anilinium stations on both sides and are smaller than the central macrocycle; and 3) the triphenylmethyl end cap is large enough to prevent the crown ether from dethreading, but not to prevent the central macrocycle from dethreading. Therefore, when the imine bonds of this imine-bridged heterorotaxane R1 are hydrolyzed to generate hetero[4]rotaxane [4]R1′, we expected that only the large macrocycle would be deslipped over the crown ether (ring-over-ring), producing the aniline macrocycle M and the [3]rotaxane [3]R3 (Scheme 2). Here we report the synthesis of imine-bridged heterorotaxanes R and their deslipping behavior based on imine hydrolysis. Our results provide a new way to control the mobility and position of components in rotaxanes. Rotaxanes with multiple rings passing each other on a single track will open up new functionalities such as high flexibility and topological shape memory (Hart et al., 2021).
SCHEME 2. “Ring-over-ring” slippage and dethreading of macrocycle M after generation of hetero [4] rotaxanes [4]R1′ from imine-bridged heterorotaxanes R1.
1H and 13C NMR spectra were recorded on a Bruker BioSpin AVANCE DPX-400 and an AVANCE 400M (1H: 400 MHz, 13C: 100 MHz) spectrometer. IR spectra were taken on a JASCO FT/IR-4600 (ATR). HRMS analysis was performed on a JEOL JMS-S3000 SpiralTOF (MALDI-TOF). All melting points were determined on a METTLER TOLEDO MP90. Column chromatography was performed on silica gel 60 (YMC, particle size 40–63 μm). GPC purification was carried out on LC-908 with JAIGEL-1HH + 2HH columns eluted with CHCl3. Reactions were carried out under an argon atmosphere. All commercially available compounds were used without further purification unless otherwise indicated. The macrocycle M (Kawai et al., 2006), imine-bridged prerotaxane P1 (Kawai et al., 2006), and 4-(2-bromoethoxy)benzaldehyde E1 (Wang et al., 2015) were prepared by following the known procedures.
To a solution of E1 (230 mg, 1.0 mmol) and 4-triphenylmethylaniline (350 mg, 1.0 mmol) in CHCl3 (55 mL) was added MS4Å. After the reaction mixture was stirred for 68 h at room temperature, it was filtered. The crude product obtained by concentrating the filtrate was diluted with THF (30 mL) and ethanol (30 mL), and NaBH4 (10.3 mg, 0.27 mmol) and anhydrous MgSO4 (ca. 50 mg) were added to it, stirred for 22 h at room temperature, and filtered. The filtrate was diluted with chloroform and water and separated. The organic layer was washed successively with H2O and brine, dried over MgSO4, and then filtered. The crude product obtained by concentrating the filtrate was subjected to chromatography on silica gel eluted with 12% ethyl acetate/hexane to give E2 (185 mg, 34% yield, two steps) as a yellow solid.
M.p.: 102–150°C (decomp.); 1H NMR (400 MHz, CDCl3): δ/ppm 7.28 (d, J = 8.5 Hz, 2H), 7.25–7.16 (m, 15 H), 6.97 (d, J = 9.0 Hz, 2H), 6.88 (d, J = 9.0 Hz, 2H), 6.51 (d, J = 8.5 Hz, 2H), 4.28 (t, J = 6.3 Hz, 2H), 4.22 (s, 2H), 3.88 (s, 1H), 3.62 (t, J = 6.3 Hz, 2H); 13C NMR (100 MHz, CDCl3): δ/ppm 157.37, 147.29, 145.95, 135.90, 132.29, 132.01, 129.00, 127.29, 125.67, 114.91, 114.84, 111.75, 67.95, 64.21, 47.89, 29.07; IR (ATR): 3410, 3025, 2855, 1610, 1509, 1240, 1173, 819, 746, 699 cm−1; HR-MS (MALDI-TOF-MS, DCTB): Calcd. for C34H30BrNO+Na+: 570.1403, found: 570.1420.
To a solution of E2 (710 mg, 1.3 mmol) in DMF (10 mL), was added NaN3 (84 mg, 1.3 mmol). The mixture was stirred at 100°C for 2 h. After the reaction mixture was cooled to room temperature, it was diluted with diethyl ether and washed successively with H2O and brine, dried over MgSO4, and then filtered. The yellow solid obtained by concentrating the filtrate was pure E3 (642 mg, 97% yield).
M.p.: 146–154°C; 1H NMR (400 MHz, CDCl3): δ/ppm 7.29 (d, J = 8.5 Hz, 2H), 7.25–7.14 (m, 15 H), 6.98 (d, J = 9.0 Hz, 2H), 6.89 (d, J = 9.0 Hz, 2H), 6.52 (d, J = 8.5 Hz, 2H), 4.22 (s, 2H), 4.14 (t, J = 6.3 Hz, 2H), 3.88 (s, 1H), 3.58 (t, J = 6.3 Hz, 2H); 13C NMR (100 MHz, CDCl3): δ/ppm 157.1, 147.29, 145.96, 135.88, 132.20, 132.00, 131.13, 128.97, 127.29, 125.67, 114.73, 111.74, 67.01, 64.21, 50.15, 47.90; IR (ATR): 3384, 3053, 3029, 2931, 2843, 2088, 1610, 1510, 1240, 823, 746, 699, 629 cm−1; HR-MS (MALDI-TOF-MS, DCTB): Calcd. for C34H30N4O+Na+: 510.2414, found: 510.2435.
To a solution of E3 (300 mg, 0.59 mmol) in methanol (9.0 mL) was added conc. HCl (0.1 mL). After the reaction mixture was stirred for 1 h at room temperature, it was filtered. The filtered ammonium salt was dissolved in a small amount of methanol to make a saturated solution, and satd. NH4PF6aq. was added until no more solid precipitated. The yellow solid obtained by filtering the suspension was pure E4 (360 mg, 93% yield).
M.p.: 128–136°C; 1H NMR (400 MHz, CDCl3): δ/ppm 7.31–7.08 (m, 17H), 7.02 (d, J = 8.6 Hz, 2H), 6.97 (d, J = 8.6 Hz, 2H), 6.80 (d, J = 8.6 Hz, 2H), 4.54 (s, 2H), 4.10 (t, J = 5.0 Hz, 2H), 3.59 (t, J = 5.0 Hz, 2H), 1.55 (br.s, 2H); 13C NMR (400 MHz, CDCl3): δ/ppm 159.28, 159.20, 145.92, 132.55, 132.46, 130.93, 127.70, 126.28, 122.68, 114.69, 97.09, 66.88, 64.74, 50.06; IR (ATR): 3162, 2935, 2117, 1611, 1516, 1255, 1180, 820, 748, 702, 633, 556 cm−1.
To a solution of P1 (123 mg, 80 μmol) in dry DMF (8 mL) and dry THF (12 mL) at room temperature, was added TBAF (1.0 M solution in THF, 190 μL, 190 μmol) under an argon atmosphere. After the mixture was stirred for 10 min, Cs2CO3 (130 mg, 0.4 mmol) and 3-bromopropyne (57 μL, 0.77 mmol) were added. The mixture was stirred for 16 h at room temperature. The reaction mixture was diluted with CH2Cl2 and washed successively with satd. NH4Claq., H2O, and brine, dried over MgSO4, and then filtered. The crude product obtained by concentrating the filtrate was purified by GPC separation to give P2 (56 mg, 51%) as a white solid.
M.p.: 201–250°C (decomp); 1H NMR (400 MHz, CDCl3): δ/ppm 7.47 (d, J = 8.5 Hz, 4H), 7.42 (d, J = 8.5 Hz, 4H), 7.32–6.52 (m, 30H), 4.73 (s, 4H), 4.22–3.96 (m, 8H), 3.24 (d, J = 15 Hz, 4H), 3.10 (d, J = 15 Hz, 4H), 2.54 (t, J = 2.1 Hz, 4H), 1.92–1.76 (m, 8H), 1.57–1.48 (m, 8H), 1.26 (s, 2H); 13C NMR (100 MHz, CDCl3): δ/ppm 169.34, 157.04, 148.60, 143.02, 139.24, 139.06, 134.11, 133.36, 132.35, 131.01, 130.80, 129.03, 128.58, 128.21, 128.07, 127.61, 126.82, 125.29, 123.88, 120.62, 115.22, 114.82, 114.54, 78.51, 75.61, 68.25, 56.55, 55.88, 40.45, 25.87, 25.75, 25.60; IR (ATR): 3250, 3030, 3007, 2925, 2858, 1725, 1649, 1606, 1510, 1496, 1235, 1174, 1017, 1001, 821, 795, 751 cm−1; HR-MS (MALDI-TOF-MS, DHB): Calcd. for C92H80N2O6+H+: 1309.6089, found: 1309.6142.
Pseudorotaxane with a [24]C8 was prepared by adding [24]C8 (21 mg, 57 μmol) to E4 (19 mg, 29 μmol) in dry CH2Cl2 (1.2 mL) and stirring at room temperature for 10 min. Under an argon atmosphere P2 (15 mg, 11 μmol) and [Cu(MeCN)4]PF6 (10 mg, 29 μmol) were added to the solution. The mixture was stirred for 6 h at room temperature in the dark and concentrated. The crude product was washed with methanol to give R1 (28 mg, 73%) as a pale brown solid.
M.p.: 162–170°C (decomp); 1H NMR (400 MHz, CD3CN/CDCl3 = 1:1): δ/ppm 8.99 (br.s, 4H), 7.96 (br.s, 2H), 7.48–6.45 (m, 86H), 5.22 (s, 4H), 5.07–4.99 (m, 4H), 4.80 (t, J = 4.6 Hz, 4H), 4.45 (t, J = 4.6 Hz, 4H), 4.17–4.00 (m, 8H), 3.49–3.20 (m, 64H), 3.12 (d, J = 15.0 Hz, 4H), 1.91–1.76 (m, 8H), 1.61–1.45 (m, 8H); 13C NMR (100 MHz, CD3CN/CDCl3 = 1:1): δ/ppm 170.14, 159.28, 158.52, 157.67, 149.44, 148.86, 146.71, 143.38, 139.75, 139.26, 136.16, 133.84, 133.79, 133.67, 132.58, 132.51, 131.44, 132.29, 131.37, 131.17, 129.02, 128.46, 128.39, 128.20, 127.91, 127.09, 126.76, 125.96, 124.45, 122.64, 120.95, 115.66, 115.24, 80.65, 70.86, 70.72, 68.57, 66.97, 65.44, 62.18, 57.64, 51.09, 50.16, 40.94, 29.35, 26.18; IR (ATR): 2870, 2357, 1607, 1511, 1496, 1457, 1350, 1238, 1177, 1091, 1033, 955, 837, 749, 702, 556 cm−1; HR-MS (MALDI-TOF-MS, DHB): Calcd. for C192H205N10O24++H2O: 3053.5307, found: 3053.539.
Pseudorotaxane with a DB[24]C8 was prepared by adding DB[24]C8 (13 mg, 29 μmol) to E4 (19 mg, 29 μmol) in dry CH2Cl2 (0.6 mL) and stirring at room temperature for 10 min. Under an argon atmosphere P2 (7.5 mg, 6 μmol) and [Cu(MeCN)4]PF6 (5.0 mg, 15 μmol) were added to the solution. The mixture was stirred for 6 h at room temperature in the dark and concentrated. The crude product was washed with hot toluene to give R2 (6.5 mg, 36%) as a pale brown solid.
M.p.: 170–195°C (decomp); 1H NMR (400 MHz, CD3CN/CDCl3 = 1:1): δ/ppm 8.95 (br.s, 4H), 7.96 (br.s, 2H), 7.52–6.60 (m, 94H), 5.24 (s, 4H), 5.19–5.12 (m, 4H), 4.73 (t, J = 4.5 Hz, 4H), 4.24 (t, J = 4.5 Hz, 4H), 4.08 (m, 9H), 3.98–3.93 (m, 18H), 3.64 (s, 16H), 3.32–3.16 (m, 18H), 3.13 (d, J = 15.0 Hz, 4H) 1.90–1.73 (m, 8H), 1.60–1.41 (m, 8H); 13C NMR (100 MHz, CD3CN/CDCl3 = 1:1): δ/ppm 158.94, 149.12, 147.59, 147.57, 146.64, 133.29, 132.28, 131.37, 131.29, 131.22, 131.17, 128.65, 128.54, 128.51, 128.47, 128.31, 127.55, 126.72, 125.55, 125.52, 122.03, 121.75, 121.13, 115.73, 115.69, 115.47, 115.26, 114.99, 112.49, 70.97, 70.39, 68.32, 66.75, 65.25, 51.37, 50.18, 50.16, 38.90, 28.97; IR (ATR): 2936, 2363, 1506, 1247, 1123, 836, 744, 555 cm−1; HR-MS (MALDI-TOF-MS, DHB): Calcd. for C208H205N10O24+: 3226.5113, found: 3226.5068.
To a solution of imine-bridged heterorotaxanes R1 (0.50 mg, 0.30 μmol) or R2 (0.25 mg, 0.15 μmol) in water-saturated CDCl3 and CD3CN (v/v 1:1, 0.5 mL) in an NMR tube was added 10% TFA in CDCl3 (100 eq.). The NMR tube was kept at constant temperature in a thermostatic bath at 30, 40, or 50°C. The time-courses of hydrolysis and attainment of equilibrium to give macrocycle M and [3]R3 or [3]R4 were monitored by 1H NMR spectroscopy.
[3]rotaxane [3]R3: 1H NMR (400 MHz, CD3CN/CDCl3 = 1:1): δ/ppm 9.48 (s, 2H), 9.00 (br.s, 4H), 8.02 (s, 2H), 7.66–6.54 (m, 66H), 5.22 (s, 4H), 5.25 (s, 4H) 5.03 (br.s, 4H), 4.83 (t, J = 5.0 Hz, 4H), 4.47 (t, J = 5.0 Hz, 4H), 3.81 (d, J = 15 Hz, 4H), 3.34–3.24 (m, 68H).
[3]rotaxane [3]R4: 1H NMR (400 MHz, CD3CN/CDCl3 = 1:1): δ/ppm 9.47 (s, 2H), 8.97 (br.s, 4H), 7.94 (s, 2H), 7.66–6.54 (m, 82H), 5.22 (s, 4H), 5.21–5.14 (m, 4H), 4.74 (t, J = 5.0 Hz, 4H), 4.25 (t, J = 5.0 Hz, 4H), 4.14–3.73 (m, 18H), 3.80 (d, J = 15 Hz, 4H), 3.66 (s, 16H), 3.38–3.18 (m, 22H).
The synthesis of imine-bridged heterorotaxanes R1 and R2 with one 38-membered aniline macrocycle and two [24]crown-8 ([24]C8) or dibenzo[24]crown-8 (DB24C8) rings on the axle is shown in Scheme 3. Pseudorotaxane E4•[24]C8 was prepared by threading [24]C8 added to a triphenylmethyl-type end cap E4 containing an anilinium station for the crown ether and terminated with an azide group in CH2Cl2 solution. The desired imine-bridged heterorotaxane [3]R1 was obtained by the CuAAC reaction in 73% yield by adding 0.5 equivalents of propargyl-terminated imine-bridged prerotaxane P2 and Cu (MeCN)4PF6 to the prepared solution of pseudorotaxane E4•DB[24]C8. Imine-bridged heterorotaxane R2 with DB[24]C8 was prepared by the same method in 36% yield.
The rotaxane moiety with [24]C8 was evidenced by the appearance of benzylanilinium CH2 and +NH2 moieties at large downfield shifted positions of 5.0 and 9.0 ppm, which were similar to the values observed for other rotaxanes with [24]C8 (Kimura et al., 2017) (Figure 3). In addition, the threaded [24]C8 part appeared as a large singlet at 3.3 ppm, which overlapped with the signal of the central imine-bridged station. The characteristic feature of the imine-bridged station of R1 is the methylene moiety of the five-membered ring appearing as two sets of doublets at around 3.2 ppm, which can be compared with the sets at 3.2 and 3.8 ppm of the bisaldehyde station resulting from the hydrolysis of the imine bonds (Kawai et al., 2006). Furthermore, the signals of the OCH2CH2CH2- moiety of the aniline macrocycle appear at 4.1, 1.8, and 1.5 ppm, which are close to those at 4.1, 1.8, and 1.6 ppm of the macrocycle M itself (Figure 4D). These peaks can be used as one of the probes to study the deslipping of the aniline macrocycle M. These observations confirm that the large aniline macrocycle is located on the imine station and the two [24]C8s are located on the benzyl anilinium stations at both ends, and therefore the heterorotaxane structure is stable without any dethreading of either ring.
FIGURE 3. 1H NMR spectra (400 MHz, at 298 K) of (A) pseudorotaxane E4•[24]C8 and free [24]C8 in CDCl3, (B) imine-bridged prerotaxane P2 in CDCl3, and (C) imine-bridged heterorotaxane R1 in CDCl3/CD3CN. *: impurity or satellite from solvent peak.
FIGURE 4. 1H NMR spectra (400 MHz, CDCl3/CD3CN at 303 K) of (A) imine-bridged heterorotaxane R1, (B) hetero[4]rotaxane [4]R1′ and [3]rotaxane [3]R3 generated upon the addition of TFA to a solution of R1, (C) [3]rotaxane [3]R3 and macrocycle M after 18 h and (D) time-course of hetero[4]rotaxane [4]R1′ and dethreaded macrocycle M from imine-bridged heterorotaxane R1 upon imine hydrolysis. *: impurity or satellite from solvent peak.
Next, we investigated whether the aniline macrocycle by hydrolyzing imine bonds of R1 could be deslipped over the small ring and end cap at either end to dissociate into hetero[4]rotaxane [4]R1′ and macrocycle M (Scheme 3). It was observed that when TFA was added to a CDCl3/CD3CN 1:1 (v/v) solution of imine-bridged heterorotaxane R1, several chemical species immediately appeared, some of which increased with time (Figure 4). The hydrolysis of the imine bonds was confirmed by the two aldehyde signals appearing at around 9.5 ppm and the doublet at 3.8 ppm of the five-membered ring proton at the central station, which were also observed to increase with time, and the signals of imine-bridged R1 almost disappeared after 24 h. The rotaxane structure with [24]C8 remained unchanged during these processes, which was confirmed by the fact that the signals at these sites remained constant in position and intensity. Most importantly, the increase in the signal at 1.6 ppm, which is characteristic of macrocycle M itself, demonstrates the ‘ring-over-ring’ deslipping of the aniline macrocycle M over [24]C8, accompanying the hydrolysis of the imine bonds.
An interesting finding was the observation of hydrolyzed hetero[4]rotaxane [4]R1′ as an intermediate in the process of deslipping, which provides important insight into the mechanism (rate-limiting step) of deslipping. Interestingly, the signal of the macrocycle CH2- moiety in the hydrolyzed hetero[4]rotaxane [4]R1′ was observed at 1.7 ppm, which remained almost constant in intensity as a steady state and finally disappeared. Also in the aldehyde station of the axle molecule, the signal of the intermediate appeared as a steady state at 9.4 ppm and finally disappeared with the consumption of the imine form R1. This result implies that the hydrolysis of the imine bond of R1 proceeds fast, resulting in a dynamic equilibrium state with the hetero[4]rotaxane [4]R1′, from which the deslipping of macrocycle M is a much slower, rate-limiting step. This is also consistent with previous results where an imine-bridged rotaxane immediately produces hydrolyzed [2]rotaxane upon addition of acid, resulting in a dynamic equilibrium (Kawai et al., 2006). It has also been shown that the presence of an additional hydrogen bonding station biases the equilibrium ratio toward the hydrolyzed rotaxane (the ratio of hydrolyzed rotaxane in CDCl3 at room temperature is 9% without a hydrogen bonding station and 95% with a TEG station) (Umehara et al., 2008). During the hydrolysis of R1 in this study, the ratio of imine-bridged heterorotaxane R1 to the intermediate hydrolyzed hetero[4]rotaxane [4]R1′ was about 2:1 (Figure 5), suggesting that the aniline macrocycle weakly interacts with the crown ether or triazole moiety in the hydrolyzed hetero[4]rotaxane [4]R1′. These results suggest that the rate-limiting step of deslipping is not the hydrolysis of the imine bonds of R1, but the deslipping step of the aniline macrocycle from the hydrolyzed hetero[4]rotaxane [4]R1′.
FIGURE 5. (A) X-ray structure of macrocycle M (CCDC 2158687). (B) Front view and (C) top view of the molecular model of macrocycle M and DB24C8 on the axle moiety of [4]R2′ (terminated with triazole). The molecular model (neither the most stable structure nor the transition structure) was prepared by using the SPARTAN ’14 to estimate the size of the rings.
Furthermore, the deslipping seems to be irreversible under these conditions, since the starting material R1 was almost completely consumed and the imine form R1 was not regenerated from the resulting [3]rotaxane [3]R3 and macrocycle M. A comparable deslipping study was also performed using imine-bridged heterorotaxane R2 containing the bulkier DB[24]C8 (Supplementary Figure S13, See Supplementary Material). The deslipping proceeded, although it required a longer time (τ1/2 = 27.2 h) than that of R1 (τ1/2 = 9.2 h), confirming that the aniline macrocycle was large enough to overcome DB[24]C8 (Figure 5; Supplementary Figure S14).
In order to determine the thermodynamic parameters of this deslipping process, we monitored the deslipping reaction at 303, 313, and 323 K (Figure 6; Supplementary Figure S15) and performed a pseudo-first order reaction kinetics analysis as the dissociation from the [4]rotaxane components (sum of imine R1 and hetero[4]rotaxane [4]R1′) to [3]rotaxane [3]R3 and macrocycle M. The results are shown in Table 1. The thermodynamic parameters of R1 with [24]C8 at 303 K were determined to be a rate constant k of 0.94 × 10−3 s−1 and half-life of 9.2 h (Supplementary Figure S16). The ΔH‡, ΔS‡, and ΔG303‡ values in the transition state are 12.0 kcal mol−1, -33.1 calmol−1K−1, and 22.0 kcal mol−1, respectively, indicating that the aniline macrocycle is sterically hampered by the crown ether moiety and its mobility is severely limited in the transition state. In R2 with DB[24]C8, the ΔG303‡ is 22.3 kcal mol−1 and the half-life is 27.2 h, reflecting the increased steric hindrance compared to R1. Comparison of these parameters for the deslipping of the 38-membered macrocycle M from [4]R1′ with those of the 42-membered ring B42C8 of Loeb’s system to pass over the same 24C8 ring (Zhu et al., 2018) would provide important insight into the understanding of the dynamics of mechanically bonded molecules. Loeb et al. discussed the extra energy cost required for the “ring-through-ring” slipping based on the difference in shuttling parameters between [2]rotaxane and hetero[3]rotaxane (ΔH‡ = +1.6 kcal mol−1, ΔS‡ = −4.8 cal mol−1K−1). The higher enthalpy and entropy costs for [4]R1′ (ΔH‡ = 12.0 kcal mol−1 and ΔS‡ = −33.1 cal mol−1K−1) compared to Loeb’s rotaxane is presumably due to the larger steric hindrance due to the smaller inner cavity of the 38-membered macrocycle M with inner amino groups (Figure 6), the flexibility based on two hexamethylene linkers, and the weaker interaction with the rotaxane axle.
FIGURE 6. Time-courses of ratios of imine-bridged heterorotaxane R1, hetero[4]rotaxane [4]R1′ and dethreaded macrocycle M upon imine hydrolysis of R1 at 323 K.
TABLE 1. Thermodynamic parameters for the “ring-over-ring” dethreading macrocycle M from hetero[4]rotaxanes R1 and R2 to give [3]rotaxanes [3]R3 and [3]R4 in CDCl3/CD3CN.
In summary, we constructed imine-bridged heterorotaxanes R1 and R2 with two different sized rings to investigate the rings passing each other on the rotaxane axle (single track). When the imine bridges of R1 were cleaved, a hydrolyzed hetero[4]rotaxane [4]R1′ was generated as an intermediate under dynamic equilibrium, and finally the large aniline macrocycle was deslipped over the crown ether to dissociate into a [3]rotaxane [3]R3 and a macrocycle M. The determined thermodynamic parameters revealed that the rate-limiting step of the deslipping process was attributed to steric hindrance between two rings and reduced mobility due to the proximity of M to the crown ether, which was bound to the anilinium on the axle molecule. For the future construction and operation of molecular machines, it is desirable to control the motion and state of multiple different components independently. The “ring-through-ring” mobility on the axle adds a new dimension to the motion control of rotaxanes as well as challenges for how to deal with interactions and steric hindrance between components. We are currently working on the development of a ratcheting function by combining ring-through-ring slippage with gating control of imine bridges.
The original contributions presented in the study are included in the article/Supplementary Material, further inquiries can be directed to the corresponding author.
HK conceived the project, designed the experiments and wrote the manuscript. SH synthesized, characterized the compounds, and analyzed the data. KO helped with experiments and co-wrote the manuscript. All authors contributed to the discussion of the results for the manuscript.
This work was supported by the JSPS KAKENHI (Grant Numbers JP24685008 and JP20K05478).
The authors declare that the research was conducted in the absence of any commercial or financial relationships that could be construed as a potential conflict of interest.
All claims expressed in this article are solely those of the authors and do not necessarily represent those of their affiliated organizations, or those of the publisher, the editors, and the reviewers. Any product that may be evaluated in this article, or claim that may be made by its manufacturer, is not guaranteed or endorsed by the publisher.
The authors thank the Cooperative Research Program of “NJRC Mater. & Dev.”
The Supplementary Material for this article can be found online at: https://www.frontiersin.org/articles/10.3389/fchem.2022.885939/full#supplementary-material
Amabilino, D. B., and Stoddart, J. F. (1995). Interlocked and Intertwined Structures and Superstructures. Chem. Rev. 95, 2725–2828. doi:10.1021/cr00040a005
Borsley, S., Leigh, D. A., and Roberts, B. M. W. (2021). A Doubly Kinetically-Gated Information Ratchet Autonomously Driven by Carbodiimide Hydration. J. Am. Chem. Soc. 143, 4414–4420. doi:10.1021/jacs.1c01172
Cantrill, S. J., Chichak, K. S., Peters, A. J., and Stoddart, J. F. (2005). Nanoscale Borromean Rings. Acc. Chem. Res. 38, 1–9. doi:10.1021/ar040226x
Chatterjee, M. N., Kay, E. R., and Leigh, D. A. (2006). Beyond Switches: Ratcheting a Particle Energetically Uphill with a Compartmentalized Molecular Machine. J. Am. Chem. Soc. 128, 4058–4073. doi:10.1021/ja057664z
Erbas-Cakmak, S., Leigh, D. A., McTernan, C. T., and Nussbaumer, A. L. (2015). Artificial Molecular Machines. Chem. Rev. 115, 10081–10206. doi:10.1021/acs.chemrev.5b00146
Evans, N. H., and Beer, P. D. (2014). Progress in the Synthesis and Exploitation of Catenanes since the Millennium. Chem. Soc. Rev. 43, 4658–4683. doi:10.1039/c4cs00029c
Fang, L., Olson, M. A., Benítez, D., Tkatchouk, E., Goddard III, W. A., and Stoddart, J. F. (2010). Mechanically Bonded Macromolecules. Chem. Soc. Rev. 39, 17–29. doi:10.1039/b917901a
Forgan, R. S., Sauvage, J.-P., and Stoddart, J. F. (2011). Chemical Topology: Complex Molecular Knots, Links, and Entanglements. Chem. Rev. 111, 5434–5464. doi:10.1021/cr200034u
Fuller, A.-M. L., Leigh, D. A., and Lusby, P. J. (2010). Sequence Isomerism in [3]Rotaxanes. J. Am. Chem. Soc. 132, 4954–4959. doi:10.1021/ja1006838
Grave, C., and Schlüter, A. D. (2002). Shape-Persistent, Nano-Sized Macrocycles. Eur. J. Org. Chem. 3075–3098. doi:10.1002/1099-0690(200209)2002:18<3075::aid-ejoc3075>3.0.co;2-3
Harada, A., Hashidzume, A., Yamaguchi, H., and Takashima, Y. (2009). Polymeric Rotaxanes. Chem. Rev. 109, 5974–6023. doi:10.1021/cr9000622
Hart, L. F., Hertzog, J. E., Rauscher, P. M., Rawe, B. W., Tranquilli, M. M., and Rowan, S. J. (2021). Material Properties and Applications of Mechanically Interlocked Polymers. Nat. Rev. Mater. 6, 508–530. doi:10.1038/s41578-021-00278-z
Jiang, W., Winkler, H. D. F., and Schalley, C. A. (2008). Integrative Self-Sorting: Construction of a Cascade-Stoppered Hetero[3]rotaxane. J. Am. Chem. Soc. 130, 13852–13853. doi:10.1021/ja806009d
Kawai, H., Umehara, T., Fujiwara, K., Tsuji, T., and Suzuki, T. (2006). Dynamic Covalently Bonded Rotaxanes Cross-Linked by Imine Bonds between the Axle and Ring: Inverse Temperature Dependence of Subunit Mobility. Angew. Chem. Int. Ed. 45, 4281–4286. doi:10.1002/anie.200600750
Kawase, T., Nishiyama, Y., Nakamura, T., Ebi, T., Matsumoto, K., Kurata, H., et al. (2007). Cyclic [5]Paraphenyleneacetylene: Synthesis, Properties, and Formation of a Ring-In-Ring Complex Showing a Considerably Large Association Constant and Entropy Effect. Angew. Chem. Int. Ed. 46, 1086–1088. doi:10.1002/anie.200603707
Kay, E. R., Leigh, D. A., and Zerbetto, F. (2007). Synthetic Molecular Motors and Mechanical Machines. Angew. Chem. Int. Ed. 46, 72–191. doi:10.1002/anie.200504313
Kimura, M., Mizuno, T., Ueda, M., Miyagawa, S., Kawasaki, T., and Tokunaga, Y. (2017). Four-State Molecular Shuttling of [2]Rotaxanes in Response to Acid/Base and Alkali-Metal Cation Stimuli. Chem. Asian J. 12, 1381–1390. doi:10.1002/asia.201700493
Klosterman, J. K., Veliks, J., Frantz, D. K., Yasui, Y., Loepfe, M., Zysman-Colman, E., et al. (2016). Conformations of Large Macrocycles and Ring-In-Ring Complexes. Org. Chem. Front. 3, 661–666. doi:10.1039/c6qo00024j
Neal, E. A., and Goldup, S. M. (2014). Chemical Consequences of Mechanical Bonding in Catenanes and Rotaxanes: Isomerism, Modification, Catalysis and Molecular Machines for Synthesis. Chem. Commun. 50, 5128–5142. doi:10.1039/c3cc47842d
Rao, S.-J., Zhang, Q., Mei, J., Ye, X.-H., Gao, C., Wang, Q.-C., et al. (2017). One-pot Synthesis of Hetero[6]rotaxane Bearing Three Different Kinds of Macrocycle through a Self-Sorting Process. Chem. Sci. 8, 6777–6783. doi:10.1039/C7SC03232C
Schweez, C., Shushkov, P., Grimme, S., and Höger, S. (2016). Synthesis and Dynamics of Nanosized Phenylene-Ethynylene-Butadiynylene Rotaxanes and the Role of Shape Persistence. Angew. Chem. Int. Ed. 55, 3328–3333. doi:10.1002/anie.201509702
Sugino, H., Kawai, H., Umehara, T., Fujiwara, K., and Suzuki, T. (2012). Effects of Axle-Core, Macrocycle, and Side-Station Structures on the Threading and Hydrolysis Processes of Imine-Bridged Rotaxanes. Chem. Eur. J. 18, 13722–13732. doi:10.1002/chem.201200837
Umehara, T., Kawai, H., Fujiwara, K., and Suzuki, T. (2008). Entropy- and Hydrolytic-Driven Positional Switching of Macrocycle between Imine- and Hydrogen-Bonding Stations in Rotaxane-Based Molecular Shuttles. J. Am. Chem. Soc. 130, 13981–13988. doi:10.1021/ja804888b
Wang, X.-Q., Li, W.-J., Wang, W., and Yang, H.-B. (2018). Heterorotaxanes. Chem. Commun. 54, 13303–13318. doi:10.1039/c8cc07283c
Wang, Z., Xie, Y., Xu, K., Zhao, J., and Glusac, K. D. (2015). Diiodobodipy-styrylbodipy Dyads: Preparation and Study of the Intersystem Crossing and Fluorescence Resonance Energy Transfer. J. Phys. Chem. A. 119, 6791–6806. doi:10.1021/acs.jpca.5b03463
Xue, M., Yang, Y., Chi, X., Yan, X., and Huang, F. (2015). Development of Pseudorotaxanes and Rotaxanes: From Synthesis to Stimuli-Responsive Motions to Applications. Chem. Rev. 115, 7398–7501. doi:10.1021/cr5005869
Keywords: dynamic covalent bond, imine, macrocycle, molecular shuttle, rotaxane, supramolecular chemistry
Citation: Hoshino S, Ono K and Kawai H (2022) Ring-Over-Ring Deslipping From Imine-Bridged Heterorotaxanes. Front. Chem. 10:885939. doi: 10.3389/fchem.2022.885939
Received: 28 February 2022; Accepted: 28 March 2022;
Published: 03 May 2022.
Edited by:
Keiji Hirose, Osaka University, JapanReviewed by:
Steve Goldup, University of Southampton, United KingdomCopyright © 2022 Hoshino, Ono and Kawai. This is an open-access article distributed under the terms of the Creative Commons Attribution License (CC BY). The use, distribution or reproduction in other forums is permitted, provided the original author(s) and the copyright owner(s) are credited and that the original publication in this journal is cited, in accordance with accepted academic practice. No use, distribution or reproduction is permitted which does not comply with these terms.
*Correspondence: Hidetoshi Kawai, a2F3YWloQHJzLnR1cy5hYy5qcA==
Disclaimer: All claims expressed in this article are solely those of the authors and do not necessarily represent those of their affiliated organizations, or those of the publisher, the editors and the reviewers. Any product that may be evaluated in this article or claim that may be made by its manufacturer is not guaranteed or endorsed by the publisher.
Research integrity at Frontiers
Learn more about the work of our research integrity team to safeguard the quality of each article we publish.