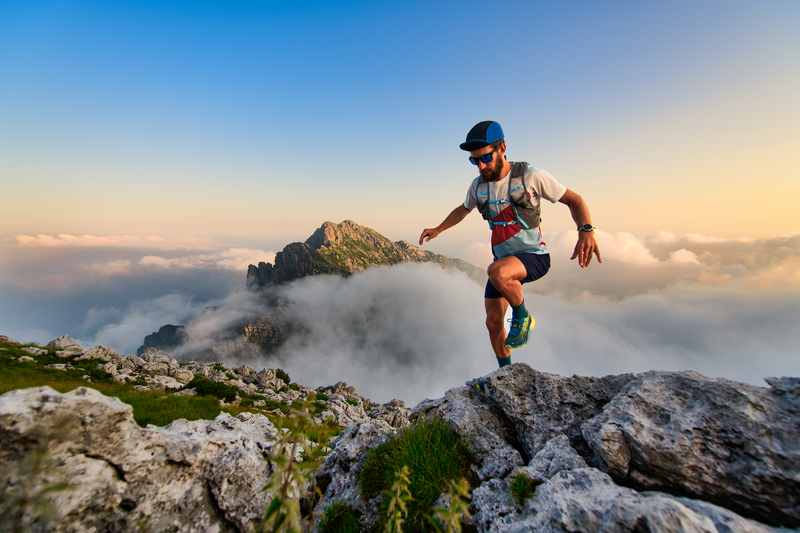
95% of researchers rate our articles as excellent or good
Learn more about the work of our research integrity team to safeguard the quality of each article we publish.
Find out more
ORIGINAL RESEARCH article
Front. Chem. , 08 June 2022
Sec. Chemical Physics and Physical Chemistry
Volume 10 - 2022 | https://doi.org/10.3389/fchem.2022.854918
This article is part of the Research Topic Solvation Effects of Organic Reactions in Ionic Liquids, Deep Eutectic Solvents, and Conventional Solvents View all 5 articles
Few kinetic parameters, or reaction rates, are known up to date in detail about 1-chloro and 1-fluoro-2,4-dinitrobenzene (ClDNB and FDNB, respectively) with a series of biothiols in aqueous media. These biological nucleophiles with thiol groups have been widely used as a reference in nucleophile reactivity assays due to their prevalence and cellular abundance. The main aim of this study was to elucidate the reaction mechanism based on Brönsted-type plots and reactivity patterns of the electrophile/nucleophile pairs. A complete kinetic study was performed in terms of the comparison of Brönsted-type slope parameters (βnuc) for the reactions and was used for assigning the mechanism and the rate-determining step associated with the reaction route. A mass spectrometry analysis demonstrated that the nucleophilic center of the biothiols is the -SH group and there is only one kinetic product. The kinetic study suggests that the reaction mechanism might be the borderline between concerted and stepwise pathways. An amine–enol equilibrium for the most reactive nucleophiles appears to be the main determining factor controlling the nucleophilic attack in the nucleophilic aromatic substitution reactions investigated, highlighting the anionic form for these nucleophiles. This amine–enol equilibrium involves a hydrogen bond which stabilizes the intermediate species in the reaction pathway. Thus, intramolecular bonds are formed and enhance the nucleophilic strength through the contribution of the solvent surrounding the electrophile/nucleophile pairs. Finally, we highlight the importance of the formation of electrophile/nucleophile adducts that could modify structures and/or functions of biological systems with potential toxic effects. Therefore, it is essential to know all these kinetic and reactivity patterns and their incidence on other studies.
Electrophiles are often potential substrates that develop adducts in a critical step of pathogenic processes, which are initiated by the exposure of these chemicals to biological nucleophiles (Aptula et al., 2005; Schultz et al., 2006; Campodónico and Contreras, 2008). The reactions between electrophiles and biological nucleophiles have early been studied by Coles, who hypothesized that the reactions of these species could have toxic effects by the formation of electrophile/nucleophile (E+/Nu) adducts and modify structures and/or functions of proteins, deoxyribonucleic acid (DNA), or ribonucleic acid (RNA) (Coles, 1984; LoPachin et al., 2009). Electrophilicity and nucleophilicity concepts are based on the general acid–base theory of Brönsted and Lowry (Lowry, 1923) and the valence electron theory of Lewis (Lewis, 1923), where E+ and Nu− correspond to electron-deficient and electron-rich species (Ingold, 1929; Ingold, 1933; Ingold, 1934). The activities of substrates and biological targets depend on the reactivity patterns of E+/Nu− pairs and their reaction mechanisms (Carlson, 1990). The most recurrent reactions of these E+/Nu− pairs correspond to Michael reactions, nucleophilic substitutions (NS), and nucleophilic aromatic substitutions (SNAr) among others (Aptula et al., 2005; Schultz et al., 2006). Biological nucleophiles such as biothiols are involved in many cellular functions and human diseases (Seshadri et al., 2002). These molecules have a thiol (–SH) group in their chemical structure. The most known biothiol is the tripeptide glutathione (GSH). Despite the importance of biological processes involving biothiols, only fragments of fundamental physical–chemical aspects are well understood.
In order to investigate one of these types of reactions (SNAr), the main aim of this work was to show that kinetic studies can be used to better understand the mechanism which is derived from reactions of known substrates: 1-chloro and 1-fluoro-2,4-dinitrobenzene (ClDNB and FDNB, respectively) with a series of biothiols in aqueous media (see Figure 1) (Ormazábal-Toledo et al., 2013a; Alarcón-Espósito et al., 2015, 2017; Sánchez et al., 2018a, 2018b). Biological nucleophiles with the thiol group have been widely used as a reference in nucleophile reactivity assays due to their prevalence and cellular abundance (Roberts et al., 2007; Schwöbel et al., 2011). ClDNB and FDNB compounds are classified by the structural alert (SA) such as i) SA_27 (nitro aromatic) and ii) SA_31a (halogenated benzene) in the compilation of chemical linked to carcinogenicity and mutagenicity (Ashby and Tennant, 1988; Worth et al., 2007; Benigni and Bossa, 2011). However, only few kinetic parameters or reaction rates for these systems are known in detail. In this study, kinetic results are discussed in terms of the comparison of Brönsted-type slope parameters (
1-Chloro and 1-fluoro-2,4-dinitrobenzene and all the biothiols were of the highest quality available such as commercial products by Merck and Sigma Aldrich. The certificate of analysis guarantees purity was ≥99%.
The kinetics of the reactions were performed spectrophotometrically (λ = 336 nm) using a diode array spectrophotometer in aqueous and buffer phosphate solutions at 25.0 and 37.0 ± 0.1°C, ionic strength 0.2 M (KCl) for aqueous media at three different pH values maintained by partial protonation of the biothiols (pH =
where
This was operated in a negative mode. Accurate mass spectra were recorded from 100 to 550 m/z. For the fragmentation study, a data-dependent scan was performed using the electrospray ionization mode with an AB Sciex Triple Quad 4500 mode. A computer was equipped with Analyst software, version 1.6.2, handled data analysis. The compounds from the reaction between substrate ClDNB and the nucleophiles: GSH and S-methyl glutathione (Me–GSH) were identified by their corresponding spectral characteristics, accurate mass, mass spectra, and feature fragmentation. This analysis supports the existence of a kinetic product at 474 m/z followed at 336 nm in a negative mode. So, from a mass spectrometry analysis it is possible to assign one chemical structure to each m/z ratio. It is worth noting that the reaction products for reactions between ClDNB and FDNB with biothiol series will be the same or similar. Hence, in this analysis only ClDNB was considered.
Figure 2A considers the fragmentation patterns associated with the reaction between GSH with ClDNB. The chemical structure of GSH in Figure 2A shows the possible nucleophilic centers located on N- (a, b) and S- (c) groups denoted by arrows. Note that, this is a general chemical structure of GSH, and it does not consider the protonation states. Figure 2A shows four strong signals: 182.8, 237, 305.9, and 473. One of the most important signals corresponds to m/z = 473, which was assigned to the kinetic product (see Figure 3). The fluctuation of m/z between 471 and 473 could be attributed to different protonation states of the kinetic product. However, the reaction product may be oriented toward those three positions (a, b, or c), but position b might be discarded by steric hindrance. However, the most important reason is the chemical nature of b position; it is an amide, which is a weak nucleophilic center due to resonance effects with the carbonyl group. Thus, the possibilities of nucleophilic attack should be a and c oriented to SH- or amino (NH2-) groups in the chemical structure of GSH. In order to determine the reaction center in GHS, the mass analysis of the reaction between Me–GSH with ClDNB (see Figure 2B below) was performed. In contrast, Figure 2B shows no signal attributed to the kinetic product (m/z = 473). The chemical structure of Me–GSH in Figure 2B shows it is blocked in the c position by a methyl group. Therefore, the only nucleophilic center on GHS able to react with ClDNB will be c position, the SH- group. (See Figures 2A, 3).
FIGURE 2. (A) Mass/charge ratio for the reaction between GSH with ClDNB in aqueous media. (B) Mass/charge ratio for the reaction between Me–GSH with ClDNB in aqueous media.
FIGURE 3. Chemical structure associated with mass/charge ratio for the reaction product between GSH and ClDNB.
Product authentication was performed by a complete mass spectroscopy analysis which suggested the presence of a series of compounds from the reacting pair in aqueous media. Figure 2 shows the mass spectrum for the reaction between ClDNB and GHS (Figure 2A) and Me–GSH (Figure 2B), respectively.
Figure 4 shows the possible compounds derived from the studied reactions. Figure 2A shows another three strong signals: 182.8, 237.0, and 305.9 and Figure 2B shows three strong signals: 182.8, 261.9, and 320.0. The signals at 305.9 (Figure 2A) and 320.0 (Figure 2B) might be attributed to GSH and Me–GSH, respectively. As reaction conditions are pseudo-first-order, the concentration of nucleophile is almost 10 times more concentrated in comparison to the substrate. Figure 2A shows a signal located at 237.0, which is not shown in Figure 2B. At the same time, Figure 2B shows a signal located at 261.9, which is not shown in Figure 2A. So, these signals might be attributed to decomposition products from the reacting pair where the signal located at 237.0 is attributed to compound III and the signal located at 261.9 to compound I. Finally, both spectra have only one common signal located at 182.8. This might be associated to a decomposition product from the GHS and/or Me–GSH named compound II.
FIGURE 4. Chemical structures associated with mass/charge ratio for the reaction between ClDNB and GSH and Me–GSH.
Under the experimental conditions used, only one product formation was spectrophotometrically observed for all the reactions which displayed an increase of a band centered in the range of 330–550 nm and was attributed to the corresponding reaction product for all nucleophiles studied (see Figure 3). Therefore, the possibility of a nucleophilic attack at the unsubstituted ring positions of the substrate is discarded (Um et al., 2007; Gabsi et al., 2018).
The SNAr process is well documented in the literature as a stepwise mechanism (
FIGURE 5. General reaction mechanism for a SNAr between 1-halogen-2,4-dinitrobenzene with a biothiol in the anionic form.
Considering the established
Note that, the
TABLE 1. Nucleophilic rate constant values for the reaction between ClDNB with biothiol series in aqueous media and phosphate solution at 25°C and 37°C.
The
TABLE 2. Nucleophilic rate constant values for the reaction between FDNB with biothiol series in aqueous media at 25°C.
Another mechanistic route might be a concerted pathway (
A preliminary inspection of Tables 1, 2 shows that reactivity patterns of the nucleophiles in aqueous media as reaction media toward ClDNB and FDNB increased in the following order: N-acetyl cysteine > Glutathione > Cysteine > L-cysteine ethyl ester. This order agrees with the basicity of the sulfhydryl group in biothiol (
FIGURE 6. Acid–base equilibria related to general aminothiol in the pH range between 6 and 12.
TABLE 3. Macroscopic constants for each equilibrium (
The following analysis is based on the kinetic response and its possible relationships with the macroscopic constants. Then, the most nucleophilic biothiols toward the substrates correspond to N-acetylcysteine (see Tables 1, 2 in the text) suggesting that
Note that the most reactive nucleophiles (N-acetylcysteine and glutathione) have an amide (R2-N-(CO)-R) group in their chemical structures, which might establish amine–enol equilibrium (see Figure 7 below). Then, the tautomeric equilibrium may be stabilizing the thiolate form enhancing their reactivities (see Figure 7). On the other hand, homocysteine, cysteine, and L-cysteine ethyl ester compounds cannot establish the amine–enol equilibrium mentioned before, which reinforces it has a key role in the reactivity patterns (see Tables 1, 2 in the text and see Figure 1) for N-acetylcysteine and glutathione. Therefore, the amine–enol equilibrium appears as the main determining factor controlling the nucleophilic attack in a SNAr reaction. The tautomeric equilibrium is discussed based on the reactivity patterns given by the kinetic data over the reacting pairs. However, this analysis can be reinforced with the aid of computational and theoretical studies. Considering N-acetylcysteine as a reference nucleophile (Table 2 in this work) toward FDNB; the ratio with ethanolamine (PA) is 976 times and 17.5 times to piperazine (SAA). Then, N-acetylcysteine is more reactive than other nucleophiles of similar
The Brönsted-type plots are shown in Supplementary Figures S25–S29 for each reaction studied. A Brönsted type-plot corresponds to a free energy relationship that correlates the logarithm of the nucleophilic rate coefficients and the
where
Then,
We present a complete kinetic study based on SNAr reactions. The Brönsted type-plots analysis of two known substrates with a series of biothiols suggest a concerted or borderline stepwise mechanism, where the amine–enol equilibrium established by N-acetylcysteine and glutathione toward these substrates appears as the main determining factor controlling the reactivity patterns toward a SNAr reaction. This tautomeric form is associated with the chemical structure of these biothiols and hydrogen bonds from the aqueous media might be stabilizing the anionic form of the nucleophile and/or promoting the hydrogen departure from the -SH group and enhancing the nucleophilic strength toward the substrates. In addition, a complete product analysis suggests that the thiol group is the nucleophilic center discarding the amine group. Finally, it is relevant to highlight that some biological processes would be conditioned by reactivity patterns of the E+/Nu− pairs involved in the reaction, which are demonstrated through their kinetic rates and reaction pathways.
The original contributions presented in the study are included in the article/Supplementary Material; further inquiries can be directed to the corresponding author.
PC designed the experiments, analyzed results, wrote and revised the manuscript. JA-E performed the kinetic experiments and worked in the manuscript. BO performed some kinetic data and worked in the manuscript. All the authors have approved the final revised manuscript. PC and on behalf of Collaborative Working Group.
This project was supported by a FONDECYT grant 1150759 and Instituto de Ciencias e Innovación en Medicina, Facultad de Medicina, Clínica Alemana Universidad del Desarrollo.
The authors declare that the research was conducted in the absence of any commercial or financial relationships that could be construed as a potential conflict of interest.
All claims expressed in this article are solely those of the authors and do not necessarily represent those of their affiliated organizations, or those of the publisher, the editors, and the reviewers. Any product that may be evaluated in this article, or claim that may be made by its manufacturer, is not guaranteed or endorsed by the publisher.
The Supplementary Material for this article can be found online at: https://www.frontiersin.org/articles/10.3389/fchem.2022.854918/full#supplementary-material
Alarcón-Espósito, J., Tapia, R. A., Contreras, R., and Campodónico, P. R. (2015). Changes in the SN Ar Reaction Mechanism Brought about by Preferential Solvation. RSC Adv. 5, 99322–99328. Available at: https://pubs.rsc.org/en/content/articlehtml/2015/ra/c5ra20779g. doi:10.1039/c5ra20779g
Alarcón-Espósito, J., Contreras, R., and Campodónico, P. R. (2017). Iso-solvation Effects in Mixtures of Ionic Liquids on the Kinetics of a Model SNAr Reaction. New J. Chem. 41, 13435–13441. doi:10.1039/c7nj03246c
Alvaro, C. E. S., Ayala, A. D., and Nudelman, N. S. (2011). Hydrogen-Bonded Nucleophile Effects in ANS: the Reactions of 1-chloro and 1-Fluoro-2,4-Dinitrobenzene with 2-guanidinobenzimidazole, 1-(2-aminoethyl)piperidine andN-(3-Aminopropyl)morpholine in Aprotic Solvents. J. Phys. Org. Chem. 24, 101–109. doi:10.1002/poc.1712
Aptula, A. O., Patlewicz, G., and Roberts, D. W. (2005). Skin Sensitization: Reaction Mechanistic Applicability Domains for Structure−Activity Relationships. Chem. Res. Toxicol. 18, 1420–1426. doi:10.1021/tx050075m
Ashby, J., and Tennant, R. W. (1988). Chemical Structure, Salmonella Mutagenicity and Extent of Carcinogenicity as Indicators of Genotoxic Carcinogenesis Among 222 Chemicals Tested in Rodents by the U.S. NCI/NTP. Mutat. Res./Genetic Toxicol. 204, 17–115. doi:10.1016/0165-1218(88)90114-0
Banjoko, O., and Babatunde, I. A. (2004). Rationalization of the Conflicting Effects of Hydrogen Bond Donor Solvent on Nucleophilic Aromatic Substitution Reactions in Non-polar Aprotic Solvent: Reactions of Phenyl 2,4,6-trinitrophenyl Ether with Primary and Secondary Amines in Benzene-Methanol Mixtures. Tetrahedron 60, 4645–4654. Available at: https://www.sciencedirect.com/science/article/pii/S0040402004004909. doi:10.1016/j.tet.2004.03.07
Benesch, R. E., and Benesch, R. (1955). The Acid Strength of the -SH Group in Cysteine and Related Compounds. J. Am. Chem. Soc. 77, 5877–5881.
Benigni, R., and Bossa, C. (2011). Mechanisms of Chemical Carcinogenicity and Mutagenicity: a Review with Implications for Predictive Toxicology. Chem. Rev. 111, 2507–2536. doi:10.1021/cr100222q
Bernasconi, C. F., and De Rossi, R. H. (1976). Influence of the O-Nitro Group on Base Catalysis in Nucleophilic Aromatic Substitution. Reactions in Benzene Solution. J. Org. Chem. 41, 44–49. doi:10.1021/jo00863a010
Brönsted, J. N., and Pedersen, K. (1924). Die katalytische Zersetzung des Nitramids und ihre physikalisch-chemische Bedeutung. Z. Phys. Chem. 108U, 185–235. doi:10.1515/zpch-1924-10814
Brønsted, J. N. (1923). Some Remarks on the Concept of Acids and Bases. Recl. Trav. Chim. Pays-Bas 42, 718–728.
Buncel, E., Tarkka, R., and Hoz, S. (1993). The Phenomenology of Differently Constructed Brønsted-type Plots. J. Chem. Soc. Chem. Commun., 109–110. doi:10.1039/C39930000109
Calfumán, K., Gallardo-Fuentes, S., Contreras, R., Tapia, R. A., and Campodónico, P. R. (2017). Mechanism for the SNAr Reaction of Atrazine with Endogenous Thiols: Experimental and Theoretical Study. New J. Chem. 41, 12671–12677. doi:10.1039/c7nj02708g
Campodónico, P. R., and Contreras, R. (2008). Structure-reactivity Relationships for Electrophilic Sugars in Interaction with Nucleophilic Biological Targets. Bioorg. Med. Chem. 16, 3184–3190. doi:10.1016/j.bmc.2007.12.018
Campodónico, P. R., Olivares, B., and Tapia, R. A. (2020). Experimental Analyses Emphasize the Stability of the Meisenheimer Complex in a SNAr Reaction toward Trends in Reaction Pathways. Front. Chem. 8, 583. doi:10.3389/fchem.2020.00583
Campodónico, P. R., Tapia, R. A., and Suárez-Rozas, C. (2022). How the Nature of an Alpha-Nucleophile Determines a Brønsted Type-Plot and its Reaction Pathways. An Experimental Study. Front. Chem. 9, 740161. doi:10.3389/fchem.2021.740161
Carlson, R. M. (1990). Assessment of the Propensity for Covalent Binding of Electrophiles to Biological Substrates. Environ. Health Perspect. 87, 227–232. doi:10.1289/ehp.9087227
Castro, E. A., Aliaga, M., Campodónico, P., and Santos, J. G. (2002). Kinetics and Mechanism of the Aminolysis of Methyl 4-nitrophenyl, Methyl 2,4-dinitrophenyl, and Phenyl 2,4-dinitrophenyl Carbonates. J. Org. Chem. 67, 8911–8916. doi:10.1021/jo026390k
Castro, E. A. (1999). Kinetics and Mechanisms of Reactions of Thiol, Thiono, and Dithio Analogues of Carboxylic Esters with Nucleophiles. Chem. Rev. 99, 3505–3524. doi:10.1021/cr990001d
Coles, B. (1984). Effects of Modifying Structure on Electrophilic Reactions with Biological Nucleophiles. Drug Metab. Rev. 15, 1307–1334. doi:10.3109/03602538409029962
Crampton, M. R., Emokpae, T. A., Howard, J. A. K., Isanbor, C., and Mondal, R. (2004). Leaving Group Effects on the Mechanism of Aromatic Nucleophilic Substitution (SNAr) Reactions of Some Phenyl 2,4,6-trinitrophenyl Ethers with Aniline in Acetonitrile. J. Phys. Org. Chem. 17, 65–70. doi:10.1002/poc.690
Crampton, M. R., Emokpae, T. A., Emokpae, T., and Isanbor, C. (2006). Effects of Ortho ‐and para‐Ring Activation on the Kinetics of SNAr Reactions of 1‐Chloro‐2‐nitro‐and 1‐Phenoxy‐2‐nitrobenzenes with Aliphatic Amines in AcetonitrileEur. J. Org. Chem., 1222–1230. Available at: https://ir.unilag.edu.ng/handle/123456789/4629. doi:10.1002/ejoc.200500774
Gabsi, W., Essalah, K., Goumont, R., Tangour, B., and Boubaker, T. (2018). The Ambident Electrophilic Behavior of 5-Nitro-3-X-Thiophenes in σ-complexation Processes. Int. J. Chem. Kinet. 50, 659–669. doi:10.1002/kin.21190
Gallardo-Fuentes, S., and Ormazábal-Toledo, R. (2019). σ-Holes Promote the Concertedness in Nucleophilic Aromatic Substitution Reactions of Nitroarenes. New J. Chem. 43, 7763–7769. doi:10.1039/C9NJ01493D
Gallardo-Fuentes, S., Tapia, R. A., Contreras, R., and Campodónico, P. R. (2014). Site Activation Effects Promoted by Intramolecular Hydrogen Bond Interactions in SNAr Reactions. RSC Adv. 4, 30638–30643. doi:10.1039/c4ra04725g
García-Beltrán, O., Mena, N., Pérez, E. G., Cassels, B. K., Nuñez, M. T., Werlinger, F., et al. (2011). The Development of a Fluorescence Turn-On Sensor for Cysteine, Glutathione and Other Biothiols. A Kinetic Study. Tetrahedron Lett. 52, 6606–6609. doi:10.1016/j.tetlet.2011.09.137
García-Beltrán, O., Santos, J. G., Fuentealba, S., De la Torre, P., Pavez, P., Mena, N., et al. (2015). Mechanism Study of the Thiol-Addition Reaction to Benzothiazole Derivative for Sensing Endogenous Thiols. Tetrahedron Lett. 56, 2437–2440. doi:10.1016/j.tetlet.2015.03.083
Gazitúa, M., Tapia, R. A., Contreras, R., and Campodónico, P. R. (2014). Mechanistic Pathways of Aromatic Nucleophilic Substitution in Conventional Solvents and Ionic Liquids. New J. Chem. 38, 2611–2618. doi:10.1039/C4NJ00130C
Glossman-Mitnik, D., and Maciejewska, M. (2020). Solvent Effect on a Model of SNAr Reaction in Conventional and Non-Conventional Solvents. Solvents, Ionic Liquids and Solvent Effects. Editor Campodónico Paola R. (BoD – Books on Demand), Chapter 12. Available at: https://play.google.com/store/books/details?id=7kr9DwAAQBAJ. doi:10.5772/intechopen.82039
Ingold, C. K. (1929). The Principles of Aromatic Substitution, from the Standpoint of the Electronic Theory of Valency. Recl. Trav. Chim. Pays-Bas. 48, 797–812. Available at: https://onlinelibrary.wiley.com/doi/abs/10.1002/recl.19290480808. doi:10.1002/recl.19290480808
Ingold, C. K. (1933). 266. Significance of Tautomerism and of the Reactions of Aromatic Compounds in the Electronic Theory of Organic Reactions. J. Chem. Soc., 1120–1127. doi:10.1039/jr9330001120
Ingold, C. K. (1934). Principles of an Electronic Theory of Organic Reactions. Chem. Rev. 15, 225–274. doi:10.1021/cr60051a003
Jencks, W. P., and Gilchrist, M. (1968). Nonlinear Structure-Reactivity Correlations. The Reactivity of Nucleophilic Reagents toward Esters. J. Am. Chem. Soc. 90, 2622–2637. doi:10.1021/ja01012a030
Kwan, E. E., Zeng, Y., Besser, H. A., and Jacobsen, E. N. (2018). Concerted Nucleophilic Aromatic Substitutions. Nat. Chem. 10, 917–923. doi:10.1038/s41557-018-0079-7
Lewis, G. N. (1923). Valence and the Structure of Atoms and Molecules. Incorporated: Chemical Catalog Company.
Lin, W., Yuan, L., Cao, Z., Feng, Y., and Long, L. (2009). A Sensitive and Selective Fluorescent Thiol Probe in Water Based on the Conjugate 1,4-Addition of Thiols to α,β-Unsaturated Ketones. Chem. Eur. J. 15, 5096–5103. doi:10.1002/chem.200802751
LoPachin, R. M., Gavin, T., Petersen, D. R., and Barber, D. S. (2009). Molecular Mechanisms of 4-Hydroxy-2-Nonenal and Acrolein Toxicity: Nucleophilic Targets and Adduct Formation. Chem. Res. Toxicol. 22, 1499–1508. doi:10.1021/tx900147g
Lowry, T. M. (1923). The Uniqueness of Hydrogen. J. Chem. Technol. Biotechnol. 42, 43–47. doi:10.1002/jctb.5000420302
Mortier, J. (2015). Theoretical and experimental Methods for the Analisys of Reaction Mechanisms in SNAr Processed: Fugality, Philicity and Solvent Effects. Arene Chemistry: Reaction Mechanisms and Methods for Aromatic Compounds, Editor Contreras Renato, Campodónico Paola R., and Ormazábal-Toledo Rodrigo (John Wiley & Sons), Chapter 7, 175. Available at: https://play.google.com/store/books/details?id=ffBbCwAAQBAJ.
Neumann, C. N., and Ritter, T. (2017). Facile C-F Bond Formation through a Concerted Nucleophilic Aromatic Substitution Mediated by the PhenoFluor Reagent. Acc. Chem. Res. 50, 2822–2833. doi:10.1021/acs.accounts.7b00413
Neumann, C. N., Hooker, J. M., and Ritter, T. (2016). Concerted Nucleophilic Aromatic Substitution with (19)F(-) and (18)F(-). Nature 534, 369–373. doi:10.1038/nature17667
Newington, I., Perez-Arlandis, J. M., and Welton, T. (2007). Ionic Liquids as Designer Solvents for Nucleophilic Aromatic Substitutions. Org. Lett. 9, 5247–5250. doi:10.1021/ol702435f
Nudelman, N. S., Mancini, P. M. E., Martinez, R. n. D., and Vottero, L. R. (1987). Solvents Effects on Aromatic Nucleophilic Substitutions. Part 5. Kinetics of the Reactions of 1-Fluoro-2,4-Dinitrobenzene with Piperidine in Aprotic Solvents. J. Chem. Soc. Perkin Trans. 2, 951–954. doi:10.1039/P29870000951
Ormazábal-Toledo, R., Contreras, R., and Campodónico, P. R. (2013a). Reactivity Indices Profile: a Companion Tool of the Potential Energy Surface for the Analysis of Reaction Mechanisms. Nucleophilic Aromatic Substitution Reactions as Test Case. J. Org. Chem. 78, 1091–1097. doi:10.1021/jo3025048
Ormazábal-Toledo, R., Contreras, R., Tapia, R. A., and Campodónico, P. R. (2013b). Specific Nucleophile-Electrophile Interactions in Nucleophilic Aromatic Substitutions. Org. Biomol. Chem. 11, 2302–2309. doi:10.1039/C3OB27450K
Roberts, D. W., Patlewicz, G., Kern, P. S., Gerberick, F., Kimber, I., Dearman, R. J., et al. (2007). Mechanistic Applicability Domain Classification of a Local Lymph Node Assay Dataset for Skin Sensitization. Chem. Res. Toxicol. 20, 1019–1030. doi:10.1021/tx700024w
Sánchez, B., Calderón, C., Garrido, C., Contreras, R., and Campodónico, P. R. (2018a). Solvent Effect on a Model SN Ar Reaction in Ionic Liquid/water Mixtures at Different Compositions. New J. Chem. 42, 9645–9650. Available at: https://pubs.rsc.org/en/content/articlehtml/2018/nj/c7nj04820c. doi:10.1039/c7nj04020c
Sánchez, B., Calderón, C., Tapia, R. A., Contreras, R., and Campodónico, P. R. (2018b). Activation of Electrophile/Nucleophile Pair by a Nucleophilic and Electrophilic Solvation in a SNAr Reaction. Front. Chem. 6, 509. doi:10.3389/fchem.2018.00509
Sardi, F., Manta, B., Portillo-Ledesma, S., Knoops, B., Comini, M. A., and Ferrer-Sueta, G. (2013). Determination of Acidity and Nucleophilicity in Thiols by Reaction with Monobromobimane and Fluorescence Detection. Anal. Biochem. 435, 74–82. doi:10.1016/j.ab.2012.12.017
Satterthwait, A. C., and Jencks, W. P. (1974). Mechanism of the Aminolysis of Acetate Esters. J. Am. Chem. Soc. 96, 7018–7031. doi:10.1021/ja00829a034
Schultz, T. W., Carlson, R. E., Cronin, M. T. D., Hermens, J. L. M., Johnson, R., O’Brien, P. J., et al. (2006). A Conceptual Framework for Predicting the Toxicity of Reactive Chemicals: Modeling Soft Electrophilicity. SAR QSAR Environ. Res. 17, 413–428. doi:10.1080/10629360600884371
Schwöbel, J. A. H., Koleva, Y. K., Enoch, S. J., Bajot, F., Hewitt, M., Madden, J. C., et al. (2011). Measurement and Estimation of Electrophilic Reactivity for Predictive Toxicology. Chem. Rev. 111, 2562–2596. doi:10.1021/cr100098n
Seshadri, S., Beiser, A., Selhub, J., Jacques, P. F., Rosenberg, I. H., D'Agostino, R. B., et al. (2002). Plasma Homocysteine as a Risk Factor for Dementia and Alzheimer's Disease. N. Engl. J. Med. 346, 476–483. doi:10.1056/NEJMoa011613
Terrier, F. (2013). Modern Nucleophilic Aromatic Substitution. John Wiley & Sons. Available at: https://play.google.com/store/books/details?id=Eic2TIjkCgsC.
Thomas, D. (1974). in The Proton in Chemistry. Editor R. P. Bell. 2nd edition (London: Chapman & Hall), 310. 1973. E6.30. Endeavour 33, 157. doi:10.1016/0160-9327(74)90046-5
Um, I.-H., Min, S.-W., and Dust, J. M. (2007). Choice of Solvent (MeCN vs H2O) Decides Rate-Limiting Step in SNAr Aminolysis of 1-Fluoro-2,4-Dinitrobenzene with Secondary Amines: Importance of Brønsted-type Analysis in Acetonitrile. J. Org. Chem. 72, 8797–8803. doi:10.1021/jo701549h
Um, I.-H., Im, L.-R., Kang, J.-S., Bursey, S. S., and Dust, J. M. (2012). Mechanistic Assessment of SNAr Displacement of Halides from 1-Halo-2,4-Dinitrobenzenes by Selected Primary and Secondary Amines: Brønsted and Mayr Analyses. J. Org. Chem. 77, 9738–9746. doi:10.1021/jo301862b
Um, I.-H., Kim, M.-Y., Kang, T.-A., and Dust, J. M. (2014). Kinetic Study on SNAr Reaction of 1-(y-Substituted-Phenoxy)-2,4-Dinitrobenzenes with Cyclic Secondary Amines in Acetonitrile: Evidence for Cyclic Transition-State Structure. J. Org. Chem. 79, 7025–7031. doi:10.1021/jo5011872
Worth, A. P., Bassan, A., De Bruijn, J., Gallegos Saliner, A., Netzeva, T., Pavan, M., et al. (2007). The Role of the European Chemicals Bureau in Promoting the Regulatory Use of (Q)SAR Methods. SAR QSAR Environ. Res. 18, 111–125. doi:10.1080/10629360601054255
Keywords: SNAr reactions, reaction mechanism, border mechanisms, biothiols, reactivity patterns
Citation: Campodónico PR, Alarcón-Espósito J and Olivares B (2022) Kinetics and Reaction Mechanism of Biothiols Involved in SNAr Reactions: An Experimental Study. Front. Chem. 10:854918. doi: 10.3389/fchem.2022.854918
Received: 14 January 2022; Accepted: 25 April 2022;
Published: 08 June 2022.
Edited by:
Kenneth K. Laali, University of North Florida, United StatesReviewed by:
Giovanni Finoto Caramori, Federal University of Santa Catarina, BrazilCopyright © 2022 Campodónico, Alarcón-Espósito and Olivares. This is an open-access article distributed under the terms of the Creative Commons Attribution License (CC BY). The use, distribution or reproduction in other forums is permitted, provided the original author(s) and the copyright owner(s) are credited and that the original publication in this journal is cited, in accordance with accepted academic practice. No use, distribution or reproduction is permitted which does not comply with these terms.
*Correspondence: Paola R. Campodónico, cGNhbXBvZG9uaWNvQHVkZC5jbA==
Disclaimer: All claims expressed in this article are solely those of the authors and do not necessarily represent those of their affiliated organizations, or those of the publisher, the editors and the reviewers. Any product that may be evaluated in this article or claim that may be made by its manufacturer is not guaranteed or endorsed by the publisher.
Research integrity at Frontiers
Learn more about the work of our research integrity team to safeguard the quality of each article we publish.