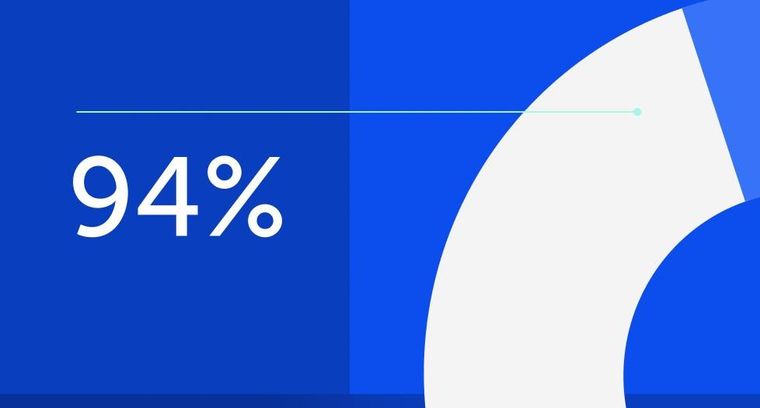
94% of researchers rate our articles as excellent or good
Learn more about the work of our research integrity team to safeguard the quality of each article we publish.
Find out more
ORIGINAL RESEARCH article
Front. Chem., 04 May 2022
Sec. Catalytic Reactions and Chemistry
Volume 10 - 2022 | https://doi.org/10.3389/fchem.2022.880884
This article is part of the Research TopicHot Topic: Chemical Reactions & Catalysis for a Sustainable FutureView all 6 articles
As an efficient and durable engine, a diesel engine has a broad application. However, soot particles (PM) and nitrogen oxides (NOx) coming from diesel engines are the main causes of air pollution, so it is necessary to design and prepare an effective catalyst for the simultaneous elimination of PM and NOx. In this work, a novel 3DOM ZrTiO4 support and a series of WxCeMnOδ/3DOM ZrTiO4 catalysts (where x indicates the wt% of W) were designed and fabricated by the colloidal crystal template technique. Among the as-prepared catalysts, the W1CeMnOδ/3DOM ZrTiO4 catalyst exhibits the highest NO conversion rate (52%) at the temperature of maximum CO2 concentration (474°C) and achieves 90% NO conversion in the temperature range of 250–396°C. The excellent catalytic performance is associated with the macroporous structure, abundant oxygen vacancies, sufficient acid sites, and the synergistic effect among the active components. The possible reaction mechanisms of WxCeMnOδ/3DOM ZrTiO4 catalysts were also discussed based on the characterization results.
Diesel engines are ideal for heavy-duty vehicles, with high durability, lower cost, and longevity (Wei et al., 2011; Chen et al., 2016; Dai et al., 2016; Cheng et al., 2017a). However, a lot of soot particles (PM) and nitrogen oxides (NOx) are simultaneously generated during diesel combustion (Epling et al., 2004; Liu and Gao, 2011). PM and NOx are the main causes of urban haze weather, which can lead to serious environmental pollution and health problems (Zhu et al., 2008; Li et al., 2012; Shen et al., 2013; Choi and Lee, 2014). As more and more stringent emission standards are set by governments, there is a growing interest in developing technologies which enable the reduction of such emissions.
At present, after-treatment technologies are widely used to eliminate soot particulates and NOx (Gogos et al., 2014; Urán et al., 2019); that is, a catalyzed diesel particulate filter (CDPF) is applied to eliminate soot particulates (Wei et al., 2011; Yu et al., 2017), and the selective catalytic reduction (SCR) technology or the nitrogen oxide storage reduction (NSR) technology is simultaneously matched for the NOx removal (Li et al., 2015; Li et al., 2016). However, traditionally, the after-treatment technique possesses some inherited flaws, such as big system volume, large mass, and high cost. In view of this, the idea of the concurrent catalytic elimination of soot particles and NOx in a single trap is attractive and has been initially projected by Yoshida et al. (1989). This approach has attracted great interest from researchers, as it reduces the pressure and shrinks the volume and mass of the system. However, a few shortcomings are still there and are yet to be overcome. For example, the PM combustion process is a characteristic gas–solid–solid catalytic reaction; in general, the diameter of PM is larger than the pore diameter of conventional catalysts, so the soot particles cannot be efficiently transported through the pores of catalytic materials and the active sites cannot be fully used either. In addition, NOx conversion takes place in a lean-burn condition, but the excessive O2 and insufficient reductant will result in a low NOx conversion.
Many catalysts have been developed for the simultaneous elimination of soot particulates and NOx, such as noble metals (Serhan et al., 2019), metal oxides (Bueno-López et al., 2016; Cheng et al., 2017b), perovskite oxides (Liu et al., 2008; Li et al., 2010; Wang et al., 2010), zeolites (Fritz and Pitchon, 1997), and spinel phases (Lin et al., 2009). Among these materials, metal oxides have shown excellent performances (Li et al., 2009; Shen et al., 2013). Mn-based catalysts have been proven to be exceptional in PM combustion and SCR reactions (Chen et al., 2019; Yu D. et al., 2021; Peng et al., 2021). As a promoter, even active catalysts, namely, CeO2-containing materials, are applied widely because of their excellent ability for oxygen storage and the aptitude to shift between Ce4+ and Ce 3+ under a stipulated redox environment (Jin et al., 2014). Specifically, tungsten species have been reported to have the function to advance the catalytic availability of active sites, oxygen-related vacancy, and acid-introduced sites (Xiong et al., 2017). In addition, TiO2 is always busy as support for SCR catalysts (Wu et al., 2011), and by the way, adding additional elements may increase the surface area and thermal stability, and enhance the surface acidity.
Herein, a new type of ZrTiO4 support with a three-dimensional ordered macroporous (3DOM) structure was synthesized by the colloidal crystal templating (CCT) technique, which is rarely reported in the previous literature. Meanwhile, three cheap active elements of W, Ce, and Mn were added to the 3DOM ZrTiO4 support by a simple and convenient method to simultaneously remove nitrogen oxides and soot particles from diesel exhausts. The as-prepared catalysts have a three-dimensional ordered, inter-connected macroporous structure, which can effectively improve the contact performance between reactants and catalytic active sites, and facilitate the effective transmission of particulate reactants. NH3 as a reducing gas was also introduced in order to improve the reduction of NOx.
The 3DOM ZrTiO4 support was fabricated by the colloidal crystal templating (CCT) technique, and the template was prepared by using polymethyl methacrylate (PMMA) spheres (Xu et al., 2011). In a typical process, C16H36O4Ti and ZrOCl2·8H2O (at a molar ratio of 1:1) were taken in the mixed solution of methanol and ethylene glycol (at a volume ratio of 3:7), and strongly stirred followed by the addition of the PMMA template. The mixture was maintained for 3 h until the PMMA templates had been fully impregnated. The excess precursor solution was separated by vacuum filtration. Subsequently, the final precipitate was maintained at 80°C for 12 h to dry. After that, the dried precipitate was calcined at 550°C for 4 h with a raising rate of 1°C/min to remove the template. Finally, 3DOM ZrTiO4 support was obtained.
The 3DOM ZrTiO4 supported catalysts were synthesized by the incipient wetness impregnation method. (NH4)6H2W12O40·xH2O, Ce(NO3)3·6H2O, and Mn(NO3)2·4H2O with different mass ratios were dissolved in distilled water, and then the mixture was added dropwise into the 0.5 g ZrTiO4 support. The volume of the mixture should be equal to the pore volume of the 3DOM ZrTiO4 support. These precursors were dried at 80°C for 12 h, and the dried precipitate was calcined at 550°C for 4 h at a rate of 1°C/min. Finally, WxCeMnOδ/3DOM ZrTiO4 catalysts were obtained, where x means the mass percent of W to ZrTiO4 support. To obtain single metal oxide supported on 3DOM ZrTiO4 catalysts, (NH4)6H2W12O40·xH2O, Ce(NO3)3·6H2O, and Mn(NO3)2·4H2O were individually dissolved in distilled water, and they were added into the ZrTiO4 support dropwise. Next, the treated processes are similar to those of WxCeMnOδ/3DOM ZrTiO4 catalysts. Finally, Mn2O3/3DOM ZrTiO4, CeO2/3DOM ZrTiO4, and WO3/3DOM ZrTiO4 were obtained.
For comparison, W1CeMnOδ/3DOM TiO2 and W1CeMnOδ/3DOM ZrO2 samples were also prepared by the incipient wetness impregnation method. A similar procedure for the preparation of 3DOM ZrTiO4 was applied for the preparation of 3DOM TiO2 and 3DOM ZrO2 supports. 3DOM TiO2 and 3DOM ZrO2 supports were impregnated by the same impregnation solution as that of the W1CeMnOδ/3DOM ZrTiO4 catalyst. The obtained precursor was dried at 80°C for 12 h, and after calculation at 550°C for 4 h, W1CeMnOδ/3DOM TiO2 and W1CeMnOδ/3DOM ZrO2 catalysts were obtained.
The phase composition and crystal structure were evaluated by an X-ray diffractometer (Ultima IV, Rigaku) using Cu-Kα radiation as the source with a Ni filter. Nitrogen adsorption–desorption investigation was achieved with a Micromeritics TriStar II: 3020 analyzer to get the textural characteristics of catalysts. The morphology and microstructure of the catalysts were scrutinized by scanning electron microscopy (SEM, Zeiss sigma 500) and transmission electron microscopy (TEM, JEM-F200). Mn 2p, W 4f, Ce 3d, and O 1s binding energies were measured by X-ray photoelectron spectroscopy (XPS, PHI-1600 ESCA). H2-TPR measurements were conducted using AutoChemi II2920 equipment; 0.05 g sample was pretreated in N2 for 1 h at 300°C, followed by cooling down to 25°C. Thereafter, the flow gas was altered to 10-vol% H2/N2 with a maximum temperature of 900°C, and the heat treatment rate was kept at 10°C/min; hydrogen consumption was examined using a thermal conductivity detector (TCD). NH3-TPD was carried out on a conventional flow apparatus, and 0.05 g sample was heated at 600°C in N2 atmosphere for 1 h and saturated with 1% NH3 for 1 h. When the temperature cooled to room temperature, N2 was used to abolish the feebly attached NH3. At last, the sample was heated to 600°C at a rate of 10°C/min. In situ diffuse reflectance infrared Fourier transform (DRIFT) spectroscopy (Thermo Nicolet Is50 spectrometer) was used to investigate the SCR reaction mechanism of the W1CeMnOδ/3DOM ZrTiO4 catalyst. The catalyst was first purged with NH3 or NO + O2 at 200 and 300°C until adsorption was saturated. Then, the NH3 or NO + O2 were closed. After that, the reaction system was purged by N2, and then, the other corresponding reacted gases were introduced into the in situ reaction cell, and the FT-IR spectra were recorded at different times.
A fixed bed reactor was applied to evaluate the performance of the as-prepared catalysts for the simultaneous elimination of PM and NOx. The reaction gases comprised 1,000 ppm NH3, 1,000 ppm NO, and 5% O2; the balance gas was N2; and the total flow rate of the gases was 100 ml/min. The catalyst (100 mg) and PM (10 mg) were mixed together, and PM was simulated by Printex-U (Degussa). The reacted gas concentrations (including NH3, N2O, NO, NO2, CO, and CO2) were tested by the infrared spectrometer. An accurate and reliable quantitative method was established to measure the multiple gaseous components (Qin and Cadet, 1997; Valencia et al., 2009; Sinelli et al., 2010; Stec et al., 2011). The catalytic performance of the oxidation PM was evaluated by the value of Tm, which was defined as the temperature for maximum CO2 concentration released. The NO reduction was defined by the highest conversion of NO to N2, and the conversion rate was calculated as follows (Zhang et al., 2011):
in which [NO]inlet and [NO]outlet, respectively, denote the inlet and outlet concentrations of NO under steady-state conditions.
The activities of WxCeMnOδ/3DOM ZrTiO4 catalysts for the simultaneous elimination of PM and NOx were evaluated, and the results are shown in Figure 1 and Table 1. The lower Tm of PM oxidation means high catalytic efficiency, which is important for the design and preparation of catalysts. As shown in Figure 1A and Table 1, the Tm value of 3DOM ZrTiO4 support for the removal of PM is 581°C, indicating that the catalytic activity of the support is very weak. Compared with Mn2O3/3DOM ZrTiO4 and CeO2/3DOM ZrTiO4 catalysts, the WO3/3DOM ZrTiO4 catalyst has the highest Tm value of 559°C. When W is combined with Ce or Mn, the Tm of the bimetallic supported catalysts decreases. The Tm values of the W1MnOδ/3DOM ZrTiO4 and W1CeOδ/3DOM ZrTiO4 catalysts are 536 and 558°C, respectively. Interestingly, for the WxCeMnOδ/3DOM ZrTiO4 catalysts, the Tm values are all below 500°C. With increasing doping amounts of W, Tm is slightly raised. This indicates that W has a low activity for soot removal, and its activity can be greatly improved by combining with Ce and Mn. Compared with the W1CeMnOδ/3DOM TiO2 catalyst, the W1CeMnOδ/3DOM ZrO2 catalyst has a lower Tm value of 453°C, and the W1CeMnOδ/3DOM ZrTiO4 catalyst has the Tm value of 474°C, which indicates that the catalytic activity can be improved by adding Zr to Ti.
FIGURE 1. CO2 concentration cures (A) and NO conversion curves (B) for the simultaneous elimination of PM and NOx over 3DOM catalysts.
TABLE 1. Peak temperature of maximum CO2 concentration and 90% temperature window of NO conversion over 3DOM catalysts.
The catalytic performance of NO reduction was evaluated by an operating temperature window (90% NO conversion). As shown in Figure 1B and Table 1, 3DOM ZrTiO4 support shows poor performance for NO conversion, and the conversion of over 50% can hardly be obtained on the ZrTiO4 support. Similarly, the WO3/ZrTiO4 catalyst also shows poor NO elimination performance. It is worth noting that the conversion window (90% NO conversion) of the Mn2O3/ZrTiO4 catalyst is only 88°C (157–245°C), and the CeO2/ZrTiO4 catalyst has no conversion window over 90%; when W is added to Mn and Ce, respectively, the conversion window of the W1MnOδ/ZrTiO4 catalyst has barely changed, but the conversion window of W1CeOδ/ZrTiO4 has been greatly improved; the W1CeMnOδ/ZrTiO4 catalyst not only exhibits the wide temperature window (250–396°C) but also has high NO conversion, which manifests that the interaction of Ce and W can widen the temperature window, and Mn has the effect of improving the NO conversion. The WxCeMnOδ/3DOM ZrTiO4 catalysts exhibit good NO conversion performance except for the W2CeMnOδ/3DOM ZrTiO4, indicating that excessive W doping is not beneficial for NO reduction. Compared with W1CeMnOδ/3DOM ZrTiO4, W1CeMnOδ/3DOM TiO2 and W1CeMnOδ/3DOM ZrO2 exhibit low catalytic activity. Therefore, the synergistic effect between Zr and Ti in ZrTiO4 support indeed has a positive effect to improve the catalytic performance. Based on the above analysis, W1CeMnOδ/3DOM ZrTiO4 catalyst not only exhibits the widest temperature window (250–396°C) at a lower temperature for 90% NO conversion but also has the highest NO conversion rate (52%) at the temperature of Tm, which illustrates that the W1CeMnOδ/ZrTiO4 catalyst can be considered as one kind of talented catalysts for the simultaneous elimination of soot particles and nitrogen oxides.
The crystal structures of the as-prepared 3DOM ZrTiO4 support and it-supported catalysts were characterized by XRD measurements, and the results are exhibited in Figure 2. As shown in Figure 2, the main characteristic peaks at 2θ = 24.75°, 30.51°, 32.82°, 35.66°, 50.27°, and 52.98° can be assigned to the (110), (111), (020), (002), (202), and (221) crystal faces of ZrTiO4 support (JCPDS No. 80-1783) (Zhang Y. et al., 2015). The peaks at 2θ = 28.50°, 32.76°, 47.82°, and 56.51° of each sample can be assigned to the (111), (200), (220), and (311) crystal faces of cubic CeO2 (JCPDS PDF# 43-1002) (Lin et al., 2018). The characteristic peaks of manganese and tungsten oxide cannot be observed because the ionic radius of Mn and W are smaller than that of Ce. Therefore, Mn ions and W ions can easily enter the lattice of CeO2. As shown in Figure 2B, the diffraction peak of WxCeMnOδ/3DOM ZrTiO4 catalysts shifts to a higher angle with respect to the diffraction peak of the CeO2/3DOM ZrTiO4 catalyst (i.e., the peak at 2θ = 28.5° shifts to 28.7°), which confirms the above conclusion. The peaks at 2θ = 32.95°, 55.19°, and 23.13° can be assigned to the crystal faces (222), (440), and (211) of α-Mn2O3 for Mn2O3/3DOM ZrTiO4 and W1MnOδ/3DOM ZrTiO4 catalysts (JCPDS No.41-1442) (Saputra et al., 2014). For the W1CeMnOδ/3DOM TiO2 catalyst, the peaks at 2θ = 25.4°, 37.9°, 48.1°, 53.9°, 55.2°, and 62.8° belong to anatase TiO2, in which the crystal faces are (101), (004), (200), (105), (211), and (204), respectively (JCPDS No. 21-1272) (Jiao et al., 2017). For the W1CeMnOδ/3DOM ZrO2 catalyst, the peaks at 2θ = 30.3°, 35.3°, 50.4°, and 60.2° belong to the characteristic peak of ZrO2 (JCPDS No. 50-1089). For the WO3/3DOM ZrTiO4 catalyst, the peaks at 2θ = 23.2°, 23.7°, 24.4°, 28.8°, 34.0°, and 50.2° belong to the characteristic peak of WO3 (JCPDS No. 20-1323).
FIGURE 2. XRD patterns (A) 10–80°, (B) 25–50°of 3DOM materials. (a) ZrTiO4; (b) W1CeMnOδ/ZrO2; (c) Mn2O3/ZrTiO4; (d) CeO2/ZrTiO4; (e) WO3/ZrTiO4; (f)W1CeMnOδ/TiO2; (g) W1CeOδ/ZrTiO4; (h) W1MnOδ/ZrTiO4; (i) W0.5CeMnOδ/ZrTiO4; (j) W1CeMnOδ/ZrTiO4; (k) W2CeMnOδ/ZrTiO4.
The SEM images, as shown in Figure 3, demonstrate that all catalysts have highly ordered macropores. From the distributions of macropores’ diameters for the as-prepared catalysts in Figures 3A-1–D-1, it can be seen that the average diameters of macropores are about 290 ± 20 nm. The diameters of macropores are lower than the PMMA diameter (400 nm), which is related to the shrinkage of polymer templates at high calcination temperature (Cheng et al., 2017a). The skeleton around macropores is constructed by uniform periodically arranged windows (marked with red circles in Figure 3), which form the layers through close linkage between the opening windows. Meanwhile, the highly ordered macropores for the as-prepared catalysts indicate the loading process of metal oxides does not destroy 3DOM structures.
FIGURE 3. SEM images of 3DOM catalysts and histograms of macropores’ diameters. (A) W1CeOδ/ZrTiO4; (B) W1MnOδ/ZrTiO4; (C) W1CeMnOδ/ZrTiO4; (D) W1CeMnOδ/TiO2.
TEM and HRTEM images of the W1CeMnOδ/3DOM ZrTiO4 catalyst are exhibited in Figure 4. Figure 4A shows that the W1CeMnOδ/3DOM ZrTiO4 catalyst has an ordered macroporous structure and the macropores are linked together by windows layer to layer. This well agrees with the SEM results. Furthermore, the surface of 3DOM ZrTiO4 is adhered by well-dispersed nanoparticles (NPs), and no large agglomerated particles can be obtained on the 3DOM skeleton, which indicates that metal oxides are evenly distributed on the surface of ZrTiO4. HRTEM image of W1CeMnOδ/3DOM ZrTiO4 is shown in Figure 4B; as observed from Figure 4B and the insert images of Figure 4B, the lattice fringes with a spacing of 0.32 nm are indexed as (111) planes of CeO2, and the second lattice fringes with a spacing of 0.36 nm correspond to (011) crystal plane of ZrTiO4. To study the distribution of W, Ce, Mn, Zr, Ti, and O elements in W1CeMnOδ/3DOM ZrTiO4, the HAADF-STEM images and EDS elemental mappings were obtained, and they are shown in Figure 5. From Figures 5B–D, the elements of O, Ti, and Zr cover the entire 3DOM skeleton because O, Ti, and Zr are the constituent elements of the support. From Figures 5E, F, it can be seen that the elements of W, Ce, and Mn are found throughout the surface of the catalyst, even inside the pores.
FIGURE 5. HAADF-STEM image and EDS elemental mappings of W1CeMnOδ/3DOM ZrTiO4. (A) STEM; (B–G) EDS mappings of O, Ti, Zr, W, Ce, and Mn.
The N2 adsorption–desorption isotherms and pore distribution curves of WxCeMnOδ/3DOM ZrTiO4 samples are presented in Figures 6, 7. The BET data of the as-prepared catalysts are summarized in Table 2. As shown in Figure 6, all the samples present typical II curves with a nearly linear relationship in the low-pressure vicinity. For 3DOM ZrTiO4 support, the H3 hysteresis loop increases slowly in the P/P0 range of 0.4–1.0, which may be due to the scraggly surface of the support. When the active components are loaded on the 3DOM ZrTiO4 support, the H3 hysteresis loop disappeared in the P/P0 range of 0.4–0.8 and the intensity in the P/P0 range of 0.8–1.0 increased sharply. This phenomenon may be due to the scraggly surface of the support being covered by the finely dispersed metal oxide, which makes the surface smooth (Xie et al., 2013).
FIGURE 6. Nitrogen adsorption–desorption isotherms of 3DOM catalysts. (A) ZrTiO4; (B) W1MnOδ/ZrTiO4; (C) W1CeOδ/ZrTiO4; (D) W1CeMnOδ/TiO2; (E) W0.5CeMnOδ/ZrTiO4; (F) W1CeMnOδ/ZrTiO4; (G) W2CeMnOδ/ZrTiO4.
FIGURE 7. Mesoporous distribution curves of 3DOM catalysts. (A) ZrTiO4; (B) W1MnOδ/ZrTiO4; (C) W1CeOδ/ZrTiO4; (D) W1CeMnOδ/TiO2; (E) W0.5CeMnOδ/ZrTiO4; (F) W1CeMnOδ/ZrTiO4; (G) W2CeMnOδ/ZrTiO4.
As shown in Figure 7, the as-prepared catalysts exhibit an obvious mesoporous structure. For 3DOM ZrTiO4 support, the mesopores with a diameter of 2–5 nm belong to the surface gap of the ZrTiO4 skeleton, and the mesopores with a diameter of 20–35 nm are associated with the accumulation of metal oxide NPs. As shown in Table 2, 3DOM ZrTiO4 exhibits the biggest surface area of 54.0 m2g−1, which may belong to the scraggly surface of ZrTiO4. However, the surface area decreased when active components are loaded on the ZrTiO4 support, which may be due to the covering of finely dispersed metal oxide on the scraggly surface. In addition, the surface area of W1CeMnOδ/3DOM ZrTiO4 is larger than that of W1CeMn/3DOM TiO2 due to the addition of ZrO2 to TiO2, which is in accordance with other reports (Zhang et al., 2012; Zhang Y. et al., 2015). Compared with the W1MnOδ/3DOM ZrTiO4 catalyst, W1CeMnOδ/3DOM ZrTiO4 exhibits a larger surface area, which is attributed to the addition of Ce. The loaded catalysts display a higher total pore volume and average pore size, and this is related to the accumulation effect of active components.
To investigate the valence state of elements and surface composition of the as-prepared catalysts, XPS measurements were carried out, and the results are shown in Table 3 and Figure 8. The ratios of Mn4+/Mn3+, Ce3+/Ce4+, and (O− + O2−)/O2- of W1CeMnOδ/3DOM ZrTiO4 are comparatively higher than those of other catalysts, indicating that tungsten, ceria, manganese, and supports have strong interaction, which leads to the good catalytic performance of the W1CeMnOδ/3DOM ZrTiO4 catalyst.
FIGURE 8. XPS spectra of Mn 2p (A), Ce3d (B), W4f (C), and O 1s (D). (a) Mn2O3; (b) CeO2; (c) WO3; (d) W1CeMnOδ/TiO2; (e) W0.5CeMnOδ/ZrTiO4; (f) W1CeMnOδ/ZrTiO4; (g) W2CeMnOδ/ZrTiO4; (h) W1CeOδ/ZrTiO4; (i) W1MnOδ/ZrTiO4.
Figure 8A shows the XPS profiles of Mn 2p for the as-prepared catalysts. The spectra of all the catalysts display two peaks. The peaks at 641.2 and 642.9 eV can be assigned to Mn3+ and Mn4+ (Li et al., 2016), respectively. It was well known that Mn4+ species are beneficial for low-temperature SCR (Yao et al., 2019; Li et al., 2021). As shown in Table 3, the ratio of Mn4+/Mn3+ of W1CeMnOδ/3DOM ZrTiO4 is higher than that of W1CeMnOδ/3DOM TiO2. In addition, the ratio of Mn4+/Mn3+ in the WxCeMnOδ/3DOM ZrTiO4 catalysts is higher than that of the WMnOδ/3DOM ZrTiO4 catalyst, which indicates the strong synergistic effects of Mn and Ce. Ce can accelerate the oxidation of Mn3+ to Mn4+; hence, more Mn4+can be produced.
Figure 8B shows the XPS profiles of Ce 3d. The peaks at about 900.5 and 883.5 eV denoted as u’ and v’ are the major peaks related to the 3d104f1 state of Ce3+ ions, and the peaks at about 882.0, 888.8, 897.8, 900.4, 907.1, and 916.2 eV named as v, v’’, v’’’, u, u’’, and u’’’ are related to the 3d104f0 state ascribed to Ce4+(Cheng et al., 2014). According to the peak area ratio of Ce3+ to Ce4+, the content of Ce3+ of monometallic oxide CeO2 is lowest in the as-prepared catalysts, indicating that the support and the doping of other metals can promote the production of more Ce3+. Meanwhile, the content of Ce3+ in W1CeMnOδ/3DOM ZrTiO4 is higher than that of W1CeMnOδ/3DOM TiO2, which indicates the synergistic effect of Zr and Ti. Similarly, the highest ratio of Ce3+/Ce4+ is also found in W1CeMnOδ/3DOM ZrTiO4, which indicates that the interaction between W and Ce will produce more Ce3+.
Figure 8C gives the W 4f5/2 and W 4f7/2 peaks at 36.6–37.6 and 34.7–35.7 assigned to W6+. As shown in Figure 8C, W1CeMnOδ/3DOM ZrTiO4 has higher binding energy than other catalysts. The higher binding energy generally represents the lower density of electron cloud, as W6+ has only four coordination bonds with surrounding O-atoms which can generate excess electrons, so Ce4+can be substituted by W6+and produce one or two excess electrons, and the excess electrons will be compensated by producing one or two Ce3+; these Ce3+ ions play a crucial role in the generation of oxygen vacancy due to its charge imbalance and unsaturated chemisorption bond; thus, it further enhances the catalytic activity greatly (Liu et al., 2020).
As shown in Figure 8D, O 1s peaks are fitted into two peaks at 528.0–530.0 and 530.0–532.0eV (according to Gaussian bands), and those peaks are ascribed to lattice oxygen (O2−, denoted as Oβ) and chemically adsorbed oxygen (O2− and/or O−, denoted as Oα), respectively (Zhang S. et al., 2015). Generally speaking, Oα is active oxygen species that has higher mobility than lattice oxygen, and it is the determining factor for low-temperature NH3-SCR reaction and soot oxidation. Because gas-phase NO is more easily obtained and reacts with active oxygen species and forms NO2, NO2 will react with NO and NH3 in the fast-SCR mode and react with soot directly. Hence, the rates for NH3-SCR and soot oxidation reactions were enhanced. Based on the results in Table 3 and Figure 1, the Oα/Oβ ratio of monometallic oxide Mn2O3, CeO2, and WO3 is lower than that of other as-prepared catalysts, which manifest the effect of supports and other doping metals. W1CeMnOδ/3DOM ZrTiO4 has the highest Oα/Oβ ratio of 51.5% among the as-prepared catalysts and shows the highest NO conversion rate at temperature Tm. Therefore, the high ratio of Oα/Oβ plays an important role in the simultaneous catalytic elimination of PM and NOx.
Catalysts with excellent redox properties are required in the simultaneous removal reaction. H2-TPR is usually applied for measuring the redox ability of catalysts. Figure 9 gives the H2-TPR results of the as-prepared catalysts. As shown in Figure 9A, the 3DOM ZrTiO4 support has almost no reduction peak, indicating that the redox capacity of the support is very weak. CeO2/3DOM ZrTiO4 has one reduction peak at 618°C, which is related to the reduction of surface oxygen species of ceria (Ma et al., 2012), since the reduction of bulk ceria occurred only above 750°C (Andreeva et al., 2004; Ndifor et al., 2007). When W is added into CeO2/3DOM ZrTiO4, the shoulder peak at 618°C belongs to the reduction of surface CeO2, and the peaks at 695°C are assigned to the reduction of surface WOx (Ma et al., 2012). When Mn is doped into CeO2/ZrTiO4, three reduction peaks can be obtained. The first peak at 365°C is associated with the reduction of MnO2 to Mn2O3, the peak at 465°C is related to the reduction of surface Mn2O3 to Mn3O4, and the third shoulder peak at 531°C belongs to the reduction of surface Mn3O4 to MnO (Yu et al., 2014).
FIGURE 9. H2-TPR profiles of the 3DOM catalysts. (A) ZrTiO4; (B) CeO2/ZrTiO4; (C) W1CeOδ/ZrTiO4; (D) W1MnOδ/ZrTiO4; (E) W1CeMnOδ/TiO2; (F) W0.5CeMnOδ/ZrTiO4; (G) W1CeMnOδ/ZrTiO4; (H) W2CeMnOδ/ZrTiO4.
As shown in Figure 9E, W1CeMnOδ/3DOM TiO2 displays one reduction peak at 490 °C, which belongs to the overlapped reduction of surface oxygen. For WxCeMnOδ/3DOM ZrTiO4 catalysts, the TPR curves display two broad peaks, the former can belong to the reductions of the MnO2 to Mn2O3 and Mn2O3 to Mn3O4, and the latter may be related to the reductions of Mn3O4 to MnO, CeO2 to Ce2O3, and WOx to W. Among the WxCeMnOδ/3DOM ZrTiO4 catalysts, the peaks of the W1CeMnOδ/3DOM ZrTiO4 catalyst shift to lower temperatures and the peak in the lower temperature has a wider reduction peak area, indicating that the 3DOM W1CeMnOδ/3DOM ZrTiO4 catalyst has strong reduction ability and much Mn4+ species. The Mn4+/Mn3+ ionic couple have proper redox characteristics and easily deliver more active sites for improving activity; therefore, the W1CeMnOδ/3DOM ZrTiO4 catalyst shows good simultaneous removal activity.
Surface acidity plays a significant role in NH3-SCR reaction (Xu et al., 2016; Xu et al., 2017). NH3-TPD was used to evaluate the amount and strength of surface acid sites. Therefore, the NH3-TPD profiles of the as-prepared catalysts were tested, and the results are shown in Figure 10. All the catalysts have broad peaks in the temperature range of 50–350°C, which is related to the desorption of NH3 on the weak and medium acid sites. Interestingly, these peaks are nearly at the same location, and no significant difference can be observed. Compared with the W1CeMnOδ/3DOM TiO2 catalyst, the peak area of the W1CeMnOδ/3DOM ZrTiO4 catalyst is larger, which indicates that the W1CeMnOδ/3DOM ZrTiO4 catalyst has more acid sites due to the doping of Zr in the ZrTiO4 support. In comparison with W1MnOδ/3DOM ZrTiO4 catalyst, more acid sites can be seen in W1CeMnOδ/3DOM ZrTiO4, and these acid sites may come from the electron-unsaturated W6+, as W6+enter the CeO2 lattice, leading to lower coordination between W6+ and surrounding oxygen, so it needs to share more electrons to balance the charge transfer. Therefore, the above analyses verify the experimental results that the W1CeMnOδ/3DOM ZrTiO4 catalyst has the highest NO removal activity.
FIGURE 10. NH3-TPD profiles of the 3DOM catalysts. (A) W1CeOδ/ZrTiO4; (B) W1MnOδ/ZrTiO4; (C) W1CeMnOδ/TiO2; (D) W0.5CeMnOδ/ZrTiO4; (E) W1CeMnOδ/ZrTiO4; (F) W2CeMnOδ/ZrTiO4.
Soot combustion is the reaction of gas–solid–solid, and the contact efficiency between catalyst and soot is a significant factor for controlling the catalytic activity. 3DOM catalysts have highly ordered macroporous structures with diameter higher than 100 nm (SEM results). Because the diameter of soot particles (≈25 nm) is smaller than that of macropores, the large pores can capture soot particles and transfer soot particles to the inner active sites so that the active sites of catalysts can be fully utilized. Meanwhile, the diffusion resistance is reduced due to highly ordered macropores. Some previous work also confirmed the effect of the macroporous structure on improving catalytic activity (Yu et al., 2019; Yu X. et al., 2021).
In addition, the effects of NPs also play important roles in removing soot particles, such as the quantum size effects and surface effects. In this work, the active components are formed nanoparticles on the surface of 3DOM ZrTiO4 support. From the TEM results, it can be found that the diameter of active components is well falling into the scale of NPs. At the same time, gaseous reactants are more easily absorbed due to a lot of NPs on the surface of 3DOM ZrTiO4 supports, thus improving the efficiency of the catalytic reaction.
To more deeply understand the reaction essence of simultaneous deSoot and deNOx, according to the results in this work and previous reports, the possible reaction mechanisms are also speculated and described in Scheme 1.
For the reaction of deSoot, based on the results of XPS (Figure 8) and H2-TPR (Figure 9), the 3DOM W1CeMnOδ/3DOM ZrTiO4 catalyst has more Mn4+, Ce3+, and Oα than other as-prepared catalysts, which indicates that it has more active sites, so gas-phase O2 molecules are more easily to be adsorbed and activated on the active sites (oxygen vacancies). On the one hand, adsorbed O2 forms active oxygen species, then soot traps the active oxygen species and forms surface oxygen–carbon complexes (SOC), and finally, the SOC further decomposes and produces CO2 and CO. On the other hand, these active oxygen species react with NO to form NO2; NO2 has stronger oxidation capacity than active oxygen species, so it can react with soot directly and changes the reaction path from gas–solid–solid to gas–gas–solid, thus accelerating the process of soot combustion. Therefore, the W1CeMnOδ/3DOM ZrTiO4 catalyst has a lower Tm value of 474°C (Figure 1).
For the reaction of deNOx, in order to investigate the SCR reaction mechanism of the W1CeMnOδ/3DOM ZrTiO4 catalyst, in situ DRIFTS were measured at 200 and 300°C, and the results are shown in Figure 11. As shown in Figure 11A, after being purged by N2, several absorbance bands were observed. The bands centered at 1,198 and 1,633 cm −1 are ascribed to adsorbed NH3 on Lewis acidic sites, and the bands at 1,396 cm−1 belong to the coordinated NH4+on Bronsted acid sites, while the bands at 3,100–3,500 cm−1 are attributed to N-H stretching vibration of coordinated ammonia (Tan et al., 2018; Liu et al., 2020). When NO + O2 was introduced into the in situ reaction cell, all the bands belonging to the ammonia species are reduced gradually in their intensities with increasing time, and the IR bands (1,198 and 3,100–3,500 cm−1) disappear after 20 min. At the same time, the nitrate species including bidentate nitrate (1,273 cm−1) and monodentate nitrate (1,520 cm−1) begin to appear on the surface of the catalyst, including bidentate nitrate (1,273 cm−1) and monodentate nitrate (1,520 cm−1). These results indicate that the gas phase NO reacts with the coordinated NH3 on the surface of the W1CeMnOδ/3DOM ZrTiO4 catalyst through the E-R mechanism.
FIGURE 11. In situ DRIFTS spectra of the W1CeMnOδ/ZrTiO4 catalyst under different temperatures and reaction gases. (A) The reaction between NO + O2 and pre-adsorbed NH3 at 200°C; (B) reaction between NH3 and pre-adsorbed NO + O2 at 200°C; (C) reaction between NO + O2 and pre-adsorbed NH3 at 300°C; (D) reaction between NH3 and pre-adsorbed NO + O2 at 300°C.
As shown in Figure 11B, after being purged by N2, bidentate nitrates (1,565 cm−1) and bridging nitrates (1,649 cm−1) (Liu et al., 2020; Wang et al., 2020) were formed on the surface of the W1CeMnOδ/3DOM ZrTiO4 catalyst because the mixture of NO + O2 was adsorbed on the surface of the catalyst. When NH3 was introduced into the in situ reaction cell, the bands related to nitrate species decreased gradually in the first 10 min, and no adsorption peaks were found for NH3 species, indicating that the gas phase NH3 reacts with the nitrate species. After 20 min, the bands belonging to NH3 species begin to appear, indicating the existence of ammonia on the surface of the catalyst; the bands at 1,189 and 1,672 cm −1 belong to the adsorbed NH3 on Lewis acidic sites; the bands at 1,422 cm−1 belong to the coordinated NH4+on Bronsted acid sites; and the peaks at 3,100–3,500 cm−1 belong to N-H stretching vibration of coordinated ammonia. Interestingly, the bands at 1,565cm−1 (bidentate nitrates) begin to shift after 5 min and decrease slowly within 15 min. After 15 min, the peak at 1,565 cm−1 (bidentate nitrates) disappeared and the peak belonged to the NH3 species increased gradually, so the IR band at 1,672 cm−1 may be caused by the overlap of the bidentate nitrates (1,565 cm−1), bridging nitrates (1,649 cm−1), and the coordinated NH3 adsorbed on the Lewis acid site (1,672 cm−1). Based on the above results and discussion, it can be found that bidentate nitrate (1,565 cm−1) and active NH3 can coexist on the surface of the W1CeMnOδ/3DOM ZrTiO4 catalyst and the SCR reaction mechanism of the W1CeMnOδ/3DOM ZrTiO4 catalyst can be both E-R mechanism and L-H mechanism at 200°C.
Figures 11C, D show the in situ DRIFT spectra of the W1CeMnOδ/3DOM ZrTiO4 catalyst measured at 300°C. As shown in Figure 11C, similarly, the catalyst was first treated by NH3. After being purged by N2, the bands centered at 1,633 cm −1 are ascribed to the adsorbed NH3 on Lewis acidic sites, and the bands at 3,100–3,500 cm−1 are attributed to N-H stretching vibration of coordinated ammonia. When NO + O2 was introduced into the in situ reaction cell, the intensities of all the bands belonging to the ammonia species gradually reduced, and the IR bands (3,100–3,500 cm−1) disappeared after 20 min. The bands, which belong to nitrate species, appear; the band centered at 1,232 cm −1 is ascribed to bridging nitrate; and the band at 1,362 cm−1 is attributed to bidentate nitrate. These results indicate that gas phase NO reacts with coordinated NH3 also through the E-R mechanism at 300°C.
As shown in Figure 11D, the catalyst was first treated with NO + O2. After purging with N2, the bands assigned to bridging nitrates (1,204 cm−1) and monodentate nitrates (1,283 cm−1) were detected to demonstrate the formation of these nitrate species on the surface of the catalyst. When NH3 was introduced, the band intensity related to nitrate species decreased gradually in the first 10 min, and no adsorption peaks were found for NH3 species. After 20 min, several bands began to appear; the bands at 1,214 cm−1 are ascribed to adsorbed NH3 on Lewis acidic sites, and the bands at 3,100–3,500 cm−1 are attributed to N-H stretching vibrational of coordinated ammonia. Similar to the case at 200°C, the bands at 1,204 cm−1 were shifted after 5 min and band intensity decreased slowly. After 15 min, the peaks at 1,204 and 1,283 cm−1 disappeared, and the intensity of the peak belonging to the NH3 species was increased gradually. The IR band at 1,214 cm−1 may be the overlap of the bridging nitrates (1,204 cm−1), monodentate nitrates (1,283 cm−1), and the adsorbed NH3 species. These results indicate that the reaction at 300°C is similar to the reaction at 200°C.
To sum up, the reaction mechanism for the simultaneous deSoot and deNOx is a complex mechanism that mixes two reactions together. In this work, four mechanisms, including the active oxygen mechanism, NO2-assisted mechanism, L-H mechanism, and E-R mechanism, were proposed, and these four mechanisms work together in the simultaneous elimination reaction.
3DOM ZrTiO4 support and a series of WxCeMnOδ/3DOM ZrTiO4 oxide catalysts were fabricated by the colloidal crystal template method and applied to the simultaneous elimination of PM and NOx. Based on the analyses of characterization and activity evaluation results, the as-prepared catalysts have a high-quality 3DOM structure, and the W1CeMnOδ/3DOM ZrTiO4 catalyst exhibits the best catalytic performance due to the perfect structure, large surface area, abundant acid sites, and the synergistic effect among the active components. Among the as-prepared catalysts, W1CeMnOδ/3DOM ZrTiO4 exhibits the widest temperature window (250–396°C) at a lower temperature for 90% NO conversion but also has the highest NO conversion rate (52%) at the temperature of Tm for soot combustion. The catalytic mechanism for the simultaneous elimination of soot particulate matter and nitrogen oxides over W1CeMnOδ/3DOM ZrTiO4 catalyst is mainly governed via the active oxygen mechanism, NO2-assisted mechanism, L-H mechanism, and E-R mechanism. The as-prepared WxCeMnOδ/3DOM ZrTiO4 catalysts have application prospects for the simultaneous elimination of soot particulate matter and nitrogen oxides from diesel engine exhausts, owing to easy preparation, low cost, and high catalytic activity.
The original contributions presented in the study are included in the article/Supplementary Material, further inquiries can be directed to the corresponding authors.
RW conducted the catalyst preparation and wrote the manuscript. CZ and DL performed the activity test and characterization. ZS, AK, YW, and JL discussed the mechanism part. XY and ZZ conceived the project and improved the text.
This work was supported by the Key Research and Development Program of MOST (2017YFE0131200), the National Natural Science Foundation of China (U1908204, 22072095, and 21761162016), the University Joint Education Project for China-Central and Eastern European Countries (2021097), the National Engineering Laboratory for Mobile Source Emission Control Technology (NELMS 2018A04), Education Office of Liaoning Province (LJC202004 and LJC202005), the University level innovation team of Shenyang Normal University, Major Incubation Program of Shenyang Normal University (ZD201901), and the National Centre for Research and Development, Poland, grant PNOX no. WPC1/PNOX/2019 (MOST program).
The authors declare that the research was conducted in the absence of any commercial or financial relationships that could be construed as a potential conflict of interest.
All claims expressed in this article are solely those of the authors and do not necessarily represent those of their affiliated organizations, or those of the publisher, the editors, and the reviewers. Any product that may be evaluated in this article, or claim that may be made by its manufacturer, is not guaranteed or endorsed by the publisher.
Andreeva, D., Nedyalkova, R., Ilieva, L., and Abrashev, M. V. (2004). Gold-vanadia Catalysts Supported on Ceria-Alumina for Complete Benzene Oxidation. Appl. Catal. B: Environ. 52, 157–165. doi:10.1016/j.apcatb.2004.03.019
Bueno-López, A., Lozano-Castelló, D., and Anderson, J. A. (2016). NOx Storage and Reduction over Copper-Based Catalysts. Part 1: BaO + CeO 2 Supports. Appl. Catal. B: Environ. 198, 189–199. doi:10.1016/j.apcatb.2016.05.067
Chen, B., Liu, Y., Chen, S., Zhao, X., Yue, W., and Pan, X. (2016). Nitrogen-rich Core/shell Magnetic Nanostructures for Selective Adsorption and Separation of Anionic Dyes from Aqueous Solution. Environ. Sci. Nano 3, 670–681. doi:10.1039/c6en00022c
Chen, M., Wang, L., Yu, X., and Zhao, Z. (2019). Application of Mn-Based Catalysts for the Catalytic Combustion of Diesel Soot. Prog. Chem. 31, 723–737.
Cheng, K., Liu, J., Zhang, T., Li, J., Zhao, Z., Wei, Y., et al. (2014). Effect of Ce Doping of TiO2 Support on NH3-SCR Activity over V2O5-WO3/CeO2-TiO2 Catalyst. J. Environ. Sci. 26, 2106–2113. doi:10.1016/j.jes.2014.08.010
Cheng, Y., Liu, J., Zhao, Z., Wei, Y., Song, W., and Xu, C. (2017a). The Simultaneous Purification of PM and NOx in Diesel Engine Exhausts over a Single 3DOM Ce0.9−xFe0.1ZrxO2 Catalyst. Environ. Sci. Nano 4, 1168–1177. doi:10.1039/c7en00170c
Cheng, Y., Song, W., Liu, J., Zheng, H., Zhao, Z., Xu, C., et al. (2017b). Simultaneous NOx and Particulate Matter Removal from Diesel Exhaust by Hierarchical Fe-Doped Ce-Zr Oxide. ACS Catal. 7, 3883–3892. doi:10.1021/acscatal.6b03387
Choi, B., and Lee, K.-S. (2014). LNT/CDPF Catalysts for Simultaneous Removal of NOx and PM from Diesel Vehicle Exhaust. Chem. Eng. J. 240, 476–486. doi:10.1016/j.cej.2013.10.100
Dai, Y., Yao, J., Song, Y., Wang, S., and Yuan, Y. (2016). Enhanced Adsorption and Degradation of Phenolic Pollutants in Water by Carbon Nanotube Modified Laccase-Carrying Electrospun Fibrous Membranes. Environ. Sci. Nano 3, 857–868. doi:10.1039/c6en00148c
Epling, W. S., Campbell, L. E., Yezerets, A., Currier, N. W., and Parks, J. E. (2004). Overview of the Fundamental Reactions and Degradation Mechanisms of NOx Storage/Reduction Catalysts. Catal. Rev. 46, 163–245. doi:10.1081/cr-200031932
Fritz, A., and Pitchon, V. (1997). The Current State of Research on Automotive Lean NOx Catalysis. Appl. Catal. B: Environ. 13, 1–25. doi:10.1016/s0926-3373(96)00102-6
Gogos, A., Kaegi, R., Zenobi, R., and Bucheli, T. D. (2014). Capabilities of Asymmetric Flow Field-Flow Fractionation Coupled to Multi-Angle Light Scattering to Detect Carbon Nanotubes in Soot and Soil. Environ. Sci. Nano 1, 584–594. doi:10.1039/c4en00070f
Jiao, J., Wei, Y., Zhao, Y., Zhao, Z., Duan, A., Liu, J., et al. (2017). AuPd/3DOM-TiO 2 Catalysts for Photocatalytic Reduction of CO 2 : High Efficient Separation of Photogenerated Charge Carriers. Appl. Catal. B: Environ. 209, 228–239. doi:10.1016/j.apcatb.2017.02.076
Jin, R., Liu, Y., Wang, Y., Cen, W., Wu, Z., Wang, H., et al. (2014). The Role of Cerium in the Improved SO2 Tolerance for NO Reduction with NH3 over Mn-Ce/TiO2 Catalyst at Low Temperature. Appl. Catal. B: Environ. 148-149, 582–588. doi:10.1016/j.apcatb.2013.09.016
Li, H., Wu, S., Wu, C.-Y., Wang, J., Li, L., and Shih, K. (2015). SCR Atmosphere Induced Reduction of Oxidized Mercury over CuO-CeO2/TiO2 Catalyst. Environ. Sci. Technol. 49, 7373–7379. doi:10.1021/acs.est.5b01104
Li, Q., Meng, M., Dai, F., Zha, Y., Xie, Y., Hu, T., et al. (2012). Multifunctional Hydrotalcite-Derived K/MnMgAlO Catalysts Used for Soot Combustion, NOx Storage and Simultaneous Soot-NOx Removal. Chem. Eng. J. 184, 106–112. doi:10.1016/j.cej.2012.01.009
Li, W.-J., Li, T.-Y., and Wey, M.-Y. (2021). Preferred Enhancement of Fast-SCR by Mn/CeSiOx Catalyst: Study on Ce/Si Promotion and Shape Dependence. Chem. Eng. J. 403, 126317. doi:10.1016/j.cej.2020.126317
Li, W. B., Yang, X. F., Chen, L. F., and Wang, J. A. (2009). Adsorption/desorption of NOx on MnO2/ZrO2 Oxides Prepared in Reverse Microemulsions. Catal. Today 148, 75–80. doi:10.1016/j.cattod.2009.03.028
Li, X., Li, J., Peng, Y., Chang, H., Zhang, T., Zhao, S., et al. (2016). Mechanism of Arsenic Poisoning on SCR Catalyst of CeW/Ti and its Novel Efficient Regeneration Method with Hydrogen. Appl. Catal. B: Environ. 184, 246–257. doi:10.1016/j.apcatb.2015.11.042
Li, Z., Meng, M., Li, Q., Xie, Y., Hu, T., and Zhang, J. (2010). Fe-substituted Nanometric La0.9K0.1Co1−xFexO3−δ Perovskite Catalysts Used for Soot Combustion, NOx Storage and Simultaneous Catalytic Removal of Soot and NOx. Chem. Eng. J. 164, 98–105. doi:10.1016/j.cej.2010.08.036
Lin, H., Li, Y., Shangguan, W., and Huang, Z. (2009). Soot Oxidation and NOx Reduction over BaAl2O4 Catalyst. Combustion and Flame 156, 2063–2070. doi:10.1016/j.combustflame.2009.08.006
Lin, X., Li, S., He, H., Wu, Z., Wu, J., Chen, L., et al. (2018). Evolution of Oxygen Vacancies in MnOx-CeO2 Mixed Oxides for Soot Oxidation. Appl. Catal. B: Environ. 223, 91–102. doi:10.1016/j.apcatb.2017.06.071
Liu, G., and Gao, P.-X. (2011). A Review of NOx Storage/reduction Catalysts: Mechanism, Materials and Degradation Studies. Catal. Sci. Technol. 1, 552. doi:10.1039/c1cy00007a
Liu, J., Zhao, Z., and Duan, C.-m. A.-j. (2008). Simultaneous Removal of NOx and Diesel Soot over Nanometer Ln-Na-Cu-O Perovskite-like Complex Oxide Catalysts. Appl. Catal. B: Environ. 78, 61–72. doi:10.1016/j.apcatb.2007.09.001
Liu, X., Jiang, P., Chen, Y., Wang, Y., Ding, Q., Sui, Z., et al. (2021). A Basic Comprehensive Study on Synergetic Effects Among the Metal Oxides in CeO2-WO3/TiO2 NH3-SCR Catalyst. Chem. Eng. J. 421, 127833. doi:10.1016/j.cej.2020.127833
Ma, Z., Weng, D., Wu, X., and Si, Z. (2012). Effects of WOx Modification on the Activity, Adsorption and Redox Properties of CeO2 Catalyst for NOx Reduction with Ammonia. J. Environ. Sci. 24, 1305–1316. doi:10.1016/s1001-0742(11)60925-x
Ndifor, E. N., Garcia, T., Solsona, B., and Taylor, S. H. (2007). Influence of Preparation Conditions of Nano-Crystalline Ceria Catalysts on the Total Oxidation of Naphthalene, a Model Polycyclic Aromatic Hydrocarbon. Appl. Catal. B: Environ. 76, 248–256. doi:10.1016/j.apcatb.2007.05.027
Peng, C., Yu, D., Wang, L., Yu, X., and Zhao, Z. (2021). Recent Advances in the Preparation and Catalytic Performance of Mn-Based Oxide Catalysts with Special Morphologies for the Removal of Air Pollutants. J. Mater. Chem. A. 9, 12947–12980. doi:10.1039/d1ta00911g
Qin, D., and Cadet, G. (1997). Quantitative Analysis of Process Streams by On-Line FT-IR Spectrometry. Anal. Chem. 69, 1942–1945. doi:10.1021/ac9610826
Saputra, E., Muhammad, S., Sun, H., Ang, H.-M., Tadé, M. O., and Wang, S. (2014). Shape-controlled Activation of Peroxymonosulfate by Single crystal α-Mn2O3 for Catalytic Phenol Degradation in Aqueous Solution. Appl. Catal. B: Environ. 154-155, 246–251. doi:10.1016/j.apcatb.2014.02.026
Serhan, N., Tsolakis, A., Wahbi, A., Martos, F. J., and Golunski, S. (2019). Modifying Catalytically the Soot Morphology and Nanostructure in Diesel Exhaust: Influence of Silver De-NOx Catalyst (Ag/Al2O3). Appl. Catal. B: Environ. 241, 471–482. doi:10.1016/j.apcatb.2018.09.068
Shen, Q., Lu, G., Du, C., Guo, Y., Wang, Y., Guo, Y., et al. (2013). Role and Reduction of NOx in the Catalytic Combustion of Soot over Iron-Ceria Mixed Oxide Catalyst. Chem. Eng. J. 218, 164–172. doi:10.1016/j.cej.2012.12.010
Sinelli, N., Limbo, S., Torri, L., Di Egidio, V., and Casiraghi, E. (2010). Evaluation of Freshness Decay of Minced Beef Stored in High-Oxygen Modified Atmosphere Packaged at Different Temperatures Using NIR and MIR Spectroscopy. Meat Sci. 86, 748–752. doi:10.1016/j.meatsci.2010.06.016
Stec, A. A., Fardell, P., Blomqvist, P., Bustamante-Valencia, L., Saragoza, L., and Guillaume, E. (2011). Quantification of Fire Gases by FTIR: Experimental Characterisation of Calibration Systems. Fire Saf. J. 46, 225–233. doi:10.1016/j.firesaf.2011.02.004
Tan, J., Wei, Y., Sun, Y., Liu, J., Zhao, Z., Song, W., et al. (2018). Simultaneous Removal of NO and Soot Particulates from Diesel Engine Exhaust by 3DOM Fe-Mn Oxide Catalysts. J. Ind. Eng. Chem. 63, 84–94. doi:10.1016/j.jiec.2018.02.002
Urán, L., Gallego, J., Li, W.-Y., and Santamaría, A. (2019). Effect of Catalyst Preparation for the Simultaneous Removal of Soot and NOx. Appl. Catal. A: Gen. 569, 157–169. doi:10.1016/j.apcata.2018.10.029
Valencia, L. B., Rogaume, T., Guillaume, E., Rein, G., and Torero, J. L. (2009). Analysis of Principal Gas Products during Combustion of Polyether Polyurethane Foam at Different Irradiance Levels. Fire Saf. J. 44, 933–940. doi:10.1016/j.firesaf.2009.05.003
Wang, B., Wang, M., Han, L., Hou, Y., Bao, W., Zhang, C., et al. (2020). Improved Activity and SO2 Resistance by Sm-Modulated Redox of MnCeSmTiOx Mesoporous Amorphous Oxides for Low-Temperature NH3-SCR of NO. ACS Catal. 10, 9034–9045. doi:10.1021/acscatal.0c02567
Wang, K., Qian, L., Zhang, L., Liu, H., and Yan, Z. (2010). Simultaneous Removal of NOx and Soot Particulates over La0.7Ag0.3MnO3 Perovskite Oxide Catalysts. Catal. Today 158, 423–426. doi:10.1016/j.cattod.2010.06.001
Wei, Y., Liu, J., Zhao, Z., Duan, A., Jiang, G., Xu, C., et al. (2011). Three-dimensionally Ordered Macroporous Ce0.8Zr0.2O2-Supported Gold Nanoparticles: Synthesis with Controllable Size and Super-catalytic Performance for Soot Oxidation. Energy Environ. Sci. 4, 2959. doi:10.1039/c0ee00813c
Wu, X., Si, Z., Li, G., Weng, D., and Ma, Z. (2011). Effects of Cerium and Vanadium on the Activity and Selectivity of MnOx-TiO2 Catalyst for Low-Temperature NH3-SCR. J. Rare Earths 29, 64–68. doi:10.1016/s1002-0721(10)60403-6
Xie, S., Dai, H., Deng, J., Liu, Y., Yang, H., Jiang, Y., et al. (2013). Au/3DOM Co3O4: Highly Active Nanocatalysts for the Oxidation of Carbon Monoxide and Toluene. Nanoscale 5, 11207. doi:10.1039/c3nr04126c
Xiong, Z.-b., Liu, J., Zhou, F., Liu, D.-y., Lu, W., Jin, J., et al. (2017). Selective Catalytic Reduction of NO with NH3 over Iron-Cerium-Tungsten Mixed Oxide Catalyst Prepared by Different methodsSelective Catalytic Reduction of NO with NH3 over Iron-Cerium-Tungsten Mixed Oxide Catalyst Prepared by Different Methods. Appl. Surf. Sci. 406, 218–225. doi:10.1016/j.apsusc.2017.02.157
Xu, H., Feng, X., Liu, S., Wang, Y., Sun, M., Wang, J., et al. (2017). Promotional Effects of Titanium Additive on the Surface Properties, Active Sites and Catalytic Activity of W/CeZrOx Monolithic Catalyst for the Selective Catalytic Reduction of NOx with NH3. Appl. Surf. Sci. 419, 697–707. doi:10.1016/j.apsusc.2017.05.055
Xu, H., Li, Y., Xu, B., Cao, Y., Feng, X., Sun, M., et al. (2016). Effectively Promote Catalytic Performance by Adjusting W/Fe Molar Ratio of FeWx/Ce0.68Zr0.32O2 Monolithic Catalyst for NH3-SCR. J. Ind. Eng. Chem. 36, 334–345. doi:10.1016/j.jiec.2016.02.024
Xu, J., Liu, J., Zhao, Z., Xu, C., Zheng, J., Duan, A., et al. (2011). Easy Synthesis of Three-Dimensionally Ordered Macroporous La1−xKxCoO3 Catalysts and Their High Activities for the Catalytic Combustion of Soot. J. Catal. 282, 1–12. doi:10.1016/j.jcat.2011.03.024
Yao, X., Chen, L., Cao, J., Chen, Y., Tian, M., Yang, F., et al. (2019). Enhancing the deNO Performance of MnO/CeO2-ZrO2 Nanorod Catalyst for Low-Temperature NH3-SCR by TiO2 Modification. Chem. Eng. J. 369, 46–56. doi:10.1016/j.cej.2019.03.052
Yoshida, K., Maklno, S., Sumlya, S., and Muramat, C. (1989). Simultaneous Reduction of NOx and Particulate Emissions from Diesel Engine Exhaust. SAE Tech. Paper 1, 1994, 2005).
Yu, D., Ren, Y., Yu, X., Fan, X., Wang, L., Wang, R., et al. (2021a). Facile Synthesis of Birnessite-type K2Mn4O8 and Cryptomelane-type K2-xMn8O16 Catalysts and Their Excellent Catalytic Performance for Soot Combustion with High Resistance to H2O and SO2. Appl. Catal. B: Environ. 285, 119779. doi:10.1016/j.apcatb.2020.119779
Yu, X., Li, J., Wei, Y., Zhao, Z., Liu, J., Jin, B., et al. (2014). Three-Dimensionally Ordered Macroporous MnxCe1-xOδ and Pt/Mn0.5Ce0.5Oδ Catalysts: Synthesis and Catalytic Performance for Soot Oxidation. Ind. Eng. Chem. Res. 53, 9653–9664. doi:10.1021/ie500666m
Yu, X., Ren, Y., Yu, D., Chen, M., Wang, L., Wang, R., et al. (2021b). Hierarchical Porous K-OMS-2/3dom-M Ti0.7Si0.3O2 Catalysts for Soot Combustion: Easy Preparation, High Catalytic Activity, and Good Resistance to H2O and SO2. ACS Catal. 11, 5554–5571. doi:10.1021/acscatal.1c00748
Yu, X., Wang, L., Chen, M., Fan, X., Zhao, Z., Cheng, K., et al. (2019). Enhanced Activity and Sulfur Resistance for Soot Combustion on Three-Dimensionally Ordered Macroporous-Mesoporous MnxCe1-xOδ/SiO2 Catalysts. Appl. Catal. B: Environ. 254, 246–259. doi:10.1016/j.apcatb.2019.04.097
Yu, X., Zhao, Z., Wei, Y., and Liu, J. (2017). Ordered Micro/macro Porous K-OMS-2/SiO2 Nanocatalysts: Facile Synthesis, Low Cost and High Catalytic Activity for Diesel Soot Combustion. Sci. Rep. 7. doi:10.1038/srep43894
Zhang, H., Han, J., Niu, X., Han, X., Wei, G., and Han, W. (2011). Study of Synthesis and Catalytic Property of WO3/TiO2 Catalysts for NO Reduction at High temperaturesStudy of Synthesis and Catalytic Property of WO3/TiO2 Catalysts for NO Reduction at High Temperatures. J. Mol. Catal. A: Chem. 350, 35–39. doi:10.1016/j.molcata.2011.09.001
Zhang, S., Zhong, Q., Shen, Y., Zhu, L., and Ding, J. (2015a). New Insight into the Promoting Role of Process on the CeO2-WO3/TiO2 Catalyst for NO Reduction with NH3 at Low-Temperature. J. Colloid Interf. Sci. 448, 417–426. doi:10.1016/j.jcis.2015.02.038
Zhang, Y., Guo, W., Wang, L., Song, M., Yang, L., Shen, K., et al. (2015b). Characterization and Activity of V2O5-CeO2/TiO2-ZrO2 Catalysts for NH3-selective Catalytic Reduction of NOx. Chin. J. Catal. 36, 1701–1710. doi:10.1016/s1872-2067(14)60916-0
Zhang, Y., Zhu, X., Shen, K., Xu, H., Sun, K., and Zhou, C. (2012). Influence of Ceria Modification on the Properties of TiO2-ZrO2 Supported V2O5 Catalysts for Selective Catalytic Reduction of NO by NH3. J. Colloid Interf. Sci. 376, 233–238. doi:10.1016/j.jcis.2012.03.001
Keywords: three-dimensional ordered macroporous structure, soot particulates, nitrogen oxides, simultaneous removal, catalysts
Citation: Wang R, Zhong C, Li D, Yu X, Zhao Z, Sojka Z, Kotarba A, Wei Y and Liu J (2022) Preparation of 3DOM ZrTiO4 Support, WxCeMnOδ/3DOM ZrTiO4 Catalysts, and Their Catalytic Performance for the Simultaneous Removal of Soot and NOx. Front. Chem. 10:880884. doi: 10.3389/fchem.2022.880884
Received: 22 February 2022; Accepted: 28 March 2022;
Published: 04 May 2022.
Edited by:
Laura Pastor-Pérez, University of Seville, SpainReviewed by:
Jinlong Wang, Wuhan University of Technology, ChinaCopyright © 2022 Wang, Zhong, Li, Yu, Zhao, Sojka, Kotarba, Wei and Liu. This is an open-access article distributed under the terms of the Creative Commons Attribution License (CC BY). The use, distribution or reproduction in other forums is permitted, provided the original author(s) and the copyright owner(s) are credited and that the original publication in this journal is cited, in accordance with accepted academic practice. No use, distribution or reproduction is permitted which does not comply with these terms.
*Correspondence: Xuehua Yu, eXV4dWVodWExOTg2QDE2My5jb20=; Zhen Zhao, emhlbnpoYW9AY3VwLmVkdS5jbg==
Disclaimer: All claims expressed in this article are solely those of the authors and do not necessarily represent those of their affiliated organizations, or those of the publisher, the editors and the reviewers. Any product that may be evaluated in this article or claim that may be made by its manufacturer is not guaranteed or endorsed by the publisher.
Research integrity at Frontiers
Learn more about the work of our research integrity team to safeguard the quality of each article we publish.