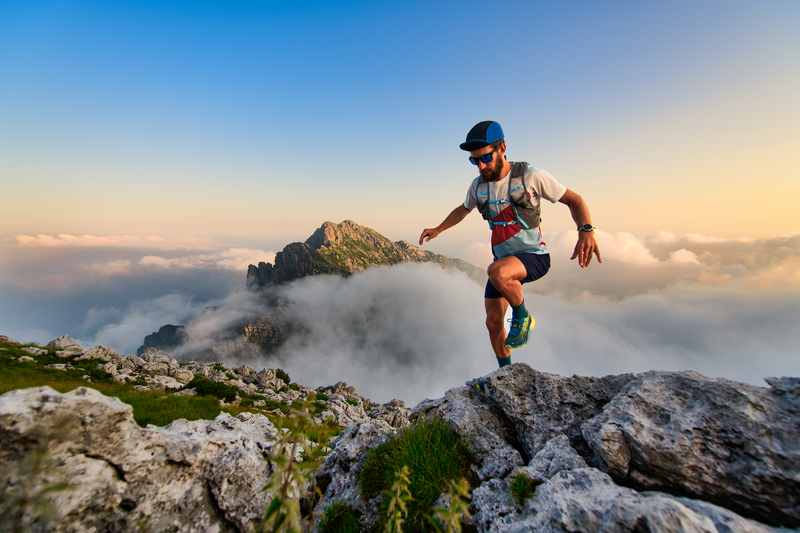
95% of researchers rate our articles as excellent or good
Learn more about the work of our research integrity team to safeguard the quality of each article we publish.
Find out more
ORIGINAL RESEARCH article
Front. Chem. , 02 June 2022
Sec. Inorganic Chemistry
Volume 10 - 2022 | https://doi.org/10.3389/fchem.2022.864143
This article is part of the Research Topic Inorganic Materials for Energy and Environmental Catalysis View all 18 articles
In this work, NiO modified BiVO4 (BiVO4/NiO) nanocomposite was synthesized using hydrothermal and calcination method. The composite of BiVO4/NiO, further employed as a low-overpotential photoanode, was consisted of BiVO4 nanoparticles and NiO nanosheets, in which the BiVO4 nanoelectrode served as the matrix for the attachment of NiO nanosheets. Photoelectrochemical (PEC) tests show that BiVO4/NiO displayed improved PEC performance compared with pure BiVO4. The BiVO4/NiO photoanode delivers a photocurrent density of 1.2 mA/cm2 at 1.23 V vs. RHE in a Na2SO4 electrolyte under an AM 1.5G solar simulator, which is 0.3 mA/cm2 higher than pure BiVO4 photoanode. Meanwhile, the onset potential also generates a 350 mV cathodic shift. The enhanced performance of the BiVO4/NiO nanocomposite is attributed to NiO unique lamellar structure capable of providing a large number of active sites. Measurements of electrochemical impedance spectra (EIS) and the incident photon-to-current efficiency (IPCE) illustrate that the enhanced PEC activities are ascribed to the improved charge carrier separation/transport and the promoted water oxidation kinetics furnished by the decoration of NiO cocatalyst.
Due to the excessive consumption of fossil energy that results in severe environmental pollution worldwide, the development of clean and sustainable energy technologies has received increasing attention. (Iwase et al., 2011; Guo et al., 2014; Zeng et al., 2021a) Clean hydrogen production is seen as a promising strategy, capable of simutaneously addressing climate change and environmental issues related to fossil fuel combustion. (Wang L. et al., 2018; Fukuzumi et al., 2018; Kim et al., 2018) Photoelectrochemical (PEC) water splitting, capable of directly converting solar energy into chemical energy, is considered a promising technology for converting solar energy into stable chemical energy, thus becoming attractive for reducing pollution associated with energy production. (Zhang J. et al., 2016; Wang and Wang, 2018; Weng et al., 2018; Zeng et al., 2019) In the PEC system, the photoanode acts the role of reaction sites for effective oxygen evolution. (Liu et al., 2014; Roger et al., 2017) In conclusion, the development of efficient photoanode materials is of great significance for constructing a practical PEC water splitting system. Various semiconductors including TiO2 (Crake et al., 2017; Zeng et al., 2020), ZnO (Han et al., 2015; Hong et al., 2015), α-Fe2O3 (Dotan et al., 2011; Huang et al., 2016), WO3 (Huang et al., 2017; Li et al., 2018), BiVO4 (Wang et al., 2017a; Wang Q. et al., 2018) and BiOBr (Wang Z.-Q. et al., 2020) etc. have been developed as photocatalytic materials. Among them, scheelite-monoclinic bismuth vanadate (BiVO4) has been widely studied for PEC water splitting owing to its relatively narrow band gap of 2.4 eV for visible-light absorption, as well as an appropriate band position for effective water oxidation and high stablility. (Malathi et al., 2018) However, the application of BiVO4 is still restricted by its inherent defects such as low charge transport (Zhou et al., 2018), high charge recombination (Zhang Y. et al., 2016) and slow poor water oxidation kinetics (Zhai et al., 2017). The photocurrent density of the pure BiVO4 is obviously lower than its theoretical value of 7.5 mA/cm2 (Xu et al., 2014; Chen, 2015) To overcome these issues, transition metal-based catalysts used as cocatalysts are one of the effective ways to improve the PEC water splitting performance.
Transition metal-based materials, especially (Co., Ni, Fe)-based materials, comparable to precious metals because of their low cost and advanced catalytic performance, are considered to be the most promising OER catalysts. (Zhang Q. et al., 2016; Kuang et al., 2016; Wang Z. et al., 2020)The combination of the OER catalyst and the semiconductor light absorber can not only improve the PEC activity by providing an interface reaction active site that reduces overpotential, but also improve the PEC stability by rapidly consuming photo-generated carriers of semiconductor materials against electrolytes (Zhou et al., 2015). (Trotochaud et al., 2014; Zeng et al., 2021b) Recently, Dai (Kenney et al., 2013; Li et al., 2017) and colleagues deposited an ultra-thin nickel film on the n-type silicon substrate as a physical protective layer. It was found that a 2 nm nickel film played a crucial role in the sustainability of n-type silicon photoanodes in the solar-driven water oxidation process. The ultra-thin nickel was used as a protective layer and a passivation layer, and the natural NiOx formed during the test was employed as an OER promoter. The NiOx/Ni/n-Si photoanode can work under constant photocurrent of 10 mA/cm2, and still have excellent stability after water oxidation of 80 h. In addition, Lewis (Zhou et al., 2019) and his colleagues introduced an ultra-thin CoOx film as an intermediate layer between NiOx layer and n-Si to enhance the interaction between co-catalysts/semiconductors. It was found that further passivation of the CoOx layer on the n-Si surface can change the initiation. The potential was more negative than NiOx/SiOx/n-Si photoanode. NiOx/CoOx/SiOx/n-Si showed the most negative flat band position, which was related to the barrier height in the semiconductor, and therefore highly improved the separation and collection of charge carriers. The above results indicate that the combination of NiO and other favorable semiconductors can remarkably reduce the overpotential of the photoelectrode.
In this study, a BiVO4 photoanode, acting the role of photoelectrocatalysis substrate, is synthesised by a electrodeposition-calcination method. On the other hand, a nanosheet structured NiO, playing the performance of the water oxidation cocatalyst to combine with the BiVO4 photoanode and thus improve the PEC performance, is preprared via a hydrothermal-calcination. Under AM 1.5G sunlight, the BiVO4/NiO film produced a relatively high photocurrent density of 1.2 mA/cm2 at 1.23 V vs. RHE, much higher than that of the pure BiVO4 film. More importantly, the onset potential is negatively shifted by 350 mV relative to pure BiVO4. The special structure of NiO is believed to be beneficial to absorb more incident photons through the light-harvesting effect, thereby enhancing the separation and transport of photo-induced charge carriers. Furthermore, the deposition of NiO cocatalyst on the surface of the BiVO4 photoanode significantly promotes the water oxidation kinetics.
Bi(NO3)3·5H2O (Sinopharm Chemical Reagent Co., Ltd., 99.0%), potassium iodide, ethylene glycol are purchased from chemical reagent co, Ltd. P-benzoquinone (Tianjin Institute of Fine Chemicals, 99.0%). Ni(NO3)2·6H2O (Sinopharm Chemical Reagent Co., Ltd., 99.0%), hexamethylenetetramine (HMTA, Chengdu Cologne Chemicals Co., Ltd., 99.0%), Anhydrous ethanol was purchased from Sinopharm Chemical Reagent Co., Ltd. All aqueous solutions were prepared with deionized water.
The preparation of BiVO4 was synthesized with reference to previous reported work. Systematically, 50 ml of 0.4 M KI solution was first adjusted to pH 1.7 with 1 M HNO3, and then 5 mmol Bi(NO3)3·5H2O was added with rapid stirring until dissolved, resulting in an orange-red mixed solution. Then 20 ml of ethanol containing 4.6 mmol of 1,4-benzoquinone was slowly added dropwise to the above solution and stirred for several tens of minutes. Next, BiOI nanosheets were synthesized in a three-electrode system by electrodeposition. Among them, the platinum electrode was used as the counter electrode, the clean FTO glass was used as the working electrode, and the Ag/AgCl (3.5 M KCl) electrode was used as the reference electrode. Cyclic voltammetry (CV) was used for electrodeposition, and the resulting membrane was rinsed with distilled water to obtain a clean BiOI membrane. Immediately after, 150 μl of 0.2 M vanadyl acetylacetonate (VO(acac)2) DMSO solution was dropped onto the above BiOI nanosheets. Calcined at 450°C for 2 h at a ramp rate of 2°C/min. The cooled membrane was washed with 1 M NaOH solution to remove excess V2O5 from the BiVO4 electrode.
The BiVO4/NiO photoanode was prepared by a hydrothermal method. The configuration takes 60 ml of solution in which the volume ratio of deionized water and ethanol solution is 2:1. After ultrasonically mixed uniformly, 1.5 mmol Ni(NO3)2·6H2O and 6 mmol HMTA were added thereto, and stirred until dissolved. Then 20 ml of the above mixture was added to a PTFE-lined stainless steel autoclave (100 ml). And the as-prepared BiVO4 photoanode was placed obliquely with the conductive side facing upwards, heated at 90°C for 4 h. The photoanode was washed three times with water and ethanol and dried at 60°C for 12 h. Finally, the BiVO4/NiO photoanode was obtained after calcination in air with a heating rate of 2°C/min at 300°C for 2 h.
The microscopic morphology of the samples was characterized by scanning electron microscopy (SEM, JSM-6701E). The crystal structure of the as-prepared photoanode was measured by X-ray diffraction (XRD) tests on the X-ray diffractometer (D/MAX-2200/PC). UV-Vis diffuse reflectance spectroscopy was used to investigate the response of the prepared photoelectrode to visible light on a UV-3100 spectrometer.
The photoelectrochemical tests of the as-prepared photoanodes were carried out on a CHI 660D electrochemical workstation. A three-electrode system was used, in which a platinum sheet, Ag/AgCl (3.5 M KCl), and the samples were the counter electrode, the reference electrode and the working electrode, respectively. The electrolyte is 0.5 M Na2SO4 solution (pH = 6.86). All tests were performed with FTO backside irradiation at room temperature. The scan rate for linear sweep voltammetry (LSV) was 10 mV s−1. The light intensity was calibrated to 100 mW/cm2 with an optical power meter. And the incident photon current efficiency (IPCE) was measured using a 300 W xenon lamp with a monochromator in 0.5 M Na2SO4 electrolyte at 1.23 V vs. RHE. The photogenerated photocurrent
where Jabs is obtained by integrating the distribution of solar power density
where
The photocurrent during sodium sulfite oxidation was measured
The morphology and elemental compositions of the synthesized BiVO4, BiVO4/NiO composite photoanode were studied with SEM and energy dispersive spectroscopy (EDS) in Figures 1A–H. Figure 1A exhibits the SEM image of nanoporous BiVO4 film. Figure 1B shows the SEM image of BiVO4/NiO. NiO nanosheets with size distribution about 5–10 nm are fairly continuous and uniformly loaded on the surface of BiVO4 in large area. The elemental composition and content of BiVO4/NiO anodes were further investigated by EDS. Figure 1C shows the EDS pattern of BiVO4/NiO photoanode, the weight percentage of elements present in the BiVO4/NiO photoanode composites are 39.4%, 31.1%, 18.6% and 10.09% for Bi, O, V and Ni, respectively. No other elements or impurities are found. The elemental composition and distribution in the BiVO4/NiO photoanode were further observed by EDS elemental mapping (Figures 1D–H). The results clarify that the elements of Bi, O, V and Ni are present and uniformly distributed in the BiVO4/NiO photoanode.
FIGURE 1. SEM images of the typical samples: (A) BiVO4, (B) BiVO4/NiO, EDS pattern (C) and the EDS elemental mapping (D–H) of as prepared BiVO4/NiO photoanode.
The optical absorption properties of pure BiVO4 and BiVO4/NiO films were investigated by UV-Vis diffuse reflectance spectroscopy. It can be seen that BiVO4 and BiVO4/NiO show good light absorption properties around 500 nm (Figure 2A), corresponding to a band gap of 2.4 eV. Notably, the BiVO4/NiO sample shows almost the same absorption edge as bare BiVO4 due to the blocking by the thicker BiVO4 layer, indicating that coating of NiO almost rarely affects light absorption of BiVO4/NiO. But the BiVO4 photocathode loaded with NiO co-catalyst shows the relatively low light absorption capacity. The reason is primarily ascribed to the poor optical transparency of the nickel based co-catalyst. (Zhou et al., 2020)
FIGURE 2. (A) UV−Vis diffuse reflectance spectra of BiVO4 and BiVO4/NiO. (B) XRD patterns of the BiVO4 and BiVO4/NiO photoanodes.
The XRD pattern characterization reveals that the product is composed of three kinds of materials with distinct crystal structures. Figure 2B displays the XRD patterns of BiVO4 and BiVO4/NiO nanocomposites. The XRD diffraction peaks of BiVO4 and BiVO4/NiO nanocomposites are completely consistent with monoclinic BiVO4 (JCPDS No. 14-0688) and tetragonal SnO2 (JCPDS No. 46-1088) derived from FTO substrates. No other impurity phases were detected. The appearance of characteristic diffraction peaks at 2θ = 43.3° is corresponding to the (200) crystal plane of the cubic phase NiO, which can be concluded that the composite sample has been successfully prepared.
In order to explore the effect of supported NiO on the PEC performance, the photoelectrochemical water splitting performance of BiVO4 photoanode modified by NiO cocatalyst was studied by electrochemical workstation. Linear sweep voltammetry (LSV) curves reflect the water oxidation properties of BiVO4/NiO and pure BiVO4. As shown in Figure 3A, the photocurrent density of unmodified BiVO4 at 1.23 V vs. RHE is 0.9 mA/cm2 and the onset potential is about 0.58 V vs. RHE, which is due to its unique structure and specific crystal orientation, leading to rapid transfer and separation of photogenerated carriers. After loading the NiO cocatalyst on BiVO4, the photoelectrode showed significant enhancement at all potentials, obtaining a photocurrent of 1.2 mA/cm2 at 1.23 V vs. RHE, which was higher than that of pristine BiVO4. In particular, BiVO4/NiO obtained a more negative onset potential compared to pure BiVO4, with a negative shift of about 0.35 V (Figure 3B). The increased photocurrent density and negatively shifted onset potential clearly indicate that the addition of NiO cocatalyst is a feasible way to enhance the water oxidation capacity of BiVO4 photoanode. Figure 3C shows the chopped photocurrent density−voltage (J−V) curves of BiVO4/NiO and pure BiVO4. All the photoanodes show an obvious “photo-switching” effect with fast response. Clearly, the BiVO4/NiO photoanode exhibits a much better PEC performance than BiVO4 film.
FIGURE 3. (A) Photocurrent density-voltage curve of BiVO4 and BiVO4/NiO photoanodes. (B) Photocurrent density-voltage curve of BiVO4 and BiVO4/NiO photoanodes in the absence of light. (C) Chopped linear sweep photocurrent-potential curve of BiVO4 and BiVO4/NiO. (D) Sulfite oxidation current curves.
The electron-hole pair recombination and charge generation kinetics of photoanode in PEC water oxidation process can be analyzed by EIS. The photoanode was measured at 1.23 V vs. RHE at AM 1.5G (100 mW/cm2), and the frequency range of the Nyquist plot was from 100 kHz to 0.1 Hz. The results of impedance spectra are useful for analyzing electrochemical surface reactions. The charge transfer resistance of the photoanode surface is estimated from the small semicircle in the Nyquist diagram, and the smaller the radius, the more effective the separation of charges. In addition, the EIS test result of dark reaction condition (Figure 4B) is consistent with that under light conditions in Figure 4A. The BiVO4/NiO photoanode exhibits the highest charge transportation, suggesting good charge separation ability.
FIGURE 4. (A) EIS curves of pure BiVO4 and BiVO4/NiO under the light, (B) and in the dark. The EIS was measured at 1.23 V vs. RHE under an AM 1.5G solar simulator. (C) Charge separation efficiency versus potential curves and (D) charge injection efficiency versus potential curves of BiVO4, BiVO4/NiO.
To further explore the charge recombination at the BiVO4 and BiVO4/NiO photoanode interfaces, the charge separation and injection efficiencies were tested in Figures 4B,C. Charge separation efficiency is an important parameter to evaluate the proportion of carriers reaching the electrode surface/electrolyte interface to participate in water oxidation (Wang et al., 2017b). Therefore, the constant charge separation efficiency is shown in Figure 3D, ascribed to the photocurrent density of the BiVO4/NiO photoanode at an applied potential of 0.6 V vs. RHE when Na2SO3 was added to the electrolyte. The separation efficiency of the pure BiVO4 photoanode increases with the applied potential, especially it can reach about 60% at 1.23 V vs. RHE. However, the BiVO4/NiO nanostructured array photoanode exhibits a charge separation efficiency of 90% at 1.23 V vs. RHE. From this point of view, the cocatalyst NiO supported on BiVO4 can significantly improve the charge separation efficiency and facilitate the flow of charge carriers to the electrode surface/electrolyte interface to participate in water oxidation.
The photogenerated holes generated on the surface of the photoanode participate in the water oxidation reaction or recombine with electrons. The charge injection efficiency, defined as the fraction of those holes at the photoanode and electrolyte interface that is used for water oxidation reactions, can be improved by reducing surface recombination or accelerating hole transfer kinetics. (Zhou et al., 2020) As shown in Figure 4D, the charge injection efficiency of the pure BiVO4 photoanode reaches 28% 1.23 V vs. RHE. While the charge injection efficiency of the BiVO4/NiO photoanode increases to 35% in the potential range of 1.23 V vs. RHE.
The quantum efficiencies of BiVO4, BiVO4/NiO photoanodes were determined by measuring incident photon current efficiency (IPCE) and absorbed photon current efficiency (APCE). (Wang et al., 2017b) The calculation of IPCE can refer to the following equation:
where J is the current density (mA/cm2) measured at each specific wavelength, λ is the wavelength of the incident light (nm), and P is the power density of the incident light (mW/cm2). As shown in Figure 5A, BiVO4/NiO nanocomposites exhibit slightly higher IPCE values in the 450–500 nm range compared to bare BiVO4. The PEC performance mainly depends on the light-harvesting efficiency, charge separation efficiency, and collection yield. Since the light-harvesting efficiency is almost unchanged after the modification of the NiO cocatalyst, the enhancement of IPCE may be due to the fast separation of charge carriers and the accelerated water oxidation kinetics of the reaction, resulting in the enhanced photocurrent. The IPCE results are consistent with the above J-V measurements.
FIGURE 5. (A) IPCE of BiVO4, BiVO4/NiO measured at 1.23 V vs. RHE in the incident wavelength range from 380 to 600 nm. (B) APCE spectra for BiVO4, BiVO4/NiO photocathodes along with the AM 1.5 irradiance spectrum.
To obtain the absorbed photon-current efficiency, the APCE value of the photoanode was measured at 0.6 V vs. RHE, as shown in Figure 5B. The APCE value of BiVO4/NiO photoanode is significantly higher than that of BiVO4 from 380 to 500 nm, which is consistent with the overall PEC performance.
Figure 6A shows the Mott Schottky barrier of BiVO4 and BiVO4/NiO. The capacitance-voltage curve is usually used to analyze the reasons for the enhancement of semiconductor performance. In order to better study the reasons for the increase of photocurrent after supporting the cocatalyst. Therefore, the capacitance-voltage curves of BiVO4 and BiVO4/NiO electrodes were measured under dark reaction conditions, and the x-axis tangent was made on the curves of BiVO4 and BiVO4/NiO. The tangent was positive, indicating that BiVO4 and BiVO4/NiO are both n-type semiconductors. The smaller slope of the composite electrode, the greater the carrier density. Thus, the BiVO4/NiO electrode has the largest carrier density. The increased carrier density causes the conductivity of BiVO4 to increase and ultimately increases its photocurrent density. Fluorescence spectroscopy (PL) can be used to effectively analyze the separation and recombination effects of photogenerated carriers. As shown in Figure 6B, it can be observed that the peak intensity of the BiVO4/NiO electrode is weaker than that of the pure BiVO4 electrode, which indicates that after NiO is loaded on the BiVO4 surface, the recombination rate of photo-generated electrons and holes becomes slower and the charge separation efficiency is improved.
Stability test is an important index parameter to evaluate the effect of photoelectric catalyst and whether it has application value. The stability test of the BiVO4/NiO photoanode was analyzed under continuous irradiation under AM 1.5G. As shown in Figure 7, it can be observed that under the continuous irradiation of 3 h, the photocurrent density of the NiO/BiVO4 electrode is not significantly attenuated, indicating that the stability of the BiVO4 photoanode can be improved after loading NiO.
Based on above results, the possible mechanisms for the enhancement in photoelectrocatalytic activity of BiVO4/NiO composite photoanode and the specific photogenerated charge carriers transfer are shown in Figure 8. In BiVO4 with monoclinic scheelite structure, the Bi 6s and O 2p orbits hybridize to form the valence band. When the BiVO4/NiO composite is irradiated with visible light, electron-hole pairs are generated in BiVO4, in which electrons in the valence band are excited to the conduction band and holes stay in the conduction band. With the NiO coated on the surface of BiVO4, NiO as a cocatalyst regulates the built-in electric field of BiVO4 photocatalyst, accelerates the charge separation rate of BiVO4, and thus the PEC performance of BiVO4 is improved.
FIGURE 8. Schematic illustration of the fabrication process of BiVO4/NiO and the light harvesting and carrier separation mechanism in the BiVO4/NiO composite photoanode system.
In conclusion, we successfully fabricated an efficient nanostructured BiVO4/NiO photoanode by a two-step method of hydrothermal calcination synthesis. The PEC performance of the NiO-modified BiVO4 photoanode was improved in 0.5 M Na2SO4 (pH = 6.86) electrolyte, reaching 1.2 mA/cm2 at 1.23 V vs. RHE, higher than that of the pure BiVO4 sample. In particular, the onset potential of the composite photoanode has a significant negative shift. The excellent PEC performance could be attributed to NiO abundant nano flake structure, the improved charge separation/transport efficiency and accelerated water oxidation kinetics thanks to the deposited NiO cocatalyst. Our work shed a light for design and fabrication of nanostructured photoelectrode with efficient PEC performances.
The original contributions presented in the study are included in the article/Supplementary Material, further inquiries can be directed to the corresponding author.
Z-QW: Writing—review and editing. HJW: Methodology.
This work was financially supported by the National Natural Science Foundation of China (Nos. 51773184 and U1810114).
The authors declare that the research was conducted in the absence of any commercial or financial relationships that could be construed as a potential conflict of interest.
All claims expressed in this article are solely those of the authors and do not necessarily represent those of their affiliated organizations, or those of the publisher, the editors and the reviewers. Any product that may be evaluated in this article, or claim that may be made by its manufacturer, is not guaranteed or endorsed by the publisher.
Chen, P. (2015). A Promising Strategy to Fabricate the Cu/BiVO4 Photocatalysts and Their Enhanced Visible-Light-Driven Photocatalytic Activities. J. Mater. Sci. Mater. Electron. 27 (3), 2394–2403. doi:10.1007/s10854-015-4037-5
Crake, A., Christoforidis, K. C., Kafizas, A., Zafeiratos, S., and Petit, C. (2017). CO 2 Capture and Photocatalytic Reduction Using Bifunctional TiO 2/MOF Nanocomposites under UV-Vis Irradiation. Appl. Catal. B: Environ. 210, 131–140. doi:10.1016/j.apcatb.2017.03.039
Dotan, H., Sivula, K., Grätzel, M., Rothschild, A., and Warren, S. C. (2011). Probing the Photoelectrochemical Properties of Hematite (α-Fe2O3) Electrodes Using Hydrogen Peroxide as a Hole Scavenger. Energy Environ. Sci. 4 (3), 958–964. doi:10.1039/c0ee00570c
Fukuzumi, S., Lee, Y.-M., and Nam, W. (2018). Thermal and Photocatalytic Production of Hydrogen with Earth-Abundant Metal Complexes. Coord. Chem. Rev. 355, 54–73. doi:10.1016/j.ccr.2017.07.014
Guo, K., Liu, Z., Zhou, C., Han, J., Zhao, Y., Liu, Z., et al. (2014). Fabrication of TiO2 Nano-Branched arrays/Cu2S Composite Structure and its Photoelectric Performance. Appl. Catal. B: Environ. 154-155, 27–35. doi:10.1016/j.apcatb.2014.02.004
Han, J., Liu, Z., Guo, K., Wang, B., Zhang, X., and Hong, T. (2015). High-efficiency Photoelectrochemical Electrodes Based on ZnIn2S4 Sensitized ZnO Nanotube Arrays. Appl. Catal. B: Environ. 163, 179–188. doi:10.1016/j.apcatb.2014.07.040
Hong, T., Liu, Z., Liu, H., Liu, J., Zhang, X., Han, J., et al. (2015). Preparation and Enhanced Photoelectrochemical Performance of Selenite-Sensitized Zinc Oxide Core/shell Composite Structure. J. Mater. Chem. A. 3 (8), 4239–4247. doi:10.1039/c4ta05973e
Huang, J., Hu, G., Ding, Y., Pang, M., and Ma, B. (2016). Mn-doping and NiFe Layered Double Hydroxide Coating: Effective Approaches to Enhancing the Performance of α-Fe2O3 in Photoelectrochemical Water Oxidation. J. Catal. 340, 261–269. doi:10.1016/j.jcat.2016.05.007
Huang, J., Zhang, Y., and Ding, Y. (2017). Rationally Designed/Constructed CoOx/WO3 Anode for Efficient Photoelectrochemical Water Oxidation. ACS Catal. 7 (3), 1841–1845. doi:10.1021/acscatal.7b00022
Iwase, A., Ng, Y. H., Ishiguro, Y., Kudo, A., and Amal, R. (2011). Reduced Graphene Oxide as a Solid-State Electron Mediator in Z-Scheme Photocatalytic Water Splitting under Visible Light. J. Am. Chem. Soc. 133 (29), 11054–11057. doi:10.1021/ja203296z
Kenney, M. J., Gong, M., Li, Y., Wu, J. Z., Feng, J., Lanza, M., et al. (2013). High-performance Silicon Photoanodes Passivated with Ultrathin Nickel Films for Water Oxidation. Science 342 (6160), 836–840. doi:10.1126/science.1241327
Kim, C.-H., Han, J.-Y., Lim, H., Lee, K.-Y., and Ryi, S.-K. (2018). Hydrogen Production by Steam Methane Reforming in Membrane Reactor Equipped with Pd Membrane Deposited on NiO/YSZ/NiO Multilayer-Treated Porous Stainless Steel. J. Membr. Sci. 563, 75–82. doi:10.1016/j.memsci.2018.05.037
Kuang, Y., Jia, Q., Nishiyama, H., Yamada, T., Kudo, A., and Domen, K. (2016). A Front-Illuminated Nanostructured Transparent BiVO4 Photoanode for >2% Efficient Water Splitting. Adv. Energ. Mater. 6, 1501645. doi:10.1002/aenm.201501645
Li, L., Xiao, S., Li, R., Cao, Y., Chen, Y., Li, Z., et al. (2018). Nanotube Array-like WO3 Photoanode with Dual-Layer Oxygen-Evolution Cocatalysts for Photoelectrocatalytic Overall Water Splitting. ACS Appl. Energ. Mater. 1 (12), 6871–6880. doi:10.1021/acsaem.8b01215
Li, S., Chen, Z., Kong, W., Jia, X., Cai, J., and Dong, S. (2017). Effect of Polyethylene Glycol on the NiO Photocathode. Nanoscale Res. Lett. 12, 501. doi:10.1186/s11671-017-2267-6
Liu, Z., Guo, K., Han, J., Li, Y., Cui, T., Wang, B., et al. (2014). Dendritic TiO2/ln2S3/AgInS2Trilaminar Core-Shell Branched Nanoarrays and the Enhanced Activity for Photoelectrochemical Water Splitting. Small 10 (15), 3153–3161. doi:10.1002/smll.201400622
Malathi, A., Madhavan, J., Ashokkumar, M., and Arunachalam, P. (2018). A Review on BiVO4 Photocatalyst: Activity Enhancement Methods for Solar Photocatalytic Applications. Appl. Catal. A: Gen. 555, 47–74. doi:10.1016/j.apcata.2018.02.010
Roger, I., Shipman, M. A., and Symes, M. D. (2017). Earth-abundant Catalysts for Electrochemical and Photoelectrochemical Water Splitting. Nat. Rev. Chem. 1 (1), 0003. doi:10.1038/s41570-016-0003
Trotochaud, L., Young, S. L., Ranney, J. K., and Boettcher, S. W. (2014). Nickel-Iron Oxyhydroxide Oxygen-Evolution Electrocatalysts: The Role of Intentional and Incidental Iron Incorporation. J. Am. Chem. Soc. 136 (18), 6744–6753. doi:10.1021/ja502379c
Wang, L., Duan, S., Jin, P., She, H., Huang, J., Lei, Z., et al. (2018). Anchored Cu(II) Tetra(4-Carboxylphenyl)porphyrin to P25 (TiO2) for Efficient Photocatalytic Ability in CO2 Reduction. Appl. Catal. B: Environ. 239, 599–608. doi:10.1016/j.apcatb.2018.08.007
Wang, Q., He, J., Shi, Y., Zhang, S., Niu, T., She, H., et al. (2017b). Designing non-noble/semiconductor Bi/BiVO4 Photoelectrode for the Enhanced Photoelectrochemical Performance. Chem. Eng. J. 326, 411–418. doi:10.1016/j.cej.2017.05.171
Wang, Q., He, J., Shi, Y., Zhang, S., Niu, T., She, H., et al. (2017a). Synthesis of MFe 2 O 4 (M = Ni, Co)/BiVO 4 Film for Photolectrochemical Hydrogen Production Activity. Appl. Catal. B: Environ. 214, 158–167. doi:10.1016/j.apcatb.2017.05.044
Wang, Q., Niu, T., Wang, L., Yan, C., Huang, J., He, J., et al. (2018). FeF2/BiVO4 Heterojuction Photoelectrodes and Evaluation of its Photoelectrochemical Performance for Water Splitting. Chem. Eng. J. 337, 506–514. doi:10.1016/j.cej.2017.12.126
Wang, Z.-Q., Wang, H., Wu, X.-F., and Chang, T.-L. (2020). Oxygen Vacancies and P-N Heterojunction Modified BiOBr for Enhancing Donor Density and Separation Efficiency under Visible-Light Irradiation. J. Alloys Compd. 834, 155025. doi:10.1016/j.jallcom.2020.155025
Wang, Z., Lei, Q., Wang, Z., Yuan, H., Cao, L., Qin, N., et al. (2020). In-situ Synthesis of Free-Standing Feni-Oxyhydroxide Nanosheets as a Highly Efficient Electrocatalyst for Water Oxidation. Chem. Eng. J. 395, 125180. doi:10.1016/j.cej.2020.125180
Wang, Z., and Wang, L. (2018). Photoelectrode for Water Splitting: Materials, Fabrication and Characterization. Sci. China Mater. 61 (6), 806–821. doi:10.1007/s40843-018-9240-y
Weng, B., Grice, C. R., Meng, W., Guan, L., Xu, F., Yu, Y., et al. (2018). Metal-Organic Framework-Derived CoWP@C Composite Nanowire Electrocatalyst for Efficient Water Splitting. ACS Energ. Lett. 3 (6), 1434–1442. doi:10.1021/acsenergylett.8b00584
Xu, X., Zou, Q., Yuan, Y., Ji, F., Fan, Z., and Zhou, B. (2014). Preparation of BiVO4-Graphene Nanocomposites and Their Photocatalytic Activity. J. Nanomater. 2014, 1–6. doi:10.1155/2014/401697
Zeng, Q., Chang, S., Beyhaqi, A., Lian, S., Xu, H., Xie, J., et al. (2020). Efficient Solar Hydrogen Production Coupled with Organics Degradation by a Hybrid Tandem Photocatalytic Fuel Cell Using a Silicon-Doped TiO2 Nanorod Array with Enhanced Electronic Properties. J. Hazard. Mater. 394, 121425. doi:10.1016/j.jhazmat.2019.121425
Zeng, Q., Chang, S., Beyhaqi, A., Wang, M., and Hu, C. (2019). Efficient Electricity Production Coupled with Water Treatment via a Highly Adaptable, Successive Water-Energy Synergistic System. Nano Energy 67, 104237. doi:10.1016/j.nanoen.2019.104237
Zeng, Q., Chang, S., Wang, M., Li, M., Deng, Q., Xiong, Z., et al. (2021a). Highly-active, Metal-free, Carbon-Based ORR Cathode for Efficient Organics Removal and Electricity Generation in a PFC System. Chin. Chem. Lett. 32 (7), 2212–2216. doi:10.1016/j.cclet.2020.12.062
Zeng, Q., Fu, X., Chang, S., Zhang, Q., Xiong, Z., Liu, Y., et al. (2021b). Ordered Ti-Doped Fevo4 Nanoblock Photoanode with Improved Charge Properties for Efficient Solar Water Splitting. J. Colloid Interf. Sci. 604, 562–567. doi:10.1016/j.jcis.2021.07.037
Zhai, Y., Yin, Y., Liu, X., Li, Y., Wang, J., Liu, C., et al. (2017). Novel Magnetically Separable BiVO 4/Fe 3 O 4 Photocatalyst: Synthesis and Photocatalytic Performance under Visible-Light Irradiation. Mater. Res. Bull. 89, 297–306. doi:10.1016/j.materresbull.2017.01.011
Zhang, J., Liu, Z., and Liu, Z. (2016). Novel WO3/Sb2S3 Heterojunction Photocatalyst Based on WO3 of Different Morphologies for Enhanced Efficiency in Photoelectrochemical Water Splitting. ACS Appl. Mater. Inter. 8 (15), 9684–9691. doi:10.1021/acsami.6b00429
Zhang, Q., Li, Z., Wang, S., Li, R., Zhang, X., Liang, Z., Han, H., et al. (2016). The Effect of Redox Cocatalysts Location on Photocatalytic Overall Water Splitting over Cubic NaTaO3 Semiconductor Crystals Exposed with Equivalent Facets. ACS Catal. 4, 2182–2191. doi:10.1021/acscatal.5b02503
Zhang, Y., Wang, D., Zhang, X., Chen, Y., Kong, L., Chen, P., et al. (2016). Enhanced Photoelectrochemical Performance of Nanoporous BiVO 4 Photoanode by Combining Surface Deposited Cobalt-Phosphate with Hydrogenation Treatment. Electrochimica Acta 195, 51–58. doi:10.1016/j.electacta.2016.02.137
Zhou, C., Wang, S., Zhao, Z., Shi, Z., Yan, S., and Zou, Z. (2018). A Facet-dependent Schottky-Junction Electron Shuttle in a BiVO4 {010}-Au-Cu2 O Z-Scheme Photocatalyst for Efficient Charge Separation. Adv. Funct. Mater. 28 (31), 1801214. doi:10.1002/adfm.201801214
Zhou, S., Chen, K., Huang, J., Wang, L., Zhang, M., Bai, B., et al. (2020). Preparation of Heterometallic CoNi-MOFs-Modified BiVO4: a Steady Photoanode for Improved Performance in Photoelectrochemical Water Splitting. Appl. Catal. B: Environ. 266, 118513. doi:10.1016/j.apcatb.2019.118513
Zhou, S., Yue, P., Huang, J., Wang, L., She, H., and Wang, Q. (2019). High-performance Photoelectrochemical Water Splitting of BiVO4@Co-MIm Prepared by a Facile In-Situ Deposition Method. Chem. Eng. J. 371, 885–892. doi:10.1016/j.cej.2019.04.124
Zhou, X., Liu, R., Sun, K., Friedrich, D., McDowell, M. T., Yang, F., et al. (2015). Interface Engineering of the Photoelectrochemical Performance of Ni-Oxide-Coated N-Si Photoanodes by Atomic-Layer Deposition of Ultrathin Films of Cobalt Oxide. Energ. Environ. Sci. 8, 2644–2649. doi:10.1039/c5ee01687h
Keywords: bismuth vanadate, nickel oxide, photoelectrochemical, cocatalyst, water oxidation
Citation: Wang Z-Q and Wang H (2022) Fabrication of Cocatalyst NiO-Modified BiVO4 Composites for Enhanced Photoelectrochemical Performances. Front. Chem. 10:864143. doi: 10.3389/fchem.2022.864143
Received: 28 January 2022; Accepted: 18 March 2022;
Published: 02 June 2022.
Edited by:
Qingyi Zeng, University of South China, ChinaReviewed by:
Qizhao Wang, Chang’an University, ChinaCopyright © 2022 Wang and Wang. This is an open-access article distributed under the terms of the Creative Commons Attribution License (CC BY). The use, distribution or reproduction in other forums is permitted, provided the original author(s) and the copyright owner(s) are credited and that the original publication in this journal is cited, in accordance with accepted academic practice. No use, distribution or reproduction is permitted which does not comply with these terms.
*Correspondence: Zhi-Qiang Wang, WmhpcWlhbmdfV2FuZzIwMjFAMTI2LmNvbQ==
Disclaimer: All claims expressed in this article are solely those of the authors and do not necessarily represent those of their affiliated organizations, or those of the publisher, the editors and the reviewers. Any product that may be evaluated in this article or claim that may be made by its manufacturer is not guaranteed or endorsed by the publisher.
Research integrity at Frontiers
Learn more about the work of our research integrity team to safeguard the quality of each article we publish.