- 1State Key Laboratory of Heavy Oil Processing, College of Chemical Engineering and Environment, China University of Petroleum, Beijing, China
- 2Fushun Research Institute of Petroleum and Petrochemicals, SINOPEC, Fushun, China
Experiments were carried out to research the different contents of Ga2O3 modification effects on the hydrodesulfurization (HDS) performance of 4,6-dimethyldibenzothiophene (4,6-DMDBT) catalyzed by the stepwise impregnation method. Characterization techniques such as XRD, BET, HRTEM, NH3-TPD, and Py-FTIR were performed to determine the effects of each modification of the catalyst by Ga on the properties of the prepared supports and catalysts. The catalytic effect of gallium is reflected in the fact that the empty d-orbitals of Ga elements participate in the formation of molecular orbitals in the active center and change their orbital properties, thus generating a direct desulfurization active phase suitable for complex sulfides for endpoint adsorption. The characterization results indicated that the introduction of Ga2O3 with appropriate content (2 wt.%) promoted Ni and Mo species to disperse uniformly and doping of more Ni atoms into the MoS2 crystals, which also increased the average stacking number and the length of MoS2. As a result, more NiMoS active phases were favored to form in the system. The specific surface area and the amounts of acid sites were increased, facilitating the adsorption of reactant molecules and the HDS reactions. The HDS results also suggested the effects of Ga modification play a very important role in the catalytic performance of the corresponding catalysts. The catalyst Ga–Ni–Mo/Al2O3 exhibited the highest conversion rate towards 4,6-DMDBT HDS when the amount of Ga2O3 loading was 2 wt.% with an LHSV of 2.5 h−1 at 290°C and Ga modification also can effectively improve the direct desulfurization (DDS) route selectivity in varying degrees.
Introduction
With the increase in global environmental pollution and environmental laws and regulations in various countries becoming stricter, exhaust from the combustion of sulfide in automotive diesel has become one of the important sources of pollution (Wang et al., 2017; Liu et al., 2018; Weng et al., 2020; Zeng et al., 2021). The Environmental Protection Agency (EPA) and E.U. stipulate that the sulfur content should not exceed 10 and 15 ppm (Kulkarni and Afonso, 2010; Liu et al., 2020; Guo et al., 2021). Therefore, low sulfurization of diesel fuel and achieving deep desulfurization of diesel fuel have become a key issue in hydrodesulfurization (Humadi et al., 2021). At present, diesel desulfurization technologies being investigated at home and abroad include adsorptive desulfurization (ADS), oxidative desulfurization (ODS), biodesulfurization (BDS), and hydrodesulfurization (HDS). ODS is a technology for oxidizing heavy sulfides by adding one or two oxygen atoms to sulfur using a suitable oxidant at low temperature and pressure; however, the chosen oxidant is not always selective, and the selective solvent for the extraction of sulfur compounds is not necessarily suitable either (Ali et al., 2006). The basic principle of ADS is to use adsorbents to adsorb sulfur compounds in diesel oil so as to remove sulfides from diesel (Selvavathi et al., 2009). However, adsorption desulfurization is difficult to regenerate, and most adsorbents are not highly selective for sulfides such as 4,6-DMDBT, which are difficult to hydrotreat (Jayaraman et al., 2004). BDS is a new technology for the removal of bound sulfur from sulfur-containing heterocyclic compounds in petroleum using aerobic and anaerobic bacteria at atmospheric pressure and temperature, with promising applications (Mohebali and Ball, 2016). Nevertheless, the desulfurization rates of biocatalysts and the ability of organic sulfides limit their large-scale commercialization (Monticello, 2001). Based on the challenges of the abovementioned three technologies, HDS remains the most widely used technology in the world, which is a heterogeneous and conventional hydrogenation reaction (Chandra Srivastava, 2012). Compared with the former three, it has longer catalyst lifetime and stronger catalyst adaptability to the feed and has the advantage of a higher desulfurization rate for HDS (Huang et al., 2018; Weng et al., 2020). However, HDS cannot effectively remove low-reactive sulfur compounds, such as dibenzothiophene (DBT) and its derivatives, especially 4,6-DMDBT. In order to effectively remove 4,6-DMDBT, two pathways, direct desulfurization (DDS) and hydrodesulfurization (HYD), have been studied by many scholars (Gates and Topsøe, 1997; Li et al., 2019). Studies have shown that unsubstituted 4,6-DMDBT HDS reaction is more dependent on the DDS path, where it not only reacts faster but also results in desulfurization under the premise of ensuring that the aromatic rings do not increase. However, it is limited by its steric hindrance of the substituents (Yin et al., 2013; Wang et al., 2020). In order to design and prepare a highly active hydrodesulfurization catalyst, Okamoto and Kubota (2003) synthesized SiO2-, TiO2-, ZrO2-, and Al2O3-supported catalysts using the CVD technique and found that the activity of hydrodesulfurization is positively correlated with the amount of CoMoS phases. Wagenhofer et al. (2020) thought that unsupported Ni–Mo sulfides react more rapidly than Al2O3-supported catalysts on the rate of hydrodesulfurization. Naboulsi et al. (2017) considered that the DDS route is a mainly hydrodesulfurization route than HYD when dual mesoporous titania is used as a support. Due to the high OH concentration on the surface of the support, an inherent Brönsted acid center is formed that is conducive to direct desulfurization through isomerization and disproportionation reactions. In addition, the exploration of support modification has not stopped, and composite supports such as SiO2–TiO2 (Gallegos–Hernández et al., 2020), SiO2–Al2O3 (Xu et al., 2017), ZrO2–Al2O3 (Baston et al., 2015; Díaz–García et al., 2017), and MgO2–Al2O3 (Rana et al., 2007; Guevara-Lara et al., 2010; Vázquez–Garrido et al., 2019) are still a hot topic for the majority of scholars. However, due to its low cost, easy industrialization, and high surface area, as well as excellent thermal, mechanical, and chemical stability, γ-Al2O3 is still the most widely used carrier for hydrodesulfurization catalysts (Egorova, 2004; Wang et al., 2016).
It has been proposed that most hydrotreating reactions take place at the MoS2 edge and HDS has been found to occur at the corner sites (Abrams et al., 1996; Eijsbouts, 1997; Kasztelan et al., 1984; Kasztelan, 1990). Schuit and Gates (1973) believed that the S-atom in the upper layer is chemically bonded to the Mo-atom in the immediate lower layer. When the Mo atoms are reduced from Mo5+ to Mo3+, the S atoms move out of the surface, thus forming active sites. The cofactor Ni enters into the surface structure of the alumina carrier and induces the formation of a tetrahedral structure of the secondary Al atoms. Each S atom is bonded to two Mo atoms, and when the S atoms are removed, the S hole formed releases the two Mo atoms and causes one of them to form an adsorption site. The performance of the catalyst is directly related to the number of active sites with high catalytic performance. For the NiMoS active phase, Ni mainly contributes to the associated generation of sulfhydryl (SH) groups and their selective incorporation at the MoS2 slab edge (Copéret, 2013; Schüth, 2009). When metal elements with suitable electronic structures are introduced into Ni–Mo/Al2O3 bimetallic catalysts, the active phase of Ni–Mo–S is affected and the concentration of active centers is increased; thus, the activity of the catalyst for hydrodesulfurization reaction is enhanced (Manoli et al., 2004; Varga et al., 2017). Ferdous et al. (2005) suggested the increased activity is related to the decrease of average slab length and the increase of MoS2 edge along with angular atom dispersion. More importantly, it can promote the formation of more type II NiMoS active phases (Zhou et al., 2017; Zhou et al., 2018; Tanimu and Alhooshani, 2019). It is generally believed that there is a great correlation between the numbers of type II NiMoS active phases and the catalytic activity of the catalyst (Chen et al., 2013). Research shows that the metal–support interaction (MSI) is crucial for electron transfer between the metal and support when the catalysts support multiple metals (Ning et al., 2017). To explore the effect of metal additives on the activity of HDS, a large number of polymetallic catalysts supported on Γ-Al2O3 have been investigated, such as Fe (Liu et al., 2021), Zn, Ru, and Ir (Niquillerothlisberger and Prins, 2006), and even noble metal Pt (Liu et al., 2021) and its alloys Pt–Pd (Niquillerothlisberger and Prins, 2006). Pt is favored by scholars over other metals because it has more advantages in providing active hydrogen species, giving the catalyst superior hydrogenation performance. However, the sulfur resistance of Pt-based catalysts is still a major problem that plagues practical applications and needs to be enhanced. In recent years, the addition of Ga as a metal additive for the synthesis of HDS catalysts has started to attract attention due to the advantage of circumventing the high cost of precious metals (Altamirano et al., 2005). Gallium ions not only have a high affinity for tetrahedral sites of alumina but also change the ratio of tetrahedral to octahedral species of Ni (Co) that can participate in the MoS2 decoration (Cimino et al., 1975; Jacono et al., 1977). Moreover, people have found that the modification of gallium on the surface will not only change the morphology of the MoS2 slab promoted by active metal Ni but also enhance the vulcanization of Mo species (Zhou et al., 2017). Altamirano et al. (2005) observed the addition of Ga increased the activity of the NiMo catalyst and affected the reaction rate of the HYD route and DDS route in different degrees. Ga has the outer electron arrangement of 4s24p1 and has an outer orbit similar to that of Ni (3d84s2) and Mo (4d55s1). As a result, the empty orbitals participate in the formation of active center molecular orbitals and modify the morphology of Ni–Mo–S active phases (Altamirano-Sanchez et al., 2008; Zepeda and Pawelec, 2012; Zhou et al., 2018). Nevertheless, for HDS activity, there is no clear and reasonable explanation about the additional amount of metal content and reaction conditions.
Considering that in this context, a series of catalysts with the Ga content ranging from 2 to 6 wt.% were prepared by the stepwise impregnation method. A succession of characterizations (XRD, BET, TEM, NH3-TPD, and Py-FTIR) were carried out to investigate the effect of Ga loading on the physical and chemical properties of the Ni–Mo/γ-Al2O3 catalyst. The hydrodesulfurization reaction was evaluated using 4,6-DMDBT as the probe molecule, and the effects of Ga loading and reaction conditions (temperature and liquid hourly space velocities) on the hydrodesulfurization conversion and DDS selectivity of 4,6-DMDBT were examined.
Experimental
Preparation of the Supports
First, γ-Al2O3 was prepared by the strip extrusion method with the following process conditions: pseudo-boehmite (Shandong Aluminum Corporation) and deionized water were mixed thoroughly at a mass ratio of 1:1, and then, 2 wt.% sesbania powder (Shandong Xunda Chemical Group Co., Ltd.) and 5 wt.% nitric acid (HNO3, Aladdin, 65%) were added. The mixture was extruded by using an extruding machine at 30 MPa pressure, and the extruded strips were shaped into a clover type with a diameter of 1.5 mm. The supports was naturally dried in a place of protection from light and ventilation for 24 h and then dried in a drying oven at 120°C for 12 h, and finally, the required γ-Al2O3 supports were obtained in a muffle furnace at a heating rate of 2°C min−1, kept at 500°C for 4.0 h, and naturally cooled to room temperature. The obtained supports were sieved into particles with the size of 20–40 meshes.
Preparation of the Ni–Mo/γ-Al2O3 Catalysts
The incipient wetness co-impregnation method was used to synthesize Ni–Mo/γ-Al2O3 catalysts, and the active metal loading was MoO3:16 wt.% and NiO:4 wt.%, respectively. The specific preparation process is as follows: a certain mass of ammonium heptamolybdate tetrahydrate [(NH4)6Mo7O24⋅4H2O, Aladdin, ≥99.8%] was added to an appropriate amount of deionized water and ammonia to dissolve it completely, and then, nickel nitrate hexahydrate [Ni(NO3)2⋅6H2O, Aladdin, ≥99.8%] solution was added and stirred uniformly to obtain the nickel–molybdenum co-impregnated solution. The prepared Ni–Mo co-impregnation solution was evenly added dropwise to the alumina surface, and the samples were naturally dried at room temperature for 24 h, then dried in an oven for 6 h, and finally, heated to 500°C at a rate of 2°C min−1 in a muffle furnace kept at a constant temperature for 4.0 h.
Preparation of the Ga-Modified Ni–Mo/γ-Al2O3 Series Catalysts
A series of Ga-modified Ni–Mo/γ-Al2O3 catalysts were prepared using the stepwise impregnation method, in which the Ga2O3 loading amount was 2 wt.%, 4 wt.%, and 6 wt.%, respectively, and the specific steps were as follows: gallium nitrate (Ga(NO3)3⋅H2O, Aladdin, ≥99.5%) was dissolved in the deionized water, and then, the solution was loaded using incipient wetness impregnation on the Ni–Mo/Al2O3. The sample was dried in air for 24 h and then dried in an oven at 120°C for 4 h. Finally, it was heated to 500°C at 2°C min−1 in a muffle furnace, with a constant temperature for 4.0 h. The obtained catalysts were denoted as SCal (2 wt.% Ga2O3), Scam (4 wt.% Ga2O3), and SCah (6 wt.% Ga2O3) depending on Ga2O3 content from low to high.
Material Characterization
All catalyst samples synthesized were characterized on a PANalytical advanced powder diffractometer using Cu Kα radiation with an accelerating voltage of 40 kV and current of 40 mA in the 2θ interval of 5–80 °via a scanning rate of 0.2 °s−1, by which the patterns of X-ray diffraction (XRD) were recorded. The surface areas of the tested samples were carefully calculated by using the Brunauer–Emmett–Teller (BET) equation in the relative pressure (P/P0) range of 0.05–0.30. The pore diameter distributions and pore volumes of all the investigated samples were calculated using the Barrett–Joyner–Halenda (BJH) method from the N2 adsorption isotherms. The catalysts were sulfided in a JQ-III fixed-bed microreactor with 2 wt.% CS2 cyclohexane solution at 320°C for 4 h. Afterward, the investigated catalysts were taken on a Philips Tecnai G2 F20 instrument with an acceleration voltage of 200 keV to obtain the images of active phases via a high-resolution transition electron microscope (HRTEM). To analyze the results of the average slab length and average stacking number of MoS2, no less than 300 MoS2 slabs were counted using the equations reported elsewhere (Ortega–Domínguez et al., 2015; W. Zhou et al., 2017).
where li is the length of the slab, ni is the number of slabs with length li, and Ni is the number of layers in slab i.
The acidity of the catalysts was assessed by NH3 temperature programmed desorption (NH3-TPD), which was performed on an Auto Chem II 2920 automatic chemical adsorption instrument. All catalysts were pretreated in an Ar and air mixture (v:v = 3:1) at 773k for 60 min and then cooled to 373 k in Ar flow and adsorbed NH3 for 40 min. Then, the physically adsorbed NH3 was removed in a continuous Ar flow for 90 min. The reactor temperature was then programmed to increase with a heating rate of 10°Cmin−1 for 65 min, and the amount of desorbed NH3 was detected by using a continuous effluent gas monitor with a thermal conductivity detector (TCD). After the IR spectra were recorded on a Magna 560 FT-IR analyzer, pyridine-adsorbed Fourier transform infrared measurements (Py-FTIR) was used to assess the acidity properties of all the samples by using pyridine as a probe molecule. Firstly, the sample was dehydrated for 3 h at 623 K under 10−2 Pa vacuum. Second, to obtain the saturated adsorption of the Py-FTIR spectrum, pure pyridine vapor was added to the measured samples at room temperature for 30 min. Finally, the adsorbed pyridine was evacuated, respectively, at 523k and 623k for an hour so as to obtain the desorbed Py-FTIR spectra.
Catalyst Assessment
4,6-DMDBT was used as a probe to assess the HDS performances of SCal, Scam, and SCah catalysts. On the fixed-bed reactor whose length was 200 mm and inner diameter was 8 mm, 2.0 g of catalyst was loaded with a particle size of 20–40 meshes. In the first place, the pre-sulfidation of tested catalysts was carried out with 2.2 wt.% CS2 cyclohexane solution at 330°C and 4 MPa with H2/oil (v/v) of 60 for 4 h. Then, the reactor temperature was allowed to drop to the temperature required for the reaction at the previous condition. Stabilization of the 4,6-DMDBT cyclohexane solution at a mass concentration of 1.0% was carried out for 4 h at 4.0 MPa and 270°C, 280, and 290°C, respectively. The H2/oil (v/v) was maintained at 120 when all catalysts were evaluated. The collected products of 4,6-DMDBT was determined by the GC–MS technique on an Agilent 4890D gas chromatograph equipped with a 60 m capillary Rtx-1 column (0.25 mm, RESREK). The column temperature was increased from 50 to 300°C at a rate of 15°C min−1, while the N2 pressure was maintained at 0.3 MPa and the flow rate was 30 ml min−1. The 4,6-DMDBT conversions and DDS route selectivity (3,3′-DMBP selectivity) were counted using the following equations, respectively:
where m4,6-DMDBT is the molar fraction of remaining 4,6-DMDBT and m3,3′-DMBP is the molar fraction of 3,3′-DMBP tested in the liquid products.
Results and Discussion
Effect of Ga Modification on Crystalline Structure
The spectra of Ni–Mo/Al2O3 modified by the stepwise impregnation method with different Ga loadings determined by powder X-ray diffraction are shown in Figure 1. It can be seen from the diagrams the characteristic diffraction peaks (4.4.0), (4.0.0), and (3.1.1) of γ-Al2O3 are almost unchanged, which shows that the crystal structure of γ-Al2O3 is not affected. No characteristic peaks of Ga2O3 and other Ga compounds are found in Figure 1, which indicates that Ga2O3 was highly dispersed on Ni–Mo/Al2O3 and loaded well. However, diffraction peaks (0.2.2), (-3.1.2), and (2.2.0) of NiMoO4 were observed, illustrating that the introduction of Ga2O3 brought about the agglomeration of Ni and Mo elements. In addition, the characteristic peak intensity of NiMoO4 increased with the increase in the content of Ga2O3, which manifests that the introduction of excessive Ga2O3 is not conducive to the dispersion of Ni and Mo elements.
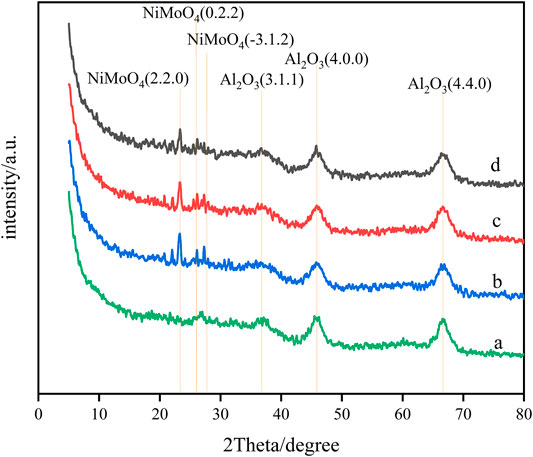
FIGURE 1. XRD patterns of impregnated Ga-modified Ni–Mo/Al2O3: (a) Ni–Mo/Al2O3, (b) SGal, (c) SGam, and (d) SGah.
Effect of Ga Modification on Pore Structure
The method of N2 physical adsorption–desorption was carried out to confirm the size of the specific surface area, average pore diameter, and pore volume of Ni–Mo/Al2O3, SGal, SGam, and SGah. The abovementioned textural properties are calculated and displayed about the four synthesized samples in Table 1, and the results of pore size distribution for two samples are recorded in Figure 2. From the data in Table 1, it is clearly found, especially for SGam, that the specific surface area increased from 214 m2 g−1 to 227 m2 g−1with the addition of Ga2O3 in comparison with Ni–Mo/Al2O3, indicating that the introduced Ga2O3 had a wide distribution in the inner and outer surface of the catalyst; however, the pore volume and pore size decreased to some extent because parts of Ga2O3 blocked the pore channel of alumina. It can be seen from Figure 2 that the pore size distribution curve moved to the direction of small pores, and the peak area decreases slightly, which also proved that a large number of pores were blocked or even disappeared with the introduction of gallium.
Effect of Ga Modification on Morphological Characteristics
In order to observe the morphologies, characteristics, and dispersion about the sulfide active phase of Ni–Mo/Al2O3 and SGax (x stands for l, m, and h) catalysts, the images obtained by HRTEM characterization are shown in Figure 3. At least, 300 MoS2 slabs of each catalyst were counted to statistically analyze the results of the average slab length and average stacking number of the active-phase slabs in Table 2. It can be clearly seen from Table 2 that the introduction of Ga contributed to an increase in the average stacking number of MoS2 and its change rule kept pace with the increase in Ga loading. Especially, the largest increase of average stacking number for SGal is from 1.1 to 3.6 layers for Ni–Mo/Al2O3, which displays that the introduction of Ga weakened the Mo–O–Al bond energy and caused a weaker interaction between MoS2 and the support, resulting in the formation of more type II Ni–Mo–S reactive phases with high stacking layers. In accordance with the abovementioned regulation, the variation of the average length also has a positive correlation with the Ga2O3 content. Also, the degrees of average length increase in the following order: SGal (2.4 nm) < Ni–Mo/Al2O3 (2.7 nm) <SGam (3.1 nm) <SGal (3.3 nm). The average length slightly increased from 2.7 nm for sample Ni–Mo/Al2O3 to 3.3 nm for sample SGah. This can also be explained by the abovementioned data.
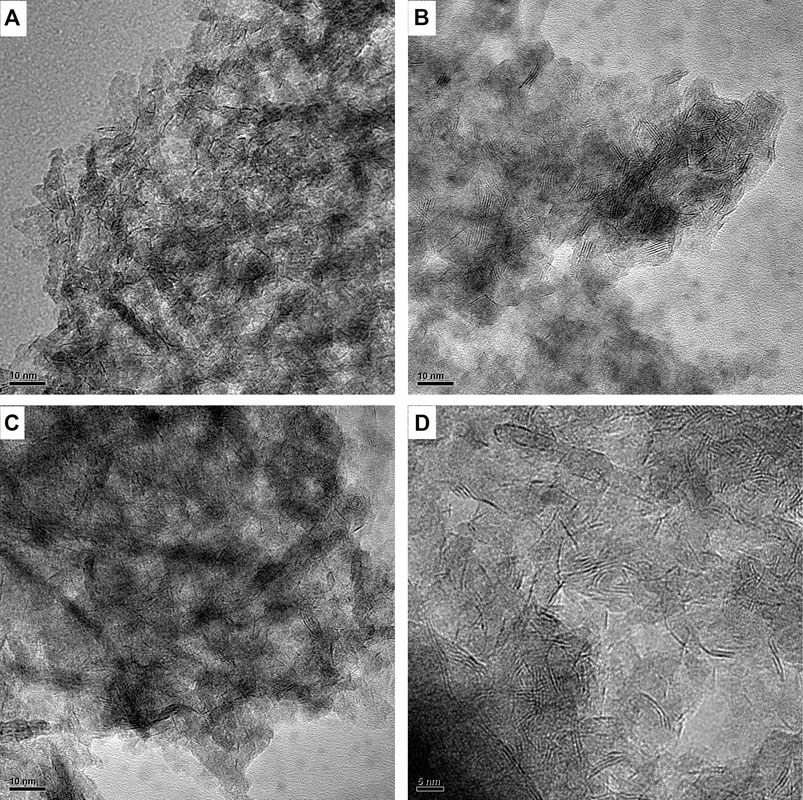
FIGURE 3. HRTEM of sulfide-impregnated Ga-modified Ni–Mo/Al2O3: (A) Ni–Mo/Al2O3, (B) SGal, (C) SGam, and (D) SGah.
Effect of Ga Modification on Acidity Properties
Acidity plays a crucial role in the formation of the active phase and the HDS reaction, so NH3-TPD characterization of the series samples was carried out and is displayed in Figure 4. The peak temperature and peak area of the NH3 desorption were calculated for each of the investigated samples and are summarized in Table 3. The NH3-TPD profiles in Figure 4 reveal the peak temperature of the Ga–Ni–Mo/Al2O3 catalyst existed mainly between 150 and 300°C, indicating that the catalyst was mainly the prince of weak acid sites and medium–strong acid sites, which was weaker than that of the Ni–Mo/Al2O3 catalyst. It can be seen from Table 3 that the introduction of Ga made the NH3 desorption peak shift to a lower temperature, manifesting that the weak acid sites of the catalyst mainly increased. According to the comparison of peak area, the number of acid sites of the Ni–Mo/Al2O3 catalyst was increased by introducing Ga2O3, but it was negatively correlated with introduced Ga2O3 content. Thus, the acid sites varied significantly for SGal. That phenomenon explained that the acid sites were covered by Ga2O3, leading to reduction in the degree of increase on the number of acid sites.
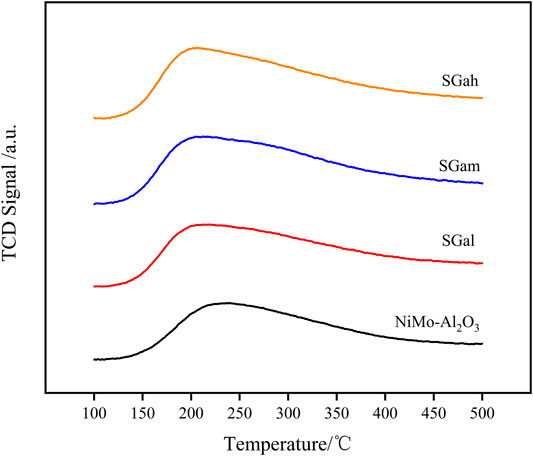
FIGURE 4. NH3-TPD patterns of impregnated Ga-modified Ni–Mo/Al2O3: (a) Ni–Mo/Al2O3, (b) SGal, (c) SGam, (d) SGah.
Effect of Ga Modification on Acid Types and Strength
It is well known that acid types and strength are the key points to decide the performance of the corresponding catalysts. As a consequence, pyridine desorption FTIR analyses were performed at different temperatures, and the results are listed in Table 4, where the specific data of weak and strong Brönsted acid sites (BAS) or Lewis acid sites (LAS) were derived from the information of pyridine desorption at 200 and 350°C, respectively. It can be clearly observed from Table 4 that the introduction of 2 wt.% Ga2O3 enhanced the weak B-acid, and the B-acid enhancement facilitates the desulfurization of 4,6-DMDBT through the isomeric desulfurization pathway (ISOM). Moreover, after the isomerization of the methyl group on 4,6-DMDBT, the steric hindrance to sulfur atoms decreased significantly, which provided access to direct desulphurization just by hydrogenolysis of sulfur atoms, and the path to remove sulfur was considered to be very ideal. In addition, the introduction of different contents of Ga increased the Lewis acid, among which the weak Lewis acid increased more and the strong Brönsted acid decreased slightly. This is consistent with the characterization of NH3-TPD. Except for the enhancement of weak Brönsted acid by SGam, the amount of Ga2O3 introduced had little effect on the acid type and amount of Brönsted acid and Lewis acid of Ni–Mo/Al2O3, but the amount of Ga2O3 introduced increased the total acid amount.
Assessment of Catalytic Activities
As is concretely confirmed, though the appropriate content of Ga2O3 modification can effectively improve the physicochemical properties of the catalysts from the characterization results mentioned above, the favorable conditions for conversion and selectivity at different temperatures need to be explored. Thus, 4,6-DMDBT served as the probe molecules in the assessment of the HDS performance of Ni–Mo/Al2O3 and SGax series catalysts on a fixed-bed reactor under a total pressure of 4 MPa and the liquid hourly space velocities (LHSVs) of 2.5 h−1 at different reaction temperatures (in the range of 270–290°C). The variation of conversions on 4,6-DMDBT at different temperatures is clearly displayed in Figure 5A, suggesting that the activities of all the investigated catalysts increased with the increase in the reaction temperature. These results also pointed out that there was no significant disparity in the conversion of SGax series catalysts with different Ga2O3 loadings, and the highest conversion rate was obtained when the Ga2O3 loading was 2 wt.%. In addition, with the increase in reaction temperature, the difference of conversion rate between SGax series catalysts and the Ni–Mo/Al2O3 catalyst became smaller, which resulted from the increase in the conversion rate of 4,6-DMDBT at high temperature close to the reaction end point.
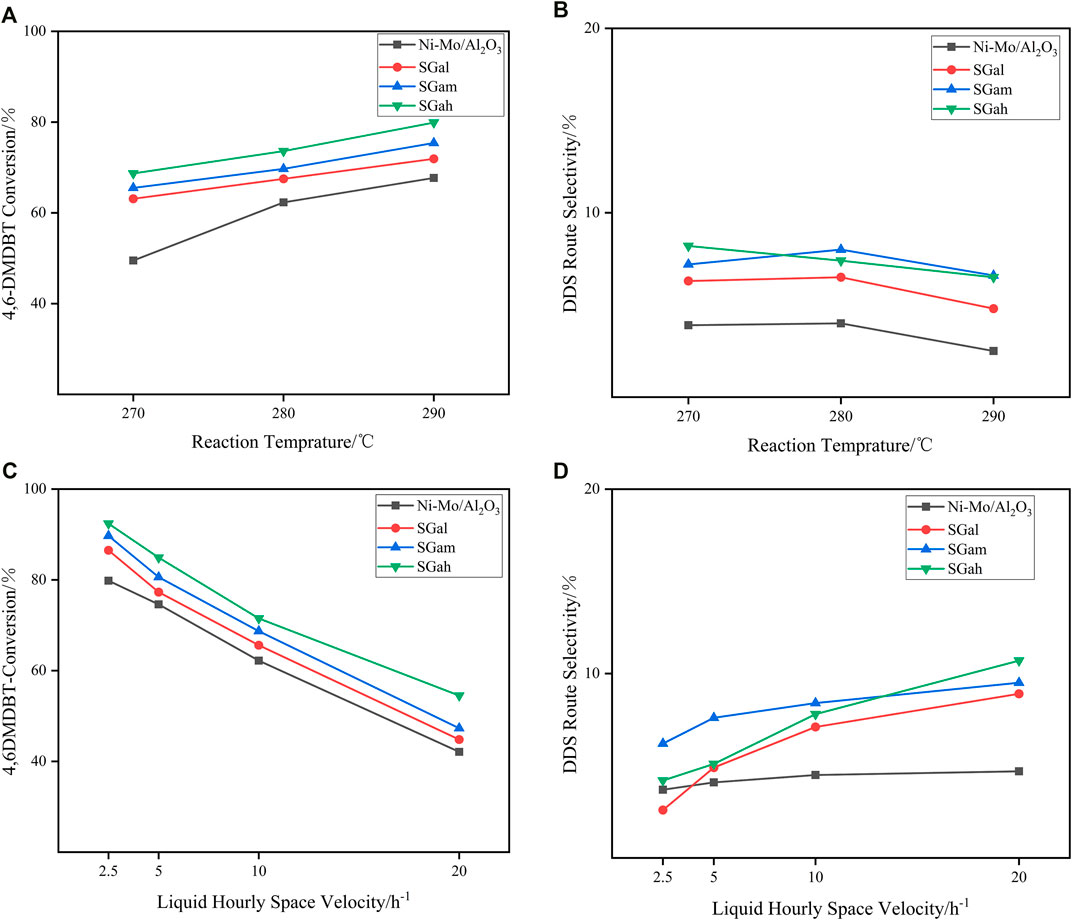
FIGURE 5. Catalytic performance of impregnated Ga-modified Ni–Mo/Al2O3: (A) 4,6-DMDBT conversion on different reaction temperatures, (B) DDS selectivity of 4,6-DMDBT on different reaction temperatures, (C) 4,6-DMDBT conversion on different LHSVs at 280°C, and (D) DDS selectivity of 4,6-DMDBT conversion on different LHSVs at 280°C.
Scheme 1 shows the HDY and DDS reaction route over Ga–Ni–Mo/Al2O3 catalysts separately. HYD pathway desulfurization is a tedious step to form the products 3,3′-dimethylcyclohexanebenzene (3,3′-DMCHB) or 3,3′-dimethylbicyclohexyl (3,3′-DMBCH), while DDS pathway desulfurization is achieved with fewer steps and without excess aromatic rings and produces 3,3′-dimethylbiphenyl (3,3′-DMBP). The DDS route selectivity over different catalysts at the same conditions mentioned above was obtained, and the results are shown in Figure 5B. It can be observed from Figure 5B that, with the increase in reaction temperature, the DDS selectivity of the tested catalysts on the DMDBT decreased, owing to that Ni gradually began to be reduced as the reaction temperature exceeded 290°C. Especially, the metal Ni has a strong hydrogenation performance. The reason mentioned above led to the enhancement of the HYD path. In addition, it was also found that if the reaction temperature is higher than 290°C, the high hydrogenation performance of Ni can even convert a small amount of DDS products into HYD products, but it was generally considered that this reaction will not occur, which was the unique characteristic of Ni-containing hydrotreating catalysts. Further increase in temperature would lead to a profound increase in the proportion of hydrogenation conversion of DDS products to HYD products. In order to properly deal with this situation, the metal promoter can be replaced with the load of Co with low hydrogenation performance, and the amount of the Ni loading or the Ni/Mo atomic ratio ought to be reduced.
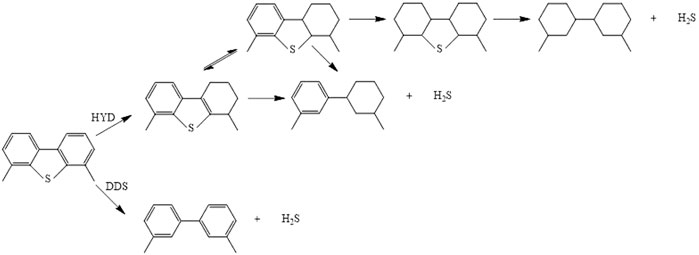
SCHEME 1. Reaction scheme of HDS for 4,6-DMDBT over Ga–Ni–Mo/Al2O3 catalysts. HYD, hydrogenation. DDS, direct desulfurization.
The influence of different LHSVs in the investigated series catalysts on the 4,6-DMDBT HDS reaction is shown in Figure 5C. As can be clearly noticed from the figure, with the decrease in LHSVs which meant increasing the residence time of raw materials, the conversion rate of 4,6-DMDBT increased gradually and over the tested catalysts was in the order of Ni–Mo/Al2O3<SGah < SGam < SGah. When the LHSV decreased gradually, the increasing rate of conversion rates slowed down gradually as the reaction is close to complete conversion. Thus, the conversion rate gap between different Ga2O3 loading catalysts was bridged.
Figure 5D reveals the transformation about selectivity of DDS route by stepwise impregnation of the Ga–Ni–Mo/Al2O3 catalyst with different LHSVs on 4,6-DMDBT at a reaction temperature of 280°C. As it is vividly shown in Figure 5D, the gallium-modified catalysts exhibited higher DDS route selectivity than the Ni–Mo/Al2O3 catalyst at high LHSV. However, the DDS route selectivity of SGax series catalysts for 4,6-DMDBT exhibited a negative correlation with the variation of LHSV. With the decrease in LHSV, the DDS selectivity of SGax series catalysts for 4,6-DMDBT even decreased to about the corresponding selectivity of the Ni–Mo/Al2O3 catalyst. On the one hand, the incorporation of Ga improved the average stacking number of the active-phase slabs and generated more type II NiMoS active phases which weekly interact with the support or do not interact with the support at all, and it is considered to be very active (Nikulshin et al., 2014; van Haandel et al., 2015), so that the HYD route got strengthened. On the other hand, the residence time of raw materials became longer while the LHSV decreased, which is beneficial to the HYD route with longer reaction path and slower reaction rate. In addition, because of the strong hydrogenation ability of the reduced metal Ni, a small part of DDS products was also transformed into HYD products. All mentioned above were identified as the reason for which the variation of LHSV had impact on the DDS route selectivity.
Conclusion
The experiments showed that the introduction of Ga2O3 with appropriate content (2 wt.%) promoted not only Ni and Mo species to disperse uniformly but also doping of more Ni atoms into the MoS2 crystals. In addition, the average stacking number and the length of Mo2S were increased. Those mentioned above resulted in the formation of more NiMoS active phases. The specific surface area and the amount of acid sites were increased, facilitating the adsorption of reactant molecules and the hydrodesulfurization reactions. Last but not the least, the catalyst Ga–Ni–Mo/Al2O3 exhibited the highest conversion rate towards 4,6-DMDBT HDS when the amount of Ga2O3 loading was 2 wt.% with an LHSV of 2.5 h−1 at 290°C, and Ga modification also can effectively improve the DDS route selectivity in varying degrees.
Data Availability Statement
The original contributions presented in the study are included in the article/Supplementary Material, further inquiries can be directed to the corresponding author.
Author Contributions
MH designed experimental plans, performed the main experimental work, analyzed and discussed the results and wrote the manuscript. WH assisted in the design of the scheme and performed the main experimental work. AL participated in manuscript writing. QW proposed the themes, ideas, and content of the research work. YJ, HY, ZY, XW and ZX assisted in literature review and manuscript sorting. YZ guided experimental work and manuscript revision.
Funding
This work was supported by the National Natural Science Foundation of China under grant No. 22078360.
Conflict of Interest
Author AL was employed by SINOPEC.
The remaining authors declare that the research was conducted in the absence of any commercial or financial relationships that could be construed as a potential conflict of interest.
Publisher’s Note
All claims expressed in this article are solely those of the authors and do not necessarily represent those of their affiliated organizations, or those of the publisher, the editors, and the reviewers. Any product that may be evaluated in this article, or claim that may be made by its manufacturer, is not guaranteed or endorsed by the publisher.
Supplementary Material
The Supplementary Material for this article can be found online at: https://www.frontiersin.org/articles/10.3389/fchem.2022.865375/full#supplementary-material
References
Ali, M., Almalki, A., Elali, B., Martinie, G., and Siddiqui, M. (2006). Deep Desulphurization of Gasoline and Diesel Fuels Using Non-hydrogen Consuming Techniques. Fuel 85 (10-11), 1354–1363. doi:10.1016/j.fuel.2005.12.006
Altamirano, E., De los Reyes, J. A., Murrieta, F., and Vrinat, M. (2008). Hydrodesulfurization of 4,6-dimethyldibenzothiophene over Co(Ni)MoS2 Catalysts Supported on Alumina: Effect of Gallium as an Additive. Catal. Today 133-135, 292–298. doi:10.1016/j.cattod.2007.12.085
Altamirano, E., Delosreyes, J., Murrieta, F., and Vrinat, M. (2005). Hydrodesulfurization of Dibenzothiophene and 4,6-Dimethyl-Dibenzothiophene: Gallium Effect over NiMo/AlO Sulfided Catalysts. J. Catal. 235 (2), 403–412. doi:10.1016/j.jcat.2005.09.011
Baston, E. P., França, A. B., Neto, A. V. d. S., and Urquieta-González, E. A. (2015). Incorporation of the Precursors of Mo and Ni Oxides Directly into the Reaction Mixture of Sol–Gel Prepared γ-Al2O3-ZrO2 Supports – Evaluation of the Sulfided Catalysts in the Thiophene Hydrodesulfurization. Catal. Today 246, 184–190. doi:10.1016/j.cattod.2014.10.035
Chandra Srivastava, V. (2012). An Evaluation of Desulfurization Technologies for Sulfur Removal from Liquid Fuels. RSC Adv. 2 (3), 759–783. doi:10.1039/C1RA00309G
Chen, W., Maugé, F., van Gestel, J., Nie, H., Li, D., and Long, X. (2013). Effect of Modification of the Alumina Acidity on the Properties of Supported Mo and CoMo Sulfide Catalysts. J. Catal. 304, 47–62. doi:10.1016/j.jcat.2013.03.004
Cimino, A., Jacono, M., and Schiavello, M. (1975). Effect of Zinc, Gallium, and Germanium Ions on the Structural and Magnetic Properties of Nickel Ions Supported on Alumina. J. Phys. Chem. 79. doi:10.1021/j100570a010
Copéret, C. (2013). Catalysis by transition metal sulphides. From molecular theory to industrial applications. H. Toulhoat and P. Raybaud, Editors, Technip Edition, Paris, 2013. Journal of Catalysis 307, 121. doi:10.1016/j.jcat.2013.06.011
Díaz-García, L., Santes, V., Viveros-García, T., Sánchez-Trujillo, A., Ramírez-Salgado, J., Ornelas, C., et al. (2017). Electronic Binding of Sulfur Sites into Al2O3-ZrO2 Supports for NiMoS Configuration and Their Application for Hydrodesulfurization. Catal. Today 282, 230–239. doi:10.1016/j.cattod.2016.08.001
Egorova, M. (2004). Hydrodesulfurization of Dibenzothiophene and 4,6-dimethyldibenzothiophene over Sulfided NiMo/?-Al2O3, CoMo/?-Al2O3, and Mo/?-Al2O3 Catalysts. J. Catal. 225 (2), 417–427. doi:10.1016/j.jcat.2004.05.002
Eijsbouts, S. (1997). On the Flexibility of the Active Phase in Hydrotreating Catalysts. Appl. Catal. A: Gen. 158, 53–92. doi:10.1016/S0926-860X(97)00035-5
Ferdous, D., Dalai, A. K., Adjaye, J., and Kotlyar, L. (2005). Surface Morphology of NiMo/Al2O3 Catalysts Incorporated with boron and Phosphorus: Experimental and Simulation. Appl. Catal. A: Gen. 294 (1), 80–91. doi:10.1016/j.apcata.2005.07.025
Gallegos-Hernández, A. Y., Martínez-Rosales, M., Rico, J. L., and Avalos-Borja, M. (2020). Improvement in the Hydrodesulfurization of Dibenzothiophene over Supported NiMoW Catalysts. Reac Kinet Mech. Cat 132 (1), 317–330. doi:10.1007/s11144-020-01909-2
Gates, B. C., and Topsøe, H. (1997). Reactivities in Deep Catalytic Hydrodesulfurization: Challenges, Opportunities, and the Importance of 4-Methyldibenzothiophene and 4,6-Dimethyldibenzothiophene. Polyhedron 16, 3213–3217. doi:10.1016/S0277-5387(97)00074-0
Guevara-Lara, A., Cruz-Pérez, A. E., Contreras-Valdez, Z., Mogica-Betancourt, J., Alvarez-Hernández, A., and Vrinat, M. (2010). Effect of Ni Promoter in the Oxide Precursors of MoS2/MgO–Al2O3 Catalysts Tested in Dibenzothiophene Hydrodesulphurization. Catal. Today 149 (3-4), 288–294. doi:10.1016/j.cattod.2009.09.014
Guo, D., Liu, Y., Ji, H., Chen, B., Shen, C., Li, F., et al. (2021). Silicate-Enhanced Heterogeneous Flow-Through Electro-Fenton System Using Iron Oxides under Nanoconfinement. Environ. Sci. Tech. 55, 4045-4053. doi:10.1021/acs.est.1c00349
Huang, T., Xu, J., and Fan, Y. (2018). Effects of Concentration and Microstructure of Active Phases on the Selective Hydrodesulfurization Performance of Sulfided CoMo/Al2O3 Catalysts. Appl. Catal. B: Environ. 220, 42–56. doi:10.1016/j.apcatb.2017.08.029
Humadi, J. I., Gheni, S. A., Ahmed, S. M. R., Abdullah, G. H., Phan, A. N., and Harvey, A. P. (2021). Fast, Non-extractive, and Ultradeep Desulfurization of Diesel in an Oscillatory Baffled Reactor. Process Saf. Environ. Prot. 152, 178–187. doi:10.1016/j.psep.2021.05.028
Jacono, M., Schiavello, M., Beer, V., and Minelli, G. (1977). Effect of Gallium Ions and of Preparation Methods on the Structural Properties of Cobalt-Molybdenum-Alumina Catalysts. J. Phys. Chem. 81. doi:10.1002/chin.19774702910.1021/j100531a014
Jayaraman, A., Hernandez-Maldonado, A. J., Yang, R. T., Chinn, D., Munson, C. L., and Mohr, D. H. (2004). Clinoptilolites for Nitrogen/methane Separation. Chem. Eng. Sci. 59 (12), 2407–2417. doi:10.1016/j.ces.2003.10.030
Kasztelan, S. (1990). A Descriptive Model of Surface Sites on Molybdenum(tungsten) Disulfide Particles. Langmuir 6. doi:10.1021/la00093a013
Kasztelan, S., Toulhoat, H., Grimblot, J., and Bonnelle, J. P. (1984). A Geometrical Model of the Active Phase of Hydrotreating Catalysts. Appl. Catal. 13, 127–159. doi:10.1016/S0166-9834(00)83333-3
Kulkarni, P. S., and Afonso, C. A. M. (2010). Deep Desulfurization of Diesel Fuel Using Ionic Liquids: Current Status and Future Challenges. Green Chemistry, 12 (7). doi:10.1039/c002113j
Li, S., Liu, Y., Feng, X., Chen, X., and Yang, C. (2019). Insights into the Reaction Pathway of Thiophene Hydrodesulfurization over Corner Site of MoS2 Catalyst: A Density Functional Theory Study. Mol. Catal. 463, 45–53. doi:10.1016/j.mcat.2018.11.018
Liu, J., Li, W.-Y., Feng, J., and Gao, X. (2021). Effects of Fe Species on Promoting the Dibenzothiophene Hydrodesulfurization over the Pt/γ-Al2O3 Catalysts. Catal. Today 371, 247–257. doi:10.1016/j.cattod.2020.07.035
Liu, Y., Li, F., Xia, Q., Wu, J., Liu, J., Huang, M., et al. (2018). Conductive 3D Sponges for Affordable and Highly-Efficient Water Purification. Nanoscale 10, 4771–4778. doi:10.1039/C7NR09435C
Liu, Y., Liu, F., Ding, N., Hu, X., Shen, C., Li, F., et al. (2020). Recent Advances on Electroactive CNT-Based Membranes for Environmental Applications: The Perfect Match of Electrochemistry and Membrane Separation. Chin. Chem. Lett. 31. doi:10.1016/j.cclet.2020.03.011
Manoli, J.-M., Da Costa, P., Brun, M., Vrinat, M., Maugé, F., and Potvin, C. (2004). Hydrodesulfurization of 4,6-dimethyldibenzothiophene over Promoted (Ni,P) Alumina-Supported Molybdenum Carbide Catalysts: Activity and Characterization of Active Sites. J. Catal. 221 (2), 365–377. doi:10.1016/j.jcat.2003.08.011
Mohebali, G., and Ball, A. S. (2016). Biodesulfurization of Diesel Fuels - Past, Present and Future Perspectives. Int. Biodeterioration Biodegradation 110, 163–180. doi:10.1016/j.ibiod.2016.03.011
Monticello, D. J. (2001). Biodesulfurization and the Upgrading of Petroleum Distillates. Curr. Opin. Biotechnol. 11, 540–546. doi:10.1016/s0958-1669(00)00154-3
Naboulsi, I., Felipe Linares Aponte, C., Lebeau, B., Brunet, S., Michelin, L., Bonne, M., et al. (2017). An Unexpected Pathway for Hydrodesulfurization of Gazole over a CoMoS Active Phase Supported on a Mesoporous TiO2 Catalyst. Chem. Commun. 53 (18), 2717–2720. doi:10.1039/C7CC00848A
Nikulshin, P. A., Salnikov, V. A., Mozhaev, A. V., Minaev, P. P., Kogan, V. M., and Pimerzin, A. A. (2014). Relationship between Active Phase Morphology and Catalytic Properties of the Carbon-Alumina-Supported Co(Ni)Mo Catalysts in HDS and HYD Reactions. J. Catal. 309, 386–396. doi:10.1016/j.jcat.2013.10.020
Ning, X., Li, Y., Dong, B., Wang, H., Yu, H., Peng, F., et al. (2017). Electron Transfer Dependent Catalysis of Pt on N-Doped Carbon Nanotubes: Effects of Synthesis Method on Metal-Support Interaction. J. Catal. 348, 100–109. doi:10.1016/j.jcat.2017.02.011
Niquillerothlisberger, A., and Prins, R. (2006). Hydrodesulfurization of 4,6-dimethyldibenzothiophene and Dibenzothiophene over Alumina-Supported Pt, Pd, and Pt-Pd Catalysts. J. Catal. 242 (1), 207–216. doi:10.1016/j.jcat.2006.06.009
Okamoto, Y., and Kubota, T. (2003). A Model Catalyst Approach to the Effects of the Support on Co–mo Hydrodesulfurization Catalysts. Catal. Today 86 (1-4), 31–43. doi:10.1016/S0920-5861(03)00402-4
Ortega-Domínguez, R. A., Mendoza-Nieto, J. A., Hernández-Hipólito, P., Garrido-Sánchez, F., Escobar-Aguilar, J., Barri, S. A. I., et al. (2015). Influence of Na Content on Behavior of NiMo Catalysts Supported on Titania Nanotubes in Hydrodesulfurization. J. Catal. 329, 457–470. doi:10.1016/j.jcat.2015.05.005
Rana, M., Ramirez, J., Gutierrezalejandre, A., Ancheyta, J., Cedeno, L., and Maity, S. (2007). Support Effects in CoMo Hydrodesulfurization Catalysts Prepared with EDTA as a Chelating Agent. J. Catal. 246 (1), 100–108. doi:10.1016/j.jcat.2006.11.025
Schuit, G. C. A., and Gates, B. C. (1973). Chemistry and Engineering of Catalytic Hydrodesulfurization. Aiche J. 19, 417–438. doi:10.1002/aic.690190303
Schüth, F. (2009). Challenges in Hydrogen Storage. Eur. Phys. J. Spec. Top. 176, 155–166. doi:10.1140/epjst/e2009-01155-x
Selvavathi, V., Chidambaram, V., Meenakshisundaram, A., Sairam, B., and Sivasankar, B. (2009). Adsorptive Desulfurization of Diesel on Activated Carbon and Nickel Supported Systems. Catal. Today 141 (1-2), 99–102. doi:10.1016/j.cattod.2008.05.009
Tanimu, A., and Alhooshani, K. (2019). Advanced Hydrodesulfurization Catalysts: A Review of Design and Synthesis. Energy Fuels 33 (4), 2810–2838. doi:10.1021/acs.energyfuels.9b00354
Van Haandel, L., Bremmer, M., Kooyman, P. J., van Veen, J. A. R., Weber, T., and Hensen, E. J. M. (2015). Structure-Activity Correlations in Hydrodesulfurization Reactions over Ni-Promoted MoxW(1-x)S2/Al2O3 Catalysts. ACS Catal. 5 (12), 7276–7287. doi:10.1021/acscatal.5b01806
Varga, Z., Szarvas, T., Tétényi, P., Hancsók, J., and Ollár, T. (2017). The Particular Characteristics of the Active Sites of MoS2, WS2 Catalysts in Thiophene Hydrodesulfurization. Reac Kinet Mech. Cat 124 (1), 61–74. doi:10.1007/s11144-017-1283-y
Vázquez-Garrido, I., López-Benítez, A., Berhault, G., and Guevara-Lara, A. (2019). Effect of Support on the Acidity of NiMo/Al2O3-MgO and NiMo/TiO2-Al2O3 Catalysts and on the Resulting Competitive Hydrodesulfurization/hydrodenitrogenation Reactions. Fuel 236, 55–64. doi:10.1016/j.fuel.2018.08.053
Wagenhofer, M. F., Shi, H., Gutiérrez, O. Y., Jentys, A., and Lercher, J. A. (2020). Enhancing Hydrogenation Activity of Ni-Mo Sulfide Hydrodesulfurization Catalysts. Sci. Adv. 6, eaax5331. doi:10.1126/sciadv.aax5331
Wang, X., Du, P., Chi, K., Duan, A., Xu, C., Zhao, Z., et al. (2017). Synthesis of NiMo Catalysts Supported on Mesoporous Silica FDU-12 with Different Morphologies and Their Catalytic Performance of DBT HDS. Catal. Today 291, 146–152. doi:10.1016/j.cattod.2016.10.035
Wang, X., Xiao, C., Zheng, P., Zhao, Z., Alabsi, M. H., Shi, Y., et al. (2020). Dendritic Micro-mesoporous Composites with center-radial Pores Assembled by TS-1 Nanocrystals to Enhance Hydrodesulfurization Activity of Dibenzothiophene and 4,6-dimethyldibenzothiophene. J. Catal. 384, 136–146. doi:10.1016/j.jcat.2020.02.013
Wang, X., Zhao, Z., Zheng, P., Chen, Z., Duan, A., Xu, C., et al. (2016). Synthesis of NiMo Catalysts Supported on Mesoporous Al2O3 with Different crystal Forms and superior Catalytic Performance for the Hydrodesulfurization of Dibenzothiophene and 4,6-dimethyldibenzothiophene. J. Catal. 344, 680–691. doi:10.1016/j.jcat.2016.10.016
Weng, X., Cao, L., Zhang, G., Chen, F., Zhao, L., Zhang, Y., et al. (2020). Ultradeep Hydrodesulfurization of Diesel: Mechanisms, Catalyst Design Strategies, and Challenges. Ind. Eng. Chem. Res. 59 (49), 21261–21274. doi:10.1021/acs.iecr.0c04049
Xu, J., Huang, T., and Fan, Y. (2017). Highly Efficient NiMo/SiO2-Al2O3 Hydrodesulfurization Catalyst Prepared from Gemini Surfactant-Dispersed Mo Precursor. Appl. Catal. B: Environ. 203, 839–850. doi:10.1016/j.apcatb.2016.10.078
Yin, C.-l., Zhai, X.-p., Zhao, L.-y., Liu, C.-g., Zhai, X.-p., Zhao, L.-y., et al. (2013). Mechanism of Hydrodesulfurization of Dibenzothiophenes on Unsupported NiMoW Catalyst. J. Fuel Chem. Tech. 41 (8), 991–997. doi:10.1016/S1872-5813(13)60043-2
Zeng, Q., Chang, S., Wang, M., Li, M., Deng, Q., Xiong, Z., et al. (2021). Highly-active, Metal-free, Carbon-Based ORR Cathode for Efficient Organics Removal and Electricity Generation in a PFC System. Chin. Chem. Lett. 32 (7), 2212–2216. doi:10.1016/j.cclet.2020.12.062
Zepeda, T. A., Pawelec, B., Díaz de León, J. N., de los Reyes, J. A., and Olivas, A. (2012). Effect of Gallium Loading on the Hydrodesulfurization Activity of Unsupported Ga2S3/WS2 catalystsEffect of Gallium Loading on the Hydrodesulfurization Activity of Unsupported Ga2S3/WS2 Catalysts. Appl. Catal. B: Environ. 111-112, 10–19. doi:10.1016/j.apcatb.20n.09.00810.1016/j.apcatb.2011.09.008
Zhou, W., Liu, M., Zhang, Q., Wei, Q., Ding, S., and Zhou, Y. (2017a). Synthesis of NiMo Catalysts Supported on Gallium-Containing Mesoporous Y Zeolites with Different Gallium Contents and Their High Activities in the Hydrodesulfurization of 4,6-Dimethyldibenzothiophene. ACS Catal. 7 (11), 7665–7679. doi:10.1021/acscatal.7b02705
Zhou, W., Zhang, Q., Zhou, Y., Wei, Q., Du, L., Ding, S., et al. (2018a). Effects of Ga- and P-Modified USY-Based NiMoS Catalysts on Ultra-deep Hydrodesulfurization for FCC Diesels. Catal. Today 305, 171–181. doi:10.1016/j.cattod.2017.07.006
Zhou, W., Zhang, Y., Tao, X., Zhou, Y., Wei, Q., and Ding, S. (2018b). Effects of Gallium Addition to Mesoporous Alumina by Impregnation on Dibenzothiophene Hydrodesulfurization Performances of the Corresponding NiMo Supported Catalysts. Fuel 228, 152–163. doi:10.1016/j.fuel.2018.04.084
Keywords: Ga modification, HDS catalyst, 4,6-DMDBT conversion rate, DDS route selectivity, active phase
Citation: Huang M, Huang W, Li A, Yang H, Jia Y, Yu Z, Xu Z, Wang X, Zhou Y and Wei Q (2022) Effect of Gallium as an Additive Over Corresponding Ni–Mo/γ-Al2O3 Catalysts on the Hydrodesulfurization Performance of 4,6-DMDBT. Front. Chem. 10:865375. doi: 10.3389/fchem.2022.865375
Received: 29 January 2022; Accepted: 17 February 2022;
Published: 15 March 2022.
Edited by:
Qingyi Zeng, University of South China, ChinaCopyright © 2022 Huang, Huang, Li, Yang, Jia, Yu, Xu, Wang, Zhou and Wei. This is an open-access article distributed under the terms of the Creative Commons Attribution License (CC BY). The use, distribution or reproduction in other forums is permitted, provided the original author(s) and the copyright owner(s) are credited and that the original publication in this journal is cited, in accordance with accepted academic practice. No use, distribution or reproduction is permitted which does not comply with these terms.
*Correspondence: Qiang Wei, qwei@cup.edu.cn