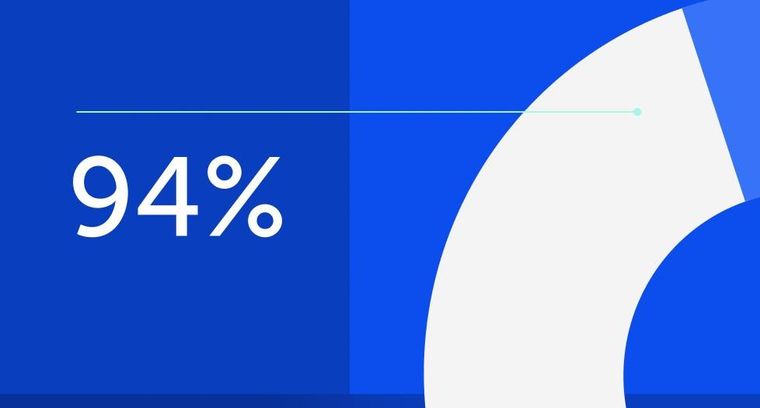
94% of researchers rate our articles as excellent or good
Learn more about the work of our research integrity team to safeguard the quality of each article we publish.
Find out more
ORIGINAL RESEARCH article
Front. Chem., 08 April 2022
Sec. Chemical Biology
Volume 10 - 2022 | https://doi.org/10.3389/fchem.2022.859822
This article is part of the Research TopicEditors’ Showcase: Chemical BiologyView all 13 articles
The amyloid-β precursor protein (APP) undergoes proteolysis by β- and γ-secretases to form amyloid-β peptides (Aβ), which is a hallmark of Alzheimer’s disease (AD). Recent findings suggest a possible role of O-glycosylation on APP’s proteolytic processing and subsequent fate for AD-related pathology. We have previously reported that Tyr681-O-glycosylation and the Swedish mutation accelerate cleavage of APP model glycopeptides by β-secretase (amyloidogenic pathway) more than α-secretase (non-amyloidogenic pathway). Therefore, to further our studies, we have synthesized additional native and Swedish-mutated (glyco)peptides with O-GalNAc moiety on Thr663 and/or Ser667 to explore the role of glycosylation on conformation, secretase activity, and aggregation kinetics of Aβ40. Our results show that conformation is strongly dependent on external conditions such as buffer ions and solvent polarity as well as internal modifications of (glyco)peptides such as length, O-glycosylation, and Swedish mutation. Furthermore, the level of β-secretase activity significantly increases for the glycopeptides containing the Swedish mutation compared to their nonglycosylated and native counterparts. Lastly, the glycopeptides impact the kinetics of Aβ40 aggregation by significantly increasing the lag phase and delaying aggregation onset, however, this effect is less pronounced for its Swedish-mutated counterparts. In conclusion, our results confirm that the Swedish mutation and/or O-glycosylation can render APP model glycopeptides more susceptible to cleavage by β-secretase. In addition, this study sheds new light on the possible role of glycosylation and/or glycan density on the rate of Aβ40 aggregation.
Alzheimer disease (AD) is one of the most common neurodegenerative disorders linked to aging (van Cauwenberghe et al., 2016; Alzheimer’s Association, 2020). It has a profound effect on the economy, health-care system, and the society, and is projected to increase even further as the population ages (Hurd et al., 2013). Genetic, biochemical, and behavioral research suggest that physiologic generation of the Aβ-forming fibrils stems from the proteolytic processing of the amyloid precursor protein (APP), a type 1 transmembrane glycoprotein, by β-secretase (BACE-1) (Hardy and Higgins, 1992; O’Brien and Wong, 2011; Selkoe and Hardy, 2016). This pathway co-exists with the nonamyloidogenic pathway, that is, initiated by α-secretase within the Aβ domain and precludes Aβ formation.
Despite the ongoing debates about the validity of amyloid cascade hypothesis, targeting amyloidogenic processing of APP is still considered a valid strategy to develop disease-modifying AD therapies (Zhao et al., 2020). New evidence continues to emerge to support the idea that deficiencies in APP trafficking and clearance of Aβ peptides is the initiating event of AD pathogenic processes (Tan and Gleeson, 2019; Zhao et al., 2020). Knowing the importance of protein glycosylation in mediating a plethora of biological functions (Krištić and Lauc, 2017; Akasaka-Manya and Manya, 2020) and considering the fact that most known AD-related molecules are either modified with glycans or play a role in glycan regulation, glycobiology may represent an interesting new insight into the understanding of AD, and a potential for new therapeutic approaches (Haukedal and Freude, 2021). The altered glycan profile of APP in the brain and cerebrospinal fluid (CSF) of AD patients versus healthy controls (Påhlsson et al., 1992; Chun et al., 2017; Boix et al., 2020; Moran et al., 2021) has been reported. Particularly, changes in O-glycosylation have been related to differences in APP processing and Aβ generation (Kitazume et al., 2010; Akasaka-Manya et al., 2017; Liu et al., 2017). APP695 is modified by a number of O-glycosylation moieties in several sites, both for mucin-linked O-glycans (O-GalNAc or N-acetylgalactosamine) and O-GlcNAc (N-acetylglucosamine) as observed in Chinese hamster ovary cells (CHO) and human CSF (Perdivara et al., 2009; Halim et al., 2011). O-GlcNAcylation has been shown to influence APP cleavage by increasing the nonamyloidogenic processing by α-secretase and reducing the secretion of Aβ in vitro and in vivo (Jacobsen and Iverfeldt, 2011; Yuzwa and Vocadlo, 2014; Chun et al., 2015, 2017). O-GalNAcylation is more abundant on APP, with extended and/or sialylated O-glycans occupying the region close to the β-secretase cleavage site (M671∼D672) of APP (Shi et al., 2021), suggesting its possible role in APP ectodomain shedding and Aβ production (Akasaka-Manya et al., 2017; Nakamura and Kurosaka, 2019). Two O-glycosylation sites, Thr663 and Ser667, located at the N-terminal side of β-secretase cleavage site have been reported to contain α-linked terminal GalNAc structure (Shi et al., 2021). Glycosylation on this region has been found to suppress the APP processing (Chun et al., 2015; Akasaka-Manya et al., 2017). In addition, a recent study has shown that the unique Tyr-O-glycosylation induces Aβ42 to form less stable fibrils, that are more susceptible towards degradation by extracellular degradation enzymes (Liu et al., 2021). The sialic acid-capped glycans, as found in the CSF samples, would likely further promote inhibition of formation of the typical β sheet-derived fibrils (Liu et al., 2021). We have previously demonstrated that a simple O-GalNAc modification on the Tyr681 residue of Aβ can induce a conformational change, provide protection from β-secretase, and slightly improve the nonamyloidogenic processing by α-secretase (Singh et al., 2021). However, in the presence of the Swedish mutation, the amyloidogenic processing by β-secretase was drastically increased (Singh et al., 2021). To date, a stimulating and inhibiting effects of glycosylation on enzyme activity have been reported (Goettig, 2016; Goth et al., 2018). Thus, a better understanding of the role of O-glycosylation on the balance between production and clearance of Aβ peptides is necessary to decipher the role of O-glycosylation in the initiating events of AD pathogenic processes.
In this study, we synthesized APP model (glyco)peptides containing the Aβ-(1–9) fragment, with extended N-terminal domain to incorporate the β-secretase cleavage site with or without the Swedish mutation (Lys670Asn/Met671Leu) and Thr663and/or Ser667 O-glycosites, respectively. These analogues were characterized for their secondary structure content using CD spectroscopy. The roles of O-glycosylation and/or Swedish mutation on proteolytic processing by β-secretase and the aggregation kinetics of Aβ40 in the absence and presence of APP (glyco)peptides were explored, respectively. Our results demonstrate a unique role of mucin-type O-glycosylation on APP’s secondary structure, proteolytic cleavage, and aggregation properties and offer an important insight into glycosylation driven changes of the intrinsic properties of APP derived peptides.
Tentagel S RAM resin was obtained from Advanced ChemTech (Louisville, KY). Fmoc-protected amino acids, and coupling reagents, 1-hydroxybenzotriazole (HOBt) and 2-(6-chloro-1H-benzotriazol-1-yl)-1,1,3,3-tetramethylaminium hexafluorophosphate (HCTU), for peptide synthesis, were purchased from Chem-Impex (Wood Dale, IL). N, N′-Diisopropylethylamine (DIPEA) was purchased from Acros Organics (Thermo Fisher Scientific, Waltham, MA). Trifluoroacetic acid (TFA), thioanisole, and all solvents (DCM, DMF, acetonitrile, and water) were of HPLC grade and purchased from Fisher Scientific (Atlanta, GA) or Sigma-Aldrich (St. Louis, MO). PBS buffer was prepared using sodium phosphate (mono- and dibasic) from Fisher Scientific (Pittsburg, PA). The O-glycosylated GalNAc building blocks of Ser 1 and Thr 2 for glycopeptide synthesis were prepared as published previously by our group (Singh et al., 2020; Beckwith et al., 2021). Recombinant human BACE-1 (rhBACE-1) and BACE-1 fluorogenic peptide substrate IV (MCA-Ser-Glu-Val-Asn-Leu-Asp-Ala-Glu-Phe-Arg-Lys(DPN)-Arg-Arg-NH2) were from R&D Systems (catalog #ES004 and #931-AS, respectively).
All peptide analogs of APP were synthesized using standard Fmoc chemistry and solid-phase peptide synthesis (SPPS) on a PS3 automated peptide synthesizer (Protein Technologies Inc., Tucson, AZ). The amino acid couplings on the synthesizer were done using a 4-fold excess of amino acids, HOBt, and HCTU, in the presence of 0.4 MN-methylmorpholine (NMM) in DMF. The Fmoc protecting group was removed using 20% piperidine in DMF. For glycopeptides, at the desired site of glycosylation, the Fmoc-protected pentafluorophenyl ester of Ser-O-GalNAc 1) and/or Thr-O-GalNAc 2) was coupled manually using a 1.5-fold excess, in the presence of DIPEA (pH 8) for 16 h. After coupling was confirmed using the ninhydrin test, the remainder of the peptide’s amino acid sequence was completed on the PS3. All the (glyco)peptides were cleaved from the resin using a TFA/thioanisole/water mixture in 95:2.5:2.5 ratio for 3 h, followed by precipitation in cold methyl-tert-butyl-ether (MTBE) to precipitate the crude (glyco)peptide. For glycopeptides, the acetylated crude was deprotected using 0.01 M NaOH solution for 15 min to remove all the O-acetyl groups on the glycan moiety attached to the peptide sequence. Lastly, the crude was lyophilized to yield the final crude deacetylated glycopeptide or its nonglycosylated counterpart.
Purification of all (glyco)peptides and their corresponding analyses were performed on a 1,260 Agilent Infinity system. The analytical RP-HPLC method uses a Phenomenex Aeris Peptide C18 column (150 mm × 4.6 mm, 3.6 μm, 100 Å) at 0.8 ml/min flow rate or a Vydac Denali C18 column (250 mm × 4.6 mm, 5 μm, 120Å) at 1 ml/min flow rate, with 0.1% TFA in water (A) and 0.1% TFA in acetonitrile (B) as the eluents. The elution gradient for analytical RP-HPLC purification was 0–60% B over 30 min. The preparative RP-HPLC method uses the Grace Vydac monomeric C18 column (250 mm × 22 mm, 15–20 μm, 300Å) at 10 ml/min flow rate, with 0.1% TFA in water (A) and 0.1% TFA in acetonitrile (B) as the eluents. The elution gradient for preparative RP-HPLC purification was 0–50% over 110 min. The (glyco)peptides were detected at 214 nm by using a UV-Vis detector (Agilent 1,260 Infinity DAD). Purified (glyco)peptides were characterized by matrix-assisted laser desorption ionization time-of-flight mass spectrometry (MALDI-TOF MS) with a Voyager-DE STR system or a Bruker Microflex system, using α-cyano-4-hydroxycinnamic acid as matrix.
All (glyco)peptides were analyzed for their secondary structure using circular dichroism (CD) spectroscopy on a Jasco-810 spectropolarimeter (Jasco, Easton, MD) in three solvent systems: water, 10 mM sodium phosphate buffer (pH 7.4), and 50% trifluoroethanol (TFE) in water (v/v) mixture. The CD spectra were recorded using a quartz cell of 1 mm optical path length over a wavelength range of 180–25 nm with a scanning speed of 100 nm/min and a response time of 4 s at 25°C. A concentration of 0.065 mg/ml, determined using the analytical RP-HPLC method, gave the lowest signal-to-noise ratio for all (glyco)peptides. All spectra were baseline-corrected to account for the signal contribution from solvent and then converted into molar ellipticity (deg cm2 dmol−1) (Sreerama and Woody, 2000). Lastly, the percentages of all secondary structures were determined using the BeStSel method (Micsonai et al., 2018; Singh et al., 2021).
All APP-based substrates were prepared as 10 mM stocks in DMSO. Before proteolysis, the activity of BACE-1 was verified by the reaction with the fluorogenic BACE-1substrate Mca-SEVNLDAEFRK(Dnp)RR-NH2 (Koike et al., 1999) as per the manufacturer’s instructions. For the proteolysis assay, APP-based substrates were diluted in BACE-1 activity buffer (0.1 M sodium acetate, pH 4.0) to the final assay concentration of 100 μM. BACE-1 was diluted to 50 nM final concentration. Reactions were incubated for 24 h at 37°C in the dark. After the incubation period, the enzyme cleavage solutions containing APP-based substrates and BACE-1 were analyzed using the analytical RP-HPLC method on the Aeris C18 column with 0.1% TFA in water (A) and 0.1% TFA in acetonitrile (B) as eluents and 0–60% B as the elution gradient over 30 min with a flow rate of 0.8 ml/min, and detection at 214 nm or the Vydac Denali C18 column with 0.1% TFA in water (A) and 0.1% TFA in acetonitrile (B) as eluents and 0–60% B as the elution gradient over 30 min with a flow rate of 1 ml/min, and detection at 214 nm. The identity of intact and cleaved (N- and C-terminal) fragments of (glyco)peptides in the absence and presence of BACE-1 (β-secretase) was confirmed by MALDI-TOF and their percentages were evaluated by the integration of the RP-HPLC peaks (averaged from two injections).
Aβ40 peptide was synthesized on a PS3 solid phase peptide synthesizer (Protein Technologies Inc., Woburn, MA) using the standard Fmoc strategy. The resulting crude peptide was purified by reversed-phase high-performance liquid chromatography (RP-HPLC) using a C18 column and characterized by matrix-assisted laser desorption ionization (MALDI) mass spectrometry. The peptide was monomerized as described previously before use (Liu et al., 2018). Lyophilized peptide powder was dissolved in aqueous NaOH solution (2 mM), and the pH was adjusted to ∼11 by using 100 mM NaOH solution. The solution was sonicated for 1 h in an ice−water bath and then filtered through a 0.22 μm filter (Millipore) and kept on ice before use. The concentration of the peptide solution was determined by using the tyrosine UV absorbance at 280 nm (ε = 1280 M−1 cm−1).
The aggregation kinetics of Aβ40 in the absence and presence of APP (glyco)peptides was performed using ThT binding assay. The monomerized Aβ40 peptide solution was diluted to a final concentration of 10 µM in 50 mM phosphate buffer (pH 7.4) and 20 µM ThT dye. For the co-incubation assays with APP (glyco)peptides, APP stock solutions of 120 µM were added to the prepared Aβ40 for final (glyco) peptide concentrations of 10 µM or 50 µM and Aβ40 concentration of 10 µM in 50 mM phosphate buffer (pH 7.4) with 20 µM ThT dye. 100 µL of each prepared solution was run in triplicate of a 96-well microplate (Costar black, clear bottom). The plate was sealed with a microplate cover and loaded into a Gemini SpectraMax EM fluorescence plate reader (Molecular Devices, Sunnyvale, CA) and incubated at 37°C. The ThT fluorescence was measured from the bottom of the plate at 10 min intervals, with 5 s of shaking, and with an excitation and emission wavelengths of 440 and 480 nm, respectively. Error bars of triplicate samples are shown for the particular data points.
AFM was employed to monitor the morphological changes of Aβ40 incubated in the absence and presence of tyrosine glycosylated (14 and 16) and nonglycosylated APP analogues (13 and 15). Aliquots (15 µL) of Aβ40 solutions were collected directly from the aggregation kinetics assay and spotted onto the surface of the freshly cleaved mica surface (5 mm × 5 mm) on solid support at room temperature. Before the measurement, the samples were covered and dried in a vacuum desiccator overnight. The AFM images were acquired in tapping mode with an area of 4 μm2, using AFM workshop TT-2 (Hilton Head Island, SC) with MikroMash NSC 15/Al BS silicon cantilevers (MikroMash, Watsonville, CA). The AFM images were further visualized and analyzed using the Gwyddion software.
The short APP (glyco) peptide fragments, part of the APP 661–680 region, were prepared. The amino acid sequence included Aβ-(1–9) DAEFRHDSG at the C-terminal end and either EISEVKM or EISEVNL (NL = Swedish mutation) at the N-terminus to incorporate the β-secretase (BACE-1) cleavage site (M ∼ D or L ∼ D). Further extension of the backbone with the additional four amino acids (IKTE), furnished a platform for site-specific O-glycosylation of Thr663 and Ser667 residues that may impact APP’s proteolytic processing due to their proximity to the BACE-1 cleavage site (Goth et al., 2018; Nakamura and Kurosaka, 2019). Hence, APP glycopeptides bearing “mucin-type” O-glycosylation, α-N-acetylgalactosamine (GalNAc), on Thr663 and/or Ser667 and their nonglycosylated counterparts were prepared using standard Fmoc-based automated solid-phase peptide chemistry (Scheme 1). For glycopeptides, the building block approach was used for the incorporation of O-glycosylated Ser 1 and/or Thr 2 in the sequence. The organic synthesis of the building blocks was achieved using our previously published protocols (Singh et al., 2020; Beckwith et al., 2021). The purity of the Ser/Thr building blocks 1 and 2, respectively, were confirmed by RP-HPLC and MALDI-TOF mass spectrometry. NMR spectra ascertained the α-linkage (Singh et al., 2020; Beckwith et al., 2021).
Automated solid-phase peptide synthesis (SPPS) approach on Tentagel S RAM resin was used to assemble the APP (glyco)peptides. For glycopeptides, the O-glycosylated Ser/Thr building blocks one and two, respectively, were manually coupled at the desired site of glycosylation, Thr663 and/or Ser667, of the growing peptide chain. After completion of the (glyco)peptide sequence, the resin was treated with trifluoroacetic acid (TFA), with water and thioanisole as scavengers. The crude acetylated glycopeptides were further deprotected under basic conditions to remove acetyl groups from the glycan moiety and obtain final deacetylated glycopeptides (4, 6, 8, 9, 11, and 12). The corresponding nonglycosylated peptides (3, 5, 7, and 10) were also prepared as above except for introducing usual Fmoc-Thr(tBu)/Ser(tBu)-OH amino acids, instead of their O-glycosylated analogs (Scheme 1). (Glyco)peptides 3–12 were obtained in high purity, as indicated by their RP-HPLC elution profiles and MALDI-TOF MS analysis (Table 1 and Supplementary Material S2–S11). As expected, the RP-HPLC analysis revealed difference in retention time (tR) between the Swedish-mutated peptide analogs and their native pairs. The Swedish-mutated (glyco)peptides 5, 6, 10–12 exhibited a 1.5 min (on average) longer tR compared to their native counterparts 3, 4, 7-9, respectively, due to increased hydrophobicity of the peptide sequence (Table 1) (Mant et al., 1989; Singh et al., 2021). In contrast, the addition of the GalNAc moiety at either Ser667 (4 and 6) or Thr663 (8 and 11) residue resulted in a decrease in the tR by 0.4 min (on average) compared to their nonglycosylated counterparts 3, 5, 7, and 10, respectively, due to increased hydrophilicity of the glycopeptide sequences (Table 1) (Singh et al., 2021). The attachment of GalNAc moiety at both glycosylation sites (12), further decreased the tR by 0.5 min compared to its monoglycosylated counterpart 11 (tR = 18.9 min, Table 1).
TABLE 1. Characterization of APP (glyco)peptides 3–16 by analytical RP-HPLC and MALDI-MSa.
To study the role of O-glycosylation on the conformation of APP glycopeptides, circular dichroism (CD) spectroscopy was used to probe the secondary structure in three different solvents, water, sodium phosphate buffer (10 mM, pH 7.4), and 50% trifluoroethanol (TFE) in water (v/v) (Figure 1). The spectra were further analysed for secondary structure estimations by Beta Structure Selection (BeStSel) method, specifically designed for the analysis of beta sheet-rich proteins (Table 2) (Micsonai et al., 2015, 2018).
FIGURE 1. Circular dichroism spectra of APP (glyco)peptides 3–12 in (A) water (B) 10 mM sodium phosphate buffer, pH 7.4, and (C) TFE/water = 1:1 (v/v) at 25°C.
TABLE 2. Summary of the secondary content (%) present in APP (glyco)peptides 3–12 determined by BeStSel for CD spectra obtained in (A) water (B) 10 mM sodium phosphate buffer, pH 7.4, and (C) TFE/water = 1:1 (v/v)a.
In water, the addition of N-terminal fragment, EISEVKM (native) or EISEVNL (Swedish-mutated), to Aβ-(1–9) in 3 and 5, respectively, exhibited characteristics of β-sheet structure that closely resembled the CD spectra of other Aβ variants in water (J. and G., 1991; Juszczyk et al., 2005; Lambermon et al., 2005). However, further extension of the N-terminal fragment with the additional four amino acids (IKTE) in native 7 and Swedish-mutated 10, respectively, increased the overall hydrophilicity of Aβ-(1–9) and caused a conformation shift from β-sheet to random coil (Figure 1A). This agrees with our previous work where addition of the N-terminal fragment, IKTEEISEVKM (native), to β-sheet-forming Aβ-(1–23) peptide in 13, was also largely disordered (Singh et al., 2021). Deconvolution of the spectra revealed that native peptide 3 had the highest amount of β-structure (85.2%, anti-parallel β-sheet, parallel β-sheet, and β-turn), with the majority being anti-parallel β-sheet (59.9%), and the remaining being α-helix (14.8%) (Table 2). The presence of the Swedish double-mutation in 5, resulted in an increase in α-helix and decrease in the β-structure amounts (34.5 and 57.8%, respectively). The distribution of α-helix and β-structure content in five is similar to the Swedish-mutated Aβ-(1–23) peptide 15, (Juszczyk et al., 2005; Singh et al., 2021). CD spectra clearly indicates that regardless of the Swedish mutation, addition of the N-terminal fragment, IKTEEISEVKM or IKTEEISEVNL, to Aβ-(1–9) significantly increased the percentage of random coil in 7 and 10 (85.1 and 90.7%, respectively). Site-specific O-glycosylation of Ser667 in glycopeptides 4 and 6 caused their structure to be largely disordered (87.1% and 41.4%, respectively) with complete loss of parallel β-sheets compared to their nonglycosylated counterparts 3 and 5, respectively (Figure 1A). This effect was more pronounced for the native glycopeptide 4 where β-structure along with α-helix was significantly reduced (12.9% and 0%, respectively). On the other hand, the Swedish-mutated glycopeptide 6 was less prone to change in its β-structure and α-helix amounts (39.4% and 19.2%, respectively) (Table 2). Also, the site-specific O-glycosylation of Thr663 in glycopeptides 8 and 11 did not significantly change the secondary conformation compared to their nonglycosylated counterparts 7 and 10, respectively, that were already largely disordered in water (85–90%). Hence, we were able to confirm that the addition of a single GalNAc moiety in APP 661–694 derived glycopeptides is able to break ordered secondary structures and cause it to be disordered in water (Singh et al., 2021). Interestingly, by increasing the glycan valency in glycopeptides 9 and 12, the amount β-structure increased (64.8 and 55.0%, respectively) and random coil decreased (22.4 and 27.0%, respectively). Therefore, glycan valency is an important determinant of the secondary structure of APP glycopeptides in water.
To evaluate secondary structure in a more physiologically relevant buffer setting, the CD spectra of (glyco)peptides were recorded in sodium phosphate buffer of low ionic strength (10 mM, pH 7.4). As expected, the (glyco)peptides indicated the presence of an unfolded state and were partially disordered with some β-structure properties. Deconvolution of the spectra revealed a prominent presence of random coil (25–77%) and antiparallel β-sheet (17–48%), followed by β-turn (7–34%), and lastly, α-helix (0–10%) (Figure 1B and Table 2) (Hortschansky et al., 2005; Tew et al., 2008; Singh et al., 2021). The Swedish-mutated diglycosyated peptide 12 showed the highest amount of antiparallel β-sheet (47.9%) whereas its monoglycosylated counterpart 11 had the highest amount of random coil (76.8%) in this solvent system. Notable, in the absence of parallel β-sheets, the ratio of the remaining structural elements varied depending on the modifications (Swedish mutation and/or glycosylation) incorporated into the peptide sequences (Figure 1B and Table 2). These findings are in agreement with the previously reported CD data for Aβ peptides of different length; the Aβ-(1–42) peptide exhibited slightly higher α-helix and random coil content compared to Aβ-(1–16) peptide that has higher β-sheet and β-turn content (Tew et al., 2008). Likewise, our previously reported (glyco)peptides containing longer Aβ-(1–23) fragment (13–16) showed higher α-helix and random coil, and lower antiparallel β-sheet content, in sodium phosphate buffer (Singh et al., 2021) compared to (glyco)peptides analysed in this study that contain shorter Aβ-(1–9) fragment (3–12). Hence, Aβ occurs in various isoforms that differ by the number of residues at the C-terminal end of the peptides, which impacts the secondary structural preferences of the peptides in solution.
APP is an integral membrane protein whose behaviour can be modified by molecules such as trifluoroethanol (TFE) that partition the membrane-water interface and change the physiochemical properties of the lipid bilayer (Barry and Gawrisch, 1994; Özdirekcan et al., 2008). We have previously investigated the effects of TFE on a molecular level using CD spectroscopy to obtain an account of the α-helix-forming potential of model (glyco)peptides 13–16 containing the Tyr681 O-linked glycosylation in Aβ-(1–23) region of APP (Singh et al., 2021). Upon addition of TFE into water (1:1, v/v), the (glyco)peptides showed a significant increase in α-helix and random coil content and decrease in β-structure content (Figure 1C). Upon further analysis, shorter native peptide 3 had slightly higher α-helix content (66.2%) than its Swedish-mutated counterpart 5 (59.9%), however, this difference was larger between the longer native peptide 7 (88.7%) and its Swedish-mutated counterpart 10 (36.8%) (Table 2). Regardless of the length of Aβ fragment, the Swedish mutation significantly reduced the amount of α-helix and increased β-sheet and random coil secondary structure elements in this solvent system. Similarly, site-specific O-glycosylation of Thr663 and Tyr681 (Singh et al., 2021) slightly decreased the α-helix and increased the random coil content in native glycopeptides, 8 and 14 respectively, whereas it increased α-helix and decreased random coil content in Swedish-mutated glycopeptides, 11 and 16 respectively, with this effect being more pronounced for 16. Lastly, regardless of the Swedish mutation, the attachment of two GalNAc moieties on Thr663 and Ser667 within the peptide sequence drastically increased the α-helix content in 9 and 12 (94.4% and 86.2%, respectively), in this solvent system. These findings suggest that in membrane-mimicking conditions, excess O-glycosylation can hamper the effects of the Swedish mutation on secondary structure and prompt it to become largely α-helical.
Glycosylation can alter substrate recognition and impact enzyme activity in either a positive (enhancing) or negative (inhibiting) manner (Goettig, 2016; Goth et al., 2018). The mucin-type O-linked glycosylation of a protein may not only affect its conformation but also affect its transport and localization in the cell (Matsuura et al., 1989; Chen et al., 2002). Proteases are highly regulated by post-translational modifications and drive fate, localization, and activity of many proteins (Bond, 2019). Certain mutations can also affect the subcellular localization of the cleavage event by crucial proteases and mediate a different cellular mechanism for the protein (Haass et al., 1995). We have previously reported that the Swedish mutation is an important criterion for enhancing both ADAM10 (α-secretase) and BACE1 (β-secretase) cleavage rates of Aβ-(1–23) model (glyco)peptides 13–16, where site-specific mucin-type O-linked glycosylation of Tyr681 residue in 16 further increased BACE-1 driven amyloid pathway (Singh et al., 2021). Therefore, similar enzyme cleavage assays with BACE-1 were set up to explore the effect of the Swedish mutation, length of the amino acid sequence, glycan position and valency on the proteolytic susceptibility of 3–12. BACE1 produced two fragments upon cleavage of (glyco)peptides, for which yields were determined after 24 h treatment. The yields of intact peptide and fragments were evaluated by the RP-HPLC peaks integration (Table 3, and the Supplementary Material S20–S32).
TABLE 3. Proteolytic cleavage of APP (glyco)peptides 3–12 upon treatment with BACE-1 enzyme (KM∼D/NL∼D cleavage site)a.
Regardless of glycosylation, the native Aβ-(1–9) model (glyco)peptides 3 and 4 showed almost full recovery after incubation with BACE-1 (95.5% and 98.6%, respectively). The extension of the native (glyco)peptide sequence at the N-terminus with IKTEEISEVKM in 7 and 8 also resulted in a very low BACE-1 proteolysis, 3.4% and 8.5%, respectively. Increasing the glycan valency to two, in native glycopeptide 9, did not significantly alter cleavage rates (95.9% recovery). It became evident that the presence of the Swedish mutation in five was a driving force for the near complete cleavage of the peptide (96.2%). Interestingly, the extension of the peptide sequence at the N-terminus with IKTEEISEVNL in 10 showed a lower amount of the peptide cleaved (79.7%) in comparison to shorter peptide fragment 5. Thus, a longer N-terminal fragment flanking the Aβ region can hamper its proteolytic susceptibility and result in slower cleavage rates by BACE-1. Glycosylation had a more significant effect on the sequence carrying the Swedish mutation, where regardless of the N-terminal fragment length, glycan position and valency, BACE-1 treatment resulted in 93–98% cleaved products for glycopeptides 6 and 11–12. This agrees with our previous findings that apart from the Swedish mutation, the presence of O-glycosylation can drive BACE-1 cleavage rates and result in increased Aβ production (Singh et al., 2021).
Other key aspects affecting BACE-1 cleavage rates in the presence of the Swedish mutation were the length of the C-terminal Aβ fragment and the glycan position relative to the cleavage site. For example, 16 contains Aβ-(1–23) C-terminal fragment and is cleaved to a much lesser extent (42.4%) by BACE-1 than 11 that contains Aβ-(1–9) C-terminal fragment (98.1%). Moreover, the relative position of the GalNAc moiety on Thr663 and Tyr681 in 11 and 16, respectively, can also influence enzymatic activity. Even though 16 is cleaved to a lesser extent than 11 by BACE-1, the ∼3-fold increase in cleaved products compared to its non-glycosylated counterpart 15 is observed. This further points to a stronger effect of Tyr681 O-glycosylation on the C-terminal Aβ fragment in accelerating BACE-1 activity. Therefore, we can postulate that in the presence of the Swedish mutation, excess O-glycosylation proximal to BACE-1 cleavage site can significantly increase the cleavage propensity of the peptides for the amyloid pathway, with this effect being more pronounced when the glycosylation is on the C-terminal Aβ side of the cleavage site.
The subcellular localization of BACE-1 cleavage of Swedish-mutated APP differs greatly from that for native APP, where the former is localized to a post Golgi compartment for Aβ generation and outcompetes anti-amyloidogenic processing by α-secretases (Haass et al., 1995; Thinakaran et al., 1996). However, little is known regarding the regulatory role of mucin-type O-linked glycosylation of APP on BACE-1 activity, and these results are particularly interesting, since Aβ peptides in CSF of AD patients are heavily glycosylated by mucin-type O-linked glycans (Halim et al., 2011). Thus, we can speculate that both Swedish mutation and mucin-type O-linked glycosylation increase APP processing because the former provides a better cleavage site for BACE-1, and the latter changes the conformation and increases the sensitivity of the protein to BACE-1.
We adopted the widely used thioflavin T (ThT) assay (Xue et al., 2017) to investigate how O-glycosylation impacts the kinetics of fiber formation of Aβ40, a model peptide for studying the dynamics of protein aggregation. The aggregation kinetic profile of Aβ40 peptide (10 μM) exhibited a typical sigmoidal curve with three different regions: a lag phase associated with nucleation, a rapid growth phase for elongation and polymerization by fibrils, and a final saturation phase dominated with mature fibrils (Figure 2, Supplementary Material S33–S40). At the conditions used in this study, it has been shown that Aβ amyloidogenesis proceeds by a nucleation-dependent polymerization mechanism that involves key soluble oligomeric intermediates (Du et al., 2011; Elbassal et al., 2017). The half time (t50) of the growth phase of the Aβ40 amyloidogenesis was approximately 8 h (Figure 2, Supplementary Material S33–S40), where t50 is defined as the time at which the fluorescence intensity reaches the midpoint between the pre- and post-aggregation baselines.
FIGURE 2. Effect of APP peptides 3, 5, 7, 10, 13, and 15 (A) and glycopeptides 4, 6, 8, 9, 11, 12, 14, 16 (B) on the aggregation kinetics of Aβ40 (10 µM) using ThT fluorescence assay in phosphate buffer (50 mM, pH 7.4) at 37°C. The concentration of (glyco)peptides was 10 and 50 µM aPeptide 13 was run at 10 and 25 µM *Aggregation half-time (Δt50) = APP (glyco)peptide t50—Aβ40 t50. The t50 values are means of triplicate kinetics results. Alongside are tapping mode atomic force microscopy images of Aβ40 fibril growth upon 24 h incubation with nonglycosylated peptides, 13 and 15 (A) and Tyr-O-glycopeptides, 14 and 16 (B).
Although recent studies have implicated small soluble oligomers, as the main culprits of Aβ toxicity and AD pathogenesis (Yang et al., 2017), very little is known about the exact mechanism of oligomeric assembly and the conformation of peptides in this early event of Aβ aggregation. In the presence of APP (glyco) peptides 3–16 at two concentrations (10 and 50 µM), the kinetics of aggregation of Aβ40 was described by a sigmoidal curve, with a lag phase that varied depending on the internal modifications of the (glyco)peptides such as the Swedish mutation, site-specific O-glycosylation, glycan valency and/or sequence length. The curves reached a plateau after approximately 24 h (Supplementary Material S33–S40). The nonglycosylated peptides 3, 5, and 7 displayed no marked effect either on the lag phase (Δt50 <2 h, Figure 2A) or the final ThT fluorescence intensity in comparison to control Aβ40 peptide alone (Supplementary Figures S1A–S3A, Supplementary Material S33–S35). Interestingly, we observed a slight delay in the aggregation process by the Swedish-mutated peptide 10 (Δt50 = 3.2 h at 50 μM, Figure 2A, Supplementary Figure S4A, Supplementary Material S36) with extended N-terminal domain. Increasing the C-terminal domain sequence in 13 and 15, to include Aβ-(1–23) fragment, led to a much larger effect on the lag phase (Supplementary Figures S5A, S6A, Supplementary Material S38–S39), with Δt50 = 7.1 h for 13 (25 µM) and Δt50 = 5.2 h for 15 (50 µM) (Figure 2A), indicating an inhibitory effect on Aβ40 aggregation. Notably, we observed a complete saturation of the ThT signal for native peptide 13 at higher concentration (50 µM) (Supplementary Figures S5A,B, Supplementary Material S38). Hence, the aggregation of Aβ40 in the presence of nonglycosylated peptides 3, 5, 7, 10, 13, or 15 displayed a longer lag phase when the length of the Aβ fragment was increased. The effect of the Swedish mutation was less clear and varied regardless of peptide length.
To evaluate Aβ40 aggregation in the presence of glycopeptides, we first performed kinetics with the free GalNAc sugar (10 and 50 µM) that showed minimal difference in Δt50 values (Δt50 <0.5 h, Figure 2B). The presence of a single GalNAc moiety on Ser667 in native glycopeptide four increased the lag phase substantially (Δt50 = 6.7 h at 50 μM, Figure 2B, and Supplementary Figure S1B in the Supplementary Material S33) and delayed Aβ40 aggregation much more compared to its non-glycosylated counterpart 3. Extension of the N-terminal domain sequence in native glycopeptide eight by IKTE, slightly decreased the Δt50 value (Δt50 = 5 h at 50 μM, Figure 2B, and Supplementary Figure S3B in the Supplementary Material S35) compared to four. However, increasing the glycan valency in nine resulted in gain in inhibition of Aβ40 aggregation and further increase of the lag phase (Δt50 = 7.7 h at 50 μM, Figure 2B, Supplementary Figure S3C, Supplementary Material S36). Regardless of the length of the N-terminal domain sequence, the presence of the Swedish mutation in 6 and 11 completely suppressed the inhibitory effect of O-glycosylation on Aβ40 aggregation exhibited by 8 (Δt50 = 0 h at 50 μM, Figure 2B, and Supplementary Figure S2B, S4B in the Supplementary Material S34,S37). A drastic increase in the Δt50 value for diglycosylated and Swedish mutated peptide 12 (Δt50 = 7.8 h at 50 μM, Figure 2B, and Supplementary Figure S4C in the Supplementary Material S37) clearly indicated that O-glycosylation at multiple sites of attachment can overcome the effect of the Swedish mutation and cause Aβ40 to aggregate at slower rates. Consistent with their nonglycosylated versions 13 and 15, the extension of the Aβ fragment and site-specific O-glycosylation of Tyr681 in 14 and 16 showed the largest difference in lag phase and strongest inhibition of Aβ40 aggregation profile, with Δt50 = 12.1 h for 14 (50 µM) and Δt50 = 15.2 h for 16 (50 µM) (Figure 2B, and Supplementary Figures S5C, S6B in the Supplementary Material S39–S40). Along with having a prolonged lag phase, 14 also reduces the final ThT fluorescence intensity dramatically (∼50%), suggesting a significant interference in Aβ40 aggregation. Therefore, our results suggest that O-glycosylation inhibits Aβ40 aggregation in a concentration-dependent manner, and this effect is more pronounced when the glycopeptides contain the longer Aβ-(1–23) fragment and GalNAc modification on Tyr681(14 and 16). It is also important to mention that by reducing the length of the Aβ fragment to Aβ-(1–9), we were able to detect the key differences between the Swedish-mutated (6 and 11) and mono-/diglycosylated analogues (4, 8, 9, and 12) on Aβ40 aggregation kinetics.
The morphology of the tyrosine (glyco) peptide aggregates (13–16) co-incubated with Aβ40 was examined using atomic force microscopy (AFM). In the absence of (glyco)peptides, Aβ40 forms a dense meshwork of amyloid fibrils (Figure 2). In the presence of native peptide 13 (25 μM) lower density of Aβ40 fibrils is observed (Figure 2A). Similarly, addition of glycosylated counterpart 14 (50 μM), resulted in less fibrils formed, and the ones formed were shorter in size (Figure 2B). This is consistent with the ThT kinetics data for 13 and 14 at 25 and 50 μM, respectively. The addition of the Swedish-mutated peptide 15 (50 µM) to Aβ40 resulted in the fibrilar morphology similar to that of Aβ40 alone (Figure 2A). However, its glycosylated counterpart 16 was able to partially inhibit the fibril formation, resulting in thinly dispersed and less dense Aβ40 fibril (Figure 2B). Thus, as observed in ThT kinetics, the aggregation of Aβ40 can be delayed by 15 and 16 (50 µM), however, the inhibitory potency is not enough to prevent fibril formation under the current conditions.
Our work parallels and directly complements the study by Liu et al., 2021 that shows Aβ42 peptides bearing Tyr681 O-glycosylation significantly affect both the aggregation and degradation of Aβ42. Furthermore, similar inhibiting activity of Aβ40 and Aβ42 fibrillogenesis by glycation (Emendato et al., 2018; Milordini et al., 2020) and addition of polysaccharides, such as chitosan (Liu et al., 2015; Hao et al., 2017) and heparin sulfate (Wang et al., 2021) was reported.
In summary, the dynamical interplay between O-glycosylation and aggregation affected the structure of peptides and slowed down the aggregation process. The presence of the Swedish mutation led to an increased amount of β-structure in physiological conditions, with the β-secretase activity being drastically increased, and the aggregation process remaining largely unaffected. However, this effect of the Swedish mutation on the (glyco)peptides was overcome by increasing the number of glycosylation sites near the β-secretase cleavage site, increasing the C-terminal domain (Aβ) sequence relative to the β-secretase cleavage site, and/or having Tyr681 glycosylated in the Aβ domain, resulting in glycosylation strongly inhibiting the aggregation process of Aβ40. Therefore, our studies demonstrate that O-glycosylation typically supports the non-amyloidogenic processing of APP, however, in FAD cases, it can incline towards the amyloidogenic processing of APP, where its fate lies upon the abundance and position of O-glycans relative to the β-secretase cleavage site.
The original contributions presented in the study are included in the article/Supplementary Material, further inquiries can be directed to the corresponding author.
YS and MC wrote the manuscript. YS, DO, and RA conducted the synthesis of the glycosylated Thr/Ser building blocks. Synthesis of glycopeptides was done by YS, NV, GM and DO. The CD analysis was done by YS and NV. Proteolytic cleavage assay was done by DM and samples analyzed by YS, GM, and NV. Aggregation kinetics assays and AFM images acquired and analyzed by DR and DD. The senior authorship is shared by DM, DD, and MC.
This research was supported by the National Institute of Health (NIH) Grants R15CA242351 to MC, R15CA249788 to DM, and R15GM116006 to DD, the Alzheimer’s Association AARG-17-531423 to DD, and Palm Health Foundation and Stiles-Nicholson Brain Institute to MC.
The authors declare that the research was conducted in the absence of any commercial or financial relationships that could be construed as a potential conflict of interest.
All claims expressed in this article are solely those of the authors and do not necessarily represent those of their affiliated organizations, or those of the publisher, the editors and the reviewers. Any product that may be evaluated in this article, or claim that may be made by its manufacturer, is not guaranteed or endorsed by the publisher.
The authors are thankful to Dr. Vivian Merk (FAU) and her lab for their atomic force microscopy system that was used in this study.
The Supplementary Material for this article can be found online at: https://www.frontiersin.org/articles/10.3389/fchem.2022.859822/full#supplementary-material
Akasaka-Manya, K., Kawamura, M., Tsumoto, H., Saito, Y., Tachida, Y., Kitazume, S., et al. (2017). Excess APPO-Glycosylation by GalNAc-T6 Decreases Aβ Production. J. Biochem. 161, 99–111. doi:10.1093/jb/mvw056
Akasaka-Manya, K., and Manya, H. (2020). The Role of APP O-Glycosylation in Alzheimer's Disease. Biomolecules 10, 1569. doi:10.3390/biom10111569
Alzheimer’s Association (2020). 2020 Alzheimer's Disease Facts and Figures. Alzheimers Dement 16, 391–460. doi:10.1002/alz.12068
Barry, J. A., and Gawrisch, K. (1994). Direct NMR Evidence for Ethanol Binding to the Lipid-Water Interface of Phospholipid Bilayers. Biochemistry 33, 8082–8088. doi:10.1021/bi00192a013
Beckwith, D. M., FitzGerald, F. G., Rodriguez Benavente, M. C., Mercer, E. R., Ludwig, A.-K., Michalak, M., et al. (2021). Calorimetric Analysis of the Interplay between Synthetic Tn Antigen-Presenting MUC1 Glycopeptides and Human Macrophage Galactose-type Lectin. Biochemistry 60, 547–558. doi:10.1021/acs.biochem.0c00942
Boix, C. P., Lopez-Font, I., Cuchillo-Ibañez, I., and Sáez-Valero, J. (2020). Amyloid Precursor Protein Glycosylation Is Altered in the Brain of Patients with Alzheimer's Disease. Alz Res. Ther. 12, 96. doi:10.1186/s13195-020-00664-9
Bond, J. S. (2019). Proteases: History, Discovery, and Roles in Health and Disease. J. Biol. Chem. 294, 1643–1651. doi:10.1074/jbc.TM118.004156
Chen, P.-Y., Lin, C.-C., Chang, Y.-T., Lin, S.-C., and Chan, S. I. (2002). One O-Linked Sugar Can Affect the Coil-To-β Structural Transition of the Prion Peptide. Proc. Natl. Acad. Sci. U.S.A. 99, 12633–12638. doi:10.1073/pnas.192137799
Chun, Y. S., Kwon, O.-H., and Chung, S. (2017). O-GlcNAcylation of Amyloid-β Precursor Protein at Threonine 576 Residue Regulates Trafficking and Processing. Biochem. Biophysical Res. Commun. 490, 486–491. doi:10.1016/j.bbrc.2017.06.067
Chun, Y. S., Kwon, O.-H., Oh, H. G., Kim, T.-W., McIntire, L. B., Park, M. K., et al. (2015). Threonine 576 Residue of Amyloid-β Precursor Protein Regulates its Trafficking and Processing. Biochem. Biophysical Res. Commun. 467, 955–960. doi:10.1016/j.bbrc.2015.10.037
Du, D., Murray, A. N., Cohen, E., Kim, H.-E., Simkovsky, R., Dillin, A., et al. (2011). A Kinetic Aggregation Assay Allowing Selective and Sensitive Amyloid-β Quantification in Cells and Tissues. Biochemistry 50, 1607–1617. doi:10.1021/bi1013744
Elbassal, E. A., Morris, C., Kent, T. W., Lantz, R., Ojha, B., Wojcikiewicz, E. P., et al. (2017). Gold Nanoparticles as a Probe for Amyloid-β Oligomer and Amyloid Formation. J. Phys. Chem. C 121, 20007–20015. doi:10.1021/acs.jpcc.7b05169
Emendato, A., Milordini, G., Zacco, E., Sicorello, A., Dal Piaz, F., Guerrini, R., et al. (2018). Glycation Affects Fibril Formation of Aβ Peptides. J. Biol. Chem. 293, 13100–13111. doi:10.1074/jbc.RA118.002275
Goettig, P. (2016). Effects of Glycosylation on the Enzymatic Activity and Mechanisms of Proteases. Ijms 17, 1969–1993. doi:10.3390/ijms17121969
Goth, C. K., Vakhrushev, S. Y., Joshi, H. J., Clausen, H., and Schjoldager, K. T. (2018). Fine-tuning Limited Proteolysis: A Major Role for Regulated Site-specific O -Glycosylation. Trends Biochem. Sci. 43, 269–284. doi:10.1016/j.tibs.2018.02.005
Haass, C., Lemere, C. A., Capell, A., Citron, M., Seubert, P., Schenk, D., et al. (1995). The Swedish Mutation Causes Early-Onset Alzheimer's Disease by β-secretase Cleavage within the Secretory Pathway. Nat. Med. 1, 1291–1296. doi:10.1038/nm1295-1291
Halim, A., Brinkmalm, G., Rüetschi, U., Westman-Brinkmalm, A., Portelius, E., Zetterberg, H., et al. (2011). Site-specific Characterization of Threonine, Serine, and Tyrosine Glycosylations of Amyloid Precursor Protein/amyloid β-peptides in Human Cerebrospinal Fluid. Proc. Natl. Acad. Sci. U.S.A. 108, 11848–11853. doi:10.1073/pnas.1102664108
Hao, C., Wang, W., Wang, S., Zhang, L., and Guo, Y. (2017). An Overview of the Protective Effects of Chitosan and Acetylated Chitosan Oligosaccharides against Neuronal Disorders. Mar. Drugs 15, 89. doi:10.3390/md15040089
Hardy, J. A., and Higgins, G. A. (1992). Alzheimer's Disease: The Amyloid Cascade Hypothesis. Science 256, 184–185. doi:10.1126/science.1566067
Haukedal, H., and Freude, K. K. (2021). Implications of Glycosylation in Alzheimer’s Disease. Front. Neurosci. 14, 1432. doi:10.3389/fnins.2020.625348
Hortschansky, P., Schroeckh, V., Christopeit, T., Zandomeneghi, G., and Fändrich, M. (2005). The Aggregation Kinetics of Alzheimer's β-amyloid Peptide Is Controlled by Stochastic Nucleation. Protein Sci. : A Publication Protein Soc. 14, 1753–1759. doi:10.1110/ps.041266605
Hurd, M. D., Martorell, P., Delavande, A., Mullen, K. J., and Langa, K. M. (2013). Monetary Costs of Dementia in the United States. N. Engl. J. Med. 368, 1326–1334. doi:10.1056/NEJMsa1204629
Jacobsen, K. T., and Iverfeldt, K. (2011). O-GlcNAcylation Increases Non-amyloidogenic Processing of the Amyloid-β Precursor Protein (APP). Biochem. Biophysical Res. Commun. 404, 882–886. doi:10.1016/j.bbrc.2010.12.080
Juszczyk, P., Kołodziejczyk, A. S., and Grzonka, Z. (2005). Circular Dichroism and Aggregation Studies of Amyloid Beta (11-8) Fragment and its Variants. Acta Biochim. Pol. 52, 425–431. doi:10.18388/abp.2005_3455
Kitazume, S., Tachida, Y., Kato, M., Yamaguchi, Y., Honda, T., Hashimoto, Y., et al. (2010). Brain Endothelial Cells Produce Amyloid β from Amyloid Precursor Protein 770 and Preferentially Secrete the O-Glycosylated Form. J. Biol. Chem. 285, 40097–40103. doi:10.1074/jbc.M110.144626
Koike, H., Seki, H., Kouchi, Z., Ito, M., Kinouchi, T., Tomioka, S., et al. (1999). Thiment Oligopeptidase Cleaves the Full-Length Alzheimer Amyloid Precursor Protein at a β-Secretase Cleavage Site in COS Cells. J. Biochem. 126, 235–242. doi:10.1093/oxfordjournals.jbchem.a022428
Krištić, J., and Lauc, G. (2017). “Ubiquitous Importance of Protein Glycosylation,” in High-Throughput Glycomics and Glycoproteomics: Methods and Protocols. Editors G. Lauc, and M. Wuhrer (New York, NY: Springer New York), 1–12. doi:10.1007/978-1-4939-6493-2_1
Lambermon, M. H. L., Rappaport, R. V., and McLaurin, J. (2005). Biophysical Characterization of Longer Forms of Amyloid Beta Peptides: Possible Contribution to Flocculent Plaque Formation. J. Neurochem. 95, 1667–1676. doi:10.1111/j.1471-4159.2005.03497.x
Liu, D., Wei, Q., Xia, W., He, C., Zhang, Q., Huang, L., et al. (2021). O-glycosylation Induces Amyloid-β to Form New Fibril Polymorphs Vulnerable for Degradation. J. Am. Chem. Soc. 143, 20216–20223. doi:10.1021/jacs.1c08607
Liu, F., Xu, K., Xu, Z., de las Rivas, M., Wang, C., Li, X., et al. (2017). The Small Molecule Luteolin Inhibits N-Acetyl-α-Galactosaminyltransferases and Reduces Mucin-type O-Glycosylation of Amyloid Precursor Protein. J. Biol. Chem. 292, 21304–21319. doi:10.1074/jbc.M117.814202
Liu, H., Morris, C., Lantz, R., Kent, T. W., Elbassal, E. A., Wojcikiewicz, E. P., et al. (2018). Residue‐Specific Dynamics and Local Environmental Changes in Aβ40 Oligomer and Fibril Formation. Angew. Chem. Int. Ed. 57, 8017–8021. doi:10.1002/anie.201802490
Liu, H., Ojha, B., Morris, C., Jiang, M., Wojcikiewicz, E. P., Rao, P. P. N., et al. (2015). Positively Charged Chitosan and N-Trimethyl Chitosan Inhibit Aβ40 Fibrillogenesis. Biomacromolecules 16, 2363–2373. doi:10.1021/acs.biomac.5b00603
Mant, C. T., Zhou, N. E., and Hodges, R. S. (1989). Correlation of Protein Retention Times in Reversed-phase Chromatography with Polypeptide Chain Length and Hydrophobicity. J. Chromatogr. A 476, 363–375. doi:10.1016/S0021-9673(01)93882-8
Matsuura, H., Greene, T., and Hakomori, S. (1989). An α-N-Acetylgalactosaminylation at the Threonine Residue of a Defined Peptide Sequence Creates the Oncofetal Peptide Epitope in Human Fibronectin. J. Biol. Chem. 264, 10472–10476. doi:10.1016/S0021-9258(18)81645-3
Micsonai, A., Wien, F., Bulyáki, É., Kun, J., Moussong, É., Lee, Y.-H., et al. (2018). BeStSel: A Web Server for Accurate Protein Secondary Structure Prediction and Fold Recognition from the Circular Dichroism Spectra. Nucleic Acids Res. 46, W315–W322. doi:10.1093/nar/gky497
Micsonai, A., Wien, F., Kernya, L., Lee, Y.-H., Goto, Y., Réfrégiers, M., et al. (2015). Accurate Secondary Structure Prediction and Fold Recognition for Circular Dichroism Spectroscopy. Proc. Natl. Acad. Sci. U.S.A. 112, E3095–E3103. doi:10.1073/pnas.1500851112
Milordini, G., Zacco, E., Percival, M., Puglisi, R., Dal Piaz, F., Temussi, P., et al. (2020). The Role of Glycation on the Aggregation Properties of IAPP. Front. Mol. Biosci. 7, 104. doi:10.3389/fmolb.2020.00104
Moran, F. P., Daniel, S. M., Chen, R., Michaela, Y., Shai, Z., Ehud, G., et al. (2021). Interplay between Protein Glycosylation Pathways in Alzheimer’s Disease. Sci. Adv. 3, e1601576. doi:10.1126/sciadv.1601576
Nakamura, N., and Kurosaka, A. (2019). Mucin-type Glycosylation as a Regulatory Factor of Amyloid Precursor Protein Processing. J. Biochem. 165, 205–208. doi:10.1093/jb/mvy121
O'Brien, R. J., and Wong, P. C. (2011). Amyloid Precursor Protein Processing and Alzheimer's Disease. Annu. Rev. Neurosci. 34, 185–204. doi:10.1146/annurev-neuro-061010-113613
Özdirekcan, S., Nyholm, T. K. M., Raja, M., Rijkers, D. T. S., Liskamp, R. M. J., and Killian, J. A. (2008). Influence of Trifluoroethanol on Membrane Interfacial Anchoring Interactions of Transmembrane α-Helical Peptides. Biophysical J. 94, 1315–1325. doi:10.1529/biophysj.106.101782
Påhlsson, P., Shakin-Eshleman, S. H., and Spitalnik, S. L. (1992). N-linked Glycosylation of β-amyloid Precursor Protein. Biochem. Biophysical Res. Commun. 189, 1667–1673. doi:10.1016/0006-291X(92)90269-Q
Perdivara, I., Petrovich, R., Allinquant, B., Deterding, L. J., Tomer, K. B., and Przybylski, M. (2009). Elucidation of O-Glycosylation Structures of the β-Amyloid Precursor Protein by Liquid Chromatography−Mass Spectrometry Using Electron Transfer Dissociation and Collision Induced Dissociation. J. Proteome Res. 8, 3786. doi:10.1021/pr9001096
Selkoe, D. J., and Hardy, J. (2016). The Amyloid Hypothesis of Alzheimer's Disease at 25 Years. EMBO Mol. Med. 8, 595–608. doi:10.15252/emmm.201606210
Shi, J., Ku, X., Zou, X., Hou, J., Yan, W., and Zhang, Y. (2021). Comprehensive Analysis of O-Glycosylation of Amyloid Precursor Protein (APP) Using Targeted and Multi-Fragmentation MS Strategy. Biochim. Biophys. Acta (Bba) - Gen. Subjects 1865, 129954. doi:10.1016/j.bbagen.2021.129954
Singh, Y., Ormaza, D., Massetti, A., Minond, D., and Cudic, M. (2021). Tyrosine O-GalNAc Alters the Conformation and Proteolytic Susceptibility of APP Model Glycopeptides. ACS Chem. Neurosci. 12, 2974–2980. doi:10.1021/acschemneuro.1c00387
Singh, Y., Rodriguez Benavente, M. C., Al-Huniti, M. H., Beckwith, D., Ayyalasomayajula, R., Patino, E., et al. (2020). Positional Scanning MUC1 Glycopeptide Library Reveals the Importance of PDTR Epitope Glycosylation for Lectin Binding. J. Org. Chem. 85, 1434–1445. doi:10.1021/acs.joc.9b02396
Sreerama, N., and Woody, R. W. (2000). Estimation of Protein Secondary Structure from Circular Dichroism Spectra: Comparison of CONTIN, SELCON, and CDSSTR Methods with an Expanded Reference Set. Anal. Biochem. 287, 252–260. doi:10.1006/abio.2000.4880
Tan, J. Z. A., and Gleeson, P. A. (2019). The Role of Membrane Trafficking in the Processing of Amyloid Precursor Protein and Production of Amyloid Peptides in Alzheimer's Disease. Biochim. Biophys. Acta (Bba) - Biomembranes 1861, 697–712. doi:10.1016/j.bbamem.2018.11.013
Tew, D. J., Bottomley, S. P., Smith, D. P., Ciccotosto, G. D., Babon, J., Hinds, M. G., et al. (2008). Stabilization of Neurotoxic Soluble β-Sheet-Rich Conformations of the Alzheimer's Disease Amyloid-β Peptide. Biophysical J. 94, 2752–2766. doi:10.1529/biophysj.107.119909
Thinakaran, G., Teplow, D. B., Siman, R., Greenberg, B., and Sisodia, S. S. (1996). Metabolism of the "Swedish" Amyloid Precursor Protein Variant in Neuro2a (N2a) Cells. J. Biol. Chem. 271, 9390–9397. doi:10.1074/jbc.271.16.9390
Van Cauwenberghe, C., Van Broeckhoven, C., and Sleegers, K. (2016). The Genetic Landscape of Alzheimer Disease: Clinical Implications and Perspectives. Genet. Med. 18, 421–430. doi:10.1038/gim.2015.117
Wang, P., Zhao, J., Hossaini Nasr, S., Otieno, S. A., Zhang, F., Qiang, W., et al. (2021). Probing Amyloid β Interactions with Synthetic Heparan Sulfate Oligosaccharides. ACS Chem. Biol. 16, 1894–1899. doi:10.1021/acschembio.0c00904
Xue, C., Lin, T. Y., Chang, D., and Guo, Z. (2017). Thioflavin T as an Amyloid Dye: Fibril Quantification, Optimal Concentration and Effect on Aggregation. R. Soc. Open Sci. 4, 160696. doi:10.1098/rsos.160696
Yang, T., Li, S., Xu, H., Walsh, D. M., and Selkoe, D. J. (2017). Large Soluble Oligomers of Amyloid β-Protein from Alzheimer Brain Are Far Less Neuroactive Than the Smaller Oligomers to Which They Dissociate. J. Neurosci. 37, 152–163. doi:10.1523/JNEUROSCI.1698-16.2016
Yuzwa, S. A., and Vocadlo, D. J. (2014). O-GlcNAc and Neurodegeneration: Biochemical Mechanisms and Potential Roles in Alzheimer's Disease and beyond. Chem. Soc. Rev. 43, 6839–6858. doi:10.1039/C4CS00038B
Keywords: Alzheimer’s disease, circular dichroism, proteolysis, aggregation kinetics, APP O-glycopeptides
Citation: Singh Y, Regmi D, Ormaza D, Ayyalasomayajula R, Vela N, Mundim G, Du D, Minond D and Cudic M (2022) Mucin-Type O-Glycosylation Proximal to β-Secretase Cleavage Site Affects APP Processing and Aggregation Fate. Front. Chem. 10:859822. doi: 10.3389/fchem.2022.859822
Received: 21 January 2022; Accepted: 07 March 2022;
Published: 08 April 2022.
Edited by:
John D. Wade, University of Melbourne, AustraliaReviewed by:
Matthew Robert Pratt, University of Southern California, United StatesCopyright © 2022 Singh, Regmi, Ormaza, Ayyalasomayajula, Vela, Mundim, Du, Minond and Cudic. This is an open-access article distributed under the terms of the Creative Commons Attribution License (CC BY). The use, distribution or reproduction in other forums is permitted, provided the original author(s) and the copyright owner(s) are credited and that the original publication in this journal is cited, in accordance with accepted academic practice. No use, distribution or reproduction is permitted which does not comply with these terms.
*Correspondence: Maré Cudic, bWN1ZGljQGZhdS5lZHU=
Disclaimer: All claims expressed in this article are solely those of the authors and do not necessarily represent those of their affiliated organizations, or those of the publisher, the editors and the reviewers. Any product that may be evaluated in this article or claim that may be made by its manufacturer is not guaranteed or endorsed by the publisher.
Research integrity at Frontiers
Learn more about the work of our research integrity team to safeguard the quality of each article we publish.