- 1Institute of Nanotechnology, Karlsruhe Institute of Technology, Eggenstein-Leopoldshafen, Germany
- 2Institute of Geotechnics, Slovak Academy of Sciences, Košice, Slovakia
- 3Faculty of Physics and Center for Nanointegration Duisburg-Essen, University of Duisburg-Essen, Duisburg, Germany
- 4Faculty of Science and Technology, Keio University, Yokohama, Japan
- 5Department of Physics, State University of Maringá, Maringá, Brazil
- 6Institute of Condensed Matter Physics, Braunschweig University of Technology, Braunschweig, Germany
- 7Institute of Physics, Faculty of Science, P. J. Šafárik University, Košice, Slovakia
- 8Synthon s.r.o., Blansko, Czechia
- 9Institute of Chemistry, Faculty of Natural Sciences and Mathematics, Ss. Cyril and Methodius University in Skopje, Skopje, North Macedonia
- 10Graduate School of Science, Tokyo Metropolitan University, Tokyo, Japan
- 11Faculty of Mining, Ecology, Process Control and Geotechnologies, Technical University of Košice, Košice, Slovakia
- 12Faculty of Technology, College of Technology and Business in České Budějovice, České Budějovice, Czechia
- 13Faculty of Engineering, Slovak University of Agriculture, Nitra, Slovakia
Strontium ferromolybdate, Sr2FeMoO6, is an important member of the family of double perovskites with the possible technological applications in the field of spintronics and solid oxide fuel cells. Its preparation via a multi-step ceramic route or various wet chemistry-based routes is notoriously difficult. The present work demonstrates that Sr2FeMoO6 can be mechanosynthesized at ambient temperature in air directly from its precursors (SrO, α-Fe, MoO3) in the form of nanostructured powders, without the need for solvents and/or calcination under controlled oxygen fugacity. The mechanically induced evolution of the Sr2FeMoO6 phase and the far-from-equilibrium structural state of the reaction product are systematically monitored with XRD and a variety of spectroscopic techniques including Raman spectroscopy, 57Fe Mössbauer spectroscopy, and X-ray photoelectron spectroscopy. The unique extensive oxidation of iron species (Fe0 → Fe3+) with simultaneous reduction of Mo cations (Mo6+ → Mo5+), occuring during the mechanosynthesis of Sr2FeMoO6, is attributed to the mechanically triggered formation of tiny metallic iron nanoparticles in superparamagnetic state with a large reaction surface and a high oxidation affinity, whose steady presence in the reaction mixture of the milled educts initiates/promotes the swift redox reaction. High-resolution transmission electron microscopy observations reveal that the mechanosynthesized Sr2FeMoO6, even after its moderate thermal treatment at 923 K for 30 min in air, exhibits the nanostructured nature with the average particle size of 21(4) nm. At the short-range scale, the nanostructure of the as-prepared Sr2FeMoO6 is characterized by both, the strongly distorted geometry of the constituent FeO6 octahedra and the extraordinarily high degree of anti-site disorder. The degree of anti-site disorder ASD = 0.5, derived independently from the present experimental XRD, Mössbauer, and SQUID magnetization data, corresponds to the completely random distribution of Fe3+ and Mo5+ cations over the sites of octahedral coordination provided by the double perovskite structure. Moreover, the fully anti-site disordered Sr2FeMoO6 nanoparticles exhibit superparamagnetism with the blocking temperature TB = 240 K and the deteriorated effective magnetic moment μ = 0.055 μB per formula unit.
Introduction
The straightforward synthesis of nanoscale and (simultaneously) far-from-equilibrium solids is a great challenge despite promising developments of synthesis techniques (Bensch and Breu, 2017). Nanostructured compounds with a nonequilibrium short-range structure can hardly be accessed by conventional (thermal) solid-state preparation routes requiring high synthesis temperatures. Also wet (solution) chemistry-based preparation ways lead to the thermodynamically stable rather than metastable products. Fortunately, such hurdles can be overcome by chemical reactions initiated or accelerated by the direct absorption of mechanical energy (Baláž et al., 2013; Šepelák et al., 2013; Wilkening et al., 2017; Boldyrev, 2018). In addition, the mechanically induced chemical reactions often outperform their conventional and solution-based counterparts in terms of sustainability (Bolm and Hernández, 2018; Michalchuk et al., 2021). As a consequence, the mechanically induced chemistry (mechanochemistry) becomes a “game-changer” (Gomollón-Bel, 2019), especially in chemical synthesis (Avvakumov et al., 2001; Friščić et al., 2020; Lapshin et al., 2021). Mechanochemistry is currently undergoing rapid expansion in a number of areas, and has been applied for the synthesis of various compounds, including energy storage materials (Gombotz and Wilkening, 2021; Schlem et al., 2021), pharmaceutics (Solares-Briones et al., 2021), metal-organic frameworks (Zhou et al., 2019; Głowniak et al., 2021), organic compounds (Andersen and Mack, 2018; Michalchuk et al., 2019), catalytic materials (Pickhardt et al., 2020; Porcheddu et al., 2020; Amrute et al., 2021), nanocomposites (Sherif El-Eskandarany et al., 2021), and nanocrystalline inorganic materials (Šepelák et al., 2012a; Lunghammer et al., 2019; De Oliveira et al., 2020). From the point of view of the type of mechanical impact, the strategies to mechanically promote a chemical reaction in matter include the use of mechanical milling, extrusion techniques, pulsed ultrasonication, and single-molecule force spectroscopy approaches, among others (Lapshin et al., 2021; O’Neill and Boulatov, 2021).
The mechanochemical synthesis (sometimes shortly called mechanosynthesis), studied in the present work is a reduction-oxidation (redox) reaction via high-energy ball milling of a stoichiometric mixture of SrO, α-Fe and MoO3 precursors at ambient temperature in air, leading to the formation of strontium ferromolybdate Sr2FeMoO6. Using the recently proposed pictographic nomenclature for mechanochemical syntheses (Michalchuk et al., 2021), the present redox reaction emphasizing the changes in valence state of the Fe and Mo cations can roughly be represented as 2 SrO + α-Fe0 + Mo6+O3 + ½ O2 →
→ Sr2Fe3+Mo5+O6, where the symbols
and
specify the reaction conditions; namely, they denote the use of “planetary ball mill” and “ambient atmosphere,” respectively. Although in the last few years a surge of investigations in the field of mechanochemistry has resulted in the mechanosynthesis of a variety of complex oxides by forcing a system to acquire metastable and non-equilibrium configurations (Zhang and Saito, 2012; Šepelák et al., 2013; Wilkening et al., 2017; Porodko et al., 2021; Szczȩśniak et al., 2021; Tsuzuki, 2021; Šepelák et al., 2021), there is no report in the literature on the nonconventional mechanochemical route to synthesize Sr2FeMoO6. Commonly, this material is prepared via a conventional multi-step ceramic route (Valenzuela et al., 2014; Kalanda et al., 2019; Alvarado-Flores et al., 2021) or various wet chemistry-based routes (Li et al., 2019; Farzin et al., 2020; Valdés et al., 2021); some of them involve a prolonged heat treatment at considerably high temperatures under reducing atmosphere (Iranmanesh et al., 2016). The present work demonstrates that Sr2FeMoO6 can be mechanosynthesized at ambient temperature in air directly from its precursors in the form of nanostructured powders, without the need for solvents and/or calcination under controlled oxygen fugacity, thus making the process very facile and efficient.
From the point of view of mechanochemistry, an important question providing a part of motivation for the present work is, whether it is possible to transform, by means of a mechanochemical processing, zero-valent metallic iron (one of the reaction educts) into its three-valent (ferric) state present in the reaction product. To the best of our knowledge, a mechanochemical reaction leading to such a large extent of oxidation of iron species (Fe0 → Fe3+) has not yet been reported. Note that the Fe0 → Fe2+ transformation path has been observed to occur, for example, during the single-step mechanosynthesis of nanostructured Fe2+-containing α-Fe2SiO4 and Fe2GeO4, starting from the mixture of metallic Fe0 and corresponding precursors (Šepelák et al., 2012a; Šepelák et al., 2012b), and the mechanochemical reduction of metal sulphides to prepare nanocrystalline metals (Godočíková et al., 2004). On the other hand, the mechanochemical reduction processes leading to the Fe3+ → Fe2+ → Fe0 transformations have been reported for spinel ferrites MFe2O4 (M = Mg, Ni) (Menzel et al., 2001; Šepelák et al., 2002). The present work gives evidence of the fact that the unique mechanically triggered chemical reaction leading to the extensive oxidation of the Fe0 precursor is feasible. The evolution of the Sr2FeMoO6 phase during the mechanosynthesis as well as the far-from-equilibrium structural state of the reaction product are systematically monitored with X-ray diffraction (XRD) and a variety of comprehensive spectroscopic techniques including Raman spectroscopy, 57Fe Mössbauer spectroscopy, and X-ray photoelectron spectroscopy (XPS).
A considerable interest in Sr2FeMoO6, from both fundamental and practical points of view, is prompted by the discovery of its room-temperature low-field magnetoresistance and half-metallicity (Kobayashi et al., 1998). Such properties predestinate this material for a variety of possible technological applications in the field of spintronics and solid oxide fuel cells (Kalanda et al., 2018; Huan et al., 2017; Skutina et al., 2021; Xi et al., 2021). Sr2FeMoO6 is an important member of the family of double perovskites with the general formula A2BB′O6 (A is a divalent alkaline earth cation; B and B′ are transition metal cations). It adopts the tetragonal crystal structure with space group I4/m (Nakamura and Oikawa, 2003), in which the twelve-fold coordinated Sr2+ cations reside in the cuboctahedral cavities created by the corner-sharing octahedra, while the octahedral sites are occupied by Fe and Mo ions. Despite its deceptively simple structure, Sr2FeMoO6 exhibits disordering phenomena involving the alternating positions of Fe and Mo atoms. In the fully ordered Sr2FeMoO6 double perovskite, Fe and Mo atoms alternate regularly along the c-axis of the tetragonal crystal lattice. Intervening O2− ion bridges every Fe and Mo atom pair, thus forming the sequence of regularly alternating FeO6 and MoO6 octahedra along the c-axis (Figure 1). The cation disorder in Sr2FeMoO6, in which some Fe and Mo cations interchange their crystallographic positions, is referred to as anti-site disorder or mis-site disorder. The anti-site disordered regions (sometimes called “clusters” or “patches” (Menéndez et al., 2004)) can be easily visualized as islands consisting of oxygen octahedra whose centers are all occupied by Fe atoms or all by Mo atoms. The crystal chemical formula emphasizing the cation site occupancy in Sr2FeMoO6 may be written as Sr2[FeFe]1-ASD[FeMo]ASD[MoMo]1-ASD[MoFe]ASDO6, where [FeFe], [MoMo] and [FeMo], [MoFe] denote the cations on the “right” octahedral sites (Fe cation on Fe-site and Mo cation on Mo-site) and anti-sites (Fe cation on Mo-site and Mo cation on Fe-site), respectively. The symbol ASD represents the so-called degree of anti-site disorder defined as the fraction of Fe-sites occupied by Mo ions and vice versa. Theoretically, ASD takes a value from 0 to 0.5, i.e., 0 ≤ ASD ≤ 0.5. The value of ASD in Sr2FeMoO6 crucially depends on the conditions of its synthesis. For example, the lowest value (ASD = 0.025) has been found to be at an optimum synthesis temperature of about 1423 K for ceramics long-term annealed (up to 150 h) under oxygen-deficient conditions (Shimada et al., 2003; Huang et al., 2004). On the other hand, the considerably high values of ASD ranging from 0.345 to 0.45 have been reported for Sr2FeMoO6 prepared by melt-quenching method (Sarma et al., 2000a; Kobayashi et al., 2007). Here, we were able to mechanosynthesize the nanostructured and fully anti-site disordered Sr2FeMoO6 with a record-high value of ASD = 0.5. It should be emphasized that this value, derived independently from the present experimental XRD, Mössbauer, and magnetization data, is, to the best of our knowledge, the highest value so far reported for the Sr2FeMoO6 phase.
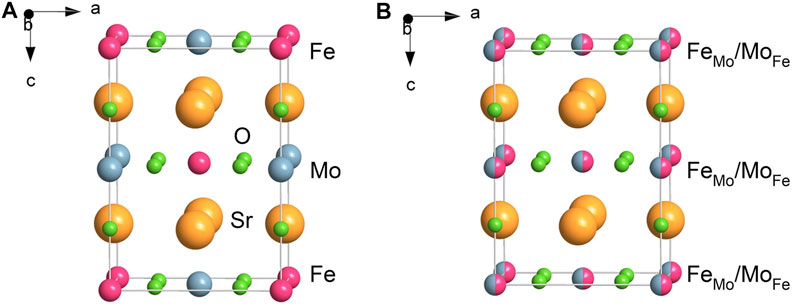
FIGURE 1. Schematic presentation of the tetragonal structure of (A) the fully ordered and (B) the fully anti-site disordered double perovskite Sr2FeMoO6. In the fully ordered Sr2FeMoO6, the Fe and Mo cations alternate regularly along the c-axis of the tetragonal crystal lattice. In the fully anti-site disordered Sr2FeMoO6, the cations are randomly distributed over the sites of octahedral coordination provided by the double perovskite structure. The crystal chemical formula of the fully disordered Sr2FeMoO6, emphasizing the cation site occupancy at the atomic level, may be written as Sr2[FeFe]0.5[FeMo]0.5[MoMo]0.5[MoFe]0.5O6, where [FeFe], [MoMo] and [FeMo], [MoFe] denote the cations on the “right” octahedral sites (Fe cation on Fe-site and Mo cation on Mo-site) and anti-sites (Fe cation on Mo-site and Mo cation on Fe-site), respectively.
Anti-site defects are recognized to drastically influence the magnetism of Sr2FeMoO6 (Reyes et al., 2016; Kalanda et al., 2020). The present study demonstrates that an extraordinarily high ASD in the as-prepared double perovskite leads to the deterioration of its magnetic properties. The mechanosynthesized Sr2FeMoO6 exhibits superparamagnetism at room temperature arising from its nanoscale nature evidenced by high-resolution transmission electron microscopy (HRTEM).
Materials and Methods
For the mechanosynthesis of Sr2FeMoO6, the reactants SrO (Alfa Aesar), α-Fe (Alfa Aesar) and MoO3 (Sigma-Aldrich) with the initial average particle sizes of 0.5, 0.7 and 0.3 μm, respectively, were mixed in a molar ratio of 2:1:1. The 2 SrO + α-Fe + MoO3 mixtures were milled for various times tM (up to 240 min) in a planetary ball mill Fritsch Pulverisette 7 Premium Line (Fritsch, Germany) at room temperature. The milling chamber (80 cm3 in volume) and 15 balls (10 mm in diameter), both made of tungsten carbide, were used. Milling experiments were performed in air at 500 rpm.
The XRD patterns were measured using a D8 Advance diffractometer (Bruker, Germany), operating in Bragg-Brentano configuration and using Cu Kα radiation. The XRD scans were collected from 10° to 70° (2 Theta), using a step of 0.03° and a data collection time of 12 s. The Powder Diffraction File (PDF) database (ICDD, Newtown Square, PA, United States) was utilized for phase identification. The tetragonal double perovskite structure of Sr2FeMoO6 was visualized using the Diamond program (Putz and Brandenburg, 2019).
Raman spectra were recorded using a micro-Raman multichannel spectrometer LabRam300 Infinity (Horiba Jobin Yvon, Japan) with the excitation wavelength of 532 nm (Nd:YAG laser). The Raman shift was calibrated using the Raman peak of silica wafer positioned at 520.7 cm−1. The acquisition time and the number of scans were set to 10 and 7 s, respectively.
57Fe Mössbauer spectra were taken in transmission geometry at room temperature (293 K) and 13 K. 57Co in Rh matrix was used as the γ-ray source. Mössbauer data were fitted using the WinNormos software package (Wissel, Germany). The derived isomer shifts (IS) are given relative to IS of α-Fe at room temperature. The degree of anti-site disorder was calculated according to formula ASD = I[FeMo]/2(I[FeFe] + I[FeMo]), where I[FeMo] and I[FeFe] are the relative intensities of Mössbauer subspectra corresponding to Fe ions on the Mo-sites and Fe-sites, respectively.
XPS measurements were performed using an XPS instrument SPECS (Specs, Germany) equipped with PHOIBOS 100 SCD and non-monochromatic X-ray source. The spectra were acquired at a basic pressure of 2 × 10−8 mbar with Mg Kα excitation at 10 kV. The spectrometer was calibrated against silver (Ag 3d). The data were analyzed using the SpecsLab2 CasaXPS software (Casa Software, United Kingdom).
HRTEM observations were performed employing a JEOL 2100F UHR microscope operated at 200 kV. High-resolution images were taken in a bright-field imaging mode. Prior to HRTEM investigations, powders were dispersed in ethanol and ultrasonicated for 10 min. Subsequently, a droplet of the water-diluted colloidal suspension was deposited on the copper-supported carbon grid. Finally, samples were stored in a vacuum chamber to evaporate residual ethanol.
Magnetic measurements were performed using a superconducting quantum interference device (SQUID) magnetometer (Quantum Design MPMS-5S). The powdered samples were filled in a small gelatin capsule, whose diamagnetic moment was subtracted from the measured magnetization values. Magnetic hysteresis loops were recorded at 10 and 300 K in external magnetic fields from 0 to ±5 T. The temperature-dependent magnetic susceptibility was measured from 5 to 300 K in a magnetic field of 0.01 T.
Results and Discussion
The evolution of the 2 SrO + α-Fe + MoO3 mixture milled in air was followed by XRD. Figure 2A shows XRD patterns of the mixture milled for various times. The XRD pattern of the starting powder (not shown) is characterized by sharp diffraction peaks corresponding to the solid reactants SrO (ICDD PDF 75-0263), α-Fe (06-0696) and MoO3 (05-0508). During the early stages of milling (for tM ≤ 15 min), XRD reveals a decrease in the intensity and an associated broadening of the Bragg peaks of the SrO and α-Fe educts, the complete disappearance of diffraction peaks assigned to the binary MoO3 precursor, and the simultaneous formation of the intermediate ternary phase SrMoO4 (08-0482); see Figure 2A (top). Note that a tiny fraction of Sr3MoO6 (27-1441) has also been detected in the sample milled up to 30 min. In analogy with our previous work on the mechanosynthesis of oxymolybdates (Tóthová et al., 2019) it can be assumed that a rapid formation of the ternary molybdates is accelerated by the emergence of the structurally disordered (or even amorphous) and, consequently, very reactive MoO3 phase induced by mechanical action already in the early stages of milling process. For milling times tM ≥ 30 min, qualitative changes are observed in the XRD patterns of the milled samples; clear evidence is observed of new diffraction features that correspond to the complete disappearance of the intermediates and the simultaneous formation of the Sr2FeMoO6 quaternary phase. In the XRD pattern of the mixture milled for 240 min (a product of the mechanochemical reaction), all diffraction peaks detected above the background are due to the tetragonal Sr2FeMoO6 phase (70-8133); see Figure 2A (bottom). Rietveld analysis of XRD data revealed the lattice parameters (a = 5.5856(5) Å, c = 7.8970(140) Å) and the random distribution of Fe and Mo cations (ASD = 0.5) in the mechanosynthesized Sr2FeMoO6. The latter will be discussed in detail concurrently with the analysis of the low-temperature 57Fe Mössbauer spectroscopic data and SQUID magnetization data (see below).
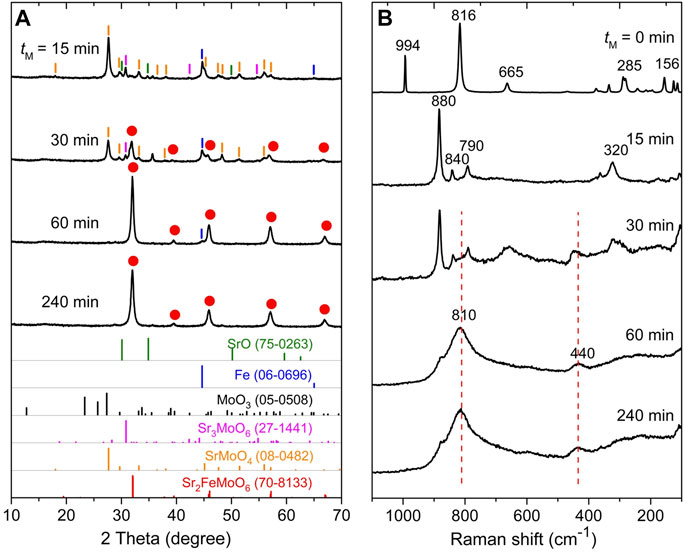
FIGURE 2. (A) XRD patterns and (B) Raman spectra of the 2 SrO + α-Fe + MoO3 mixture milled for various times (tM) up to 240 min in air. The mechanochemical reaction 2 SrO + α-Fe + MoO3 + ½ O2 →
→ Sr2FeMoO6 proceeds through the formation of intermediate phases SrMoO4 and Sr3MoO6. The theoretical Bragg peak positions with the ICDD PDF numbers corresponding to the reaction precursors, intermediate phases and the product phase are presented at the bottom of panel 2A. The positions of Raman peaks associated with the formed Sr2FeMoO6 phase are indicated in Panel 2B by the dashed lines.
To provide insight into the formation of intermediates in greater detail, the mechanochemical reaction 2 SrO + α-Fe + MoO3 + ½ O2 →
→ Sr2FeMoO6 was also followed by Raman spectroscopy. Figure 2B compares Raman spectra of the 2 SrO + α-Fe + MoO3 mixtures milled for various tM. The spectrum of the starting mixture (tM = 0 min) shows all Raman peaks typical for MoO3 reactant. Note that the first-order Raman bands are neither expected for bcc structure of α-Fe nor for SrO being two remaining solid reactants (Mon, 1972). The strongest Raman peaks at 816 cm−1 (with symmetries Ag, B1g) and 994 cm−1 (Ag, B1g) evolve from the asymmetric and symmetric stretching of the terminal oxygen atoms in MoO3, whereas the band at 665 cm−1 (B2g, B3g) appears from the asymmetric stretching of the Mo–O–Mo bridge along the c-axis (Mestl et al., 1994). The lower wavenumber bands at 281 and 289 cm−1 (B2g, B3g) are ascribed to the wagging modes of the terminal oxygen atoms, in addition to the 156 cm−1 band (Ag, B1g) that originates from the translation of rigid chains. An interesting observation is that already after 15 min of high-energy milling, all the Raman peaks assigned to the MoO3 precursor disappear, and new bands at 880, 840, 790, and 320 cm−1 evolve (Figure 2B). These bands are attributed to the formed SrMoO4 intermediate phase (Sujatha et al., 2019). This finding supports and complements the above given XRD results, which reveal the complete and rapid reaction of MoO3 and SrO precursors yielding the intermediate ternary molybdate SrMoO4 already in the early stages of milling process. Further milling (tM > 15 min) provokes significant changes in the Raman spectra; the most substantial feature is the appearance of a broad and intense complex band peaking at about 810 cm−1 (ranging from about 850 to 770 cm−1) that, in addition to the 440 cm−1 weaker band, is characteristic for Sr2FeMoO6 (Son et al., 2001; Navarro et al., 2003). The broad shape of these Raman peaks reflects the structurally disordered nature of the final reaction product. Taking into account the results of the present XRD and Raman spectroscopic investigations, the overall reaction path leading to Sr2FeMoO6 and involving the formation of the SrMoO4 intermediate can be expressed by the following sequence of two mechanochemical reactions: 2 SrO + α-Fe0 + Mo6+O3 + ½ O2 →
→ SrO + α-Fe0 + SrMo6+O4 + ½ O2 →
→ Sr2Fe3+Mo5+O6. The first reaction represents the mechanochemical synthesis of SrMo6+O4, which is not accompanied by the valence change of the constituent cations, whereas the subsequent mechanochemical redox reaction includes the reduction of Mo6+ cations and the oxidation of Fe0 atoms. Note that a small amount of the SrMoO4 intermediate remains unreacted in the sample milled up to 240 min (see a tiny Raman peak at 880 cm−1 at the bottom of Figure 2B).
To determine the mechanically induced phase evolution of the 2 SrO + α-Fe + MoO3 mixture from the point of view of the α-Fe precursor as an oxygen getter and to provide complementary insight into the short-range (local) structure of the formed Sr2FeMoO6 phase, the mechanochemical reaction was also followed by 57Fe Mössbauer spectroscopy. This nuclear spectroscopic method has been proven to be well suited for the investigation of the charge state, the local coordination, and the magnetic state of iron ions in mechanosynthesized materials (Šepelák and Becker, 2000; Šepelák et al., 2006; Šepelák et al., 2007; Da Silva et al., 2011; Harris and Šepelák, 2018; Tóthová et al., 2018; Skurikhina et al., 2020). The room-temperature 57Fe Mössbauer spectra illustrating the mechanically induced evolution of the 2 SrO + α-Fe + MoO3 mixture are presented in Figure 3. As can be seen, the spectrum of the starting mixture shows a sextet with isomer shift IS = 0.02(1) mm/s and magnetic hyperfine field Bhf = 33.1(1) T corresponding to bcc metallic Fe in ferromagnetic state. With increasing tM, the sextet becomes asymmetric toward the inside of each line, slowly collapses, and is gradually replaced by a central asymmetric doublet. In the spectrum of the sample milled for 240 min (see Figure 3, bottom), the sextet corresponding to α-Fe is not visible anymore and the central doublet becomes the only spectral component. Considering the relatively high ferrimagnetic-to-paramagnetic transition temperature of bulk (microcrystalline) Sr2FeMoO6 (Tc = 415 K (Kobayashi et al., 1998)), the presence of the doublet in the room-temperature 57Fe Mössbauer spectrum of the sample milled for 240 min seems to be an unexpected finding. It can be understood to arise mostly from Fe cations in mechanosynthesized Sr2FeMoO6 nanoparticles exhibiting superparamagnetism (see below). Note that the latter arises if particle sizes are so small that thermally induced energy fluctuations (∼kBT, where kB is the Boltzmann constant and T is temperature) overcome the magnetic anisotropy energy (∼KV, where K is the anisotropy energy constant and V is the particle volume) and change the direction of the magnetization of a particle from one easy axis to another (Long, 1987). Similar magnetic relaxation phenomena and the complete absence of the magnetic hyperfine splitting at room temperature have been observed in other Fe-containing ultrafine-particle magnetics (e.g., ferrites) prepared via mechanosynthesis (Šepelák et al., 2006; Šepelák et al., 2007; Da Silva et al., 2011).
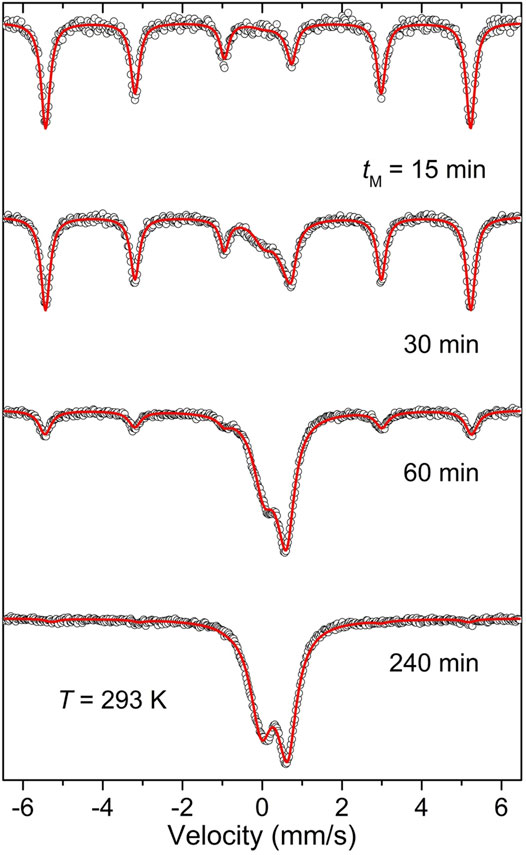
FIGURE 3. Room-temperature 57Fe Mössbauer spectra of the 2 SrO + α-Fe + MoO3 mixture milled for various times (tM) up to 240 min.
Since the relaxation time characterizing the superparamagnetic phenomenon is also a function of temperature (τ ∼ exp(KV/kBT)) (Néel, 1949), the influence of superparamagnetic relaxation can be counteracted by reducing the sample temperature. Figure 4A presents the low-temperature (13 K) 57Fe Mössbauer spectrum of the 2 SrO + α-Fe + MoO3 mixture milled for 240 min. It is clearly visible that thermal fluctuations are suppressed at 13 K, and the Mössbauer spectrum of the sample consists of the superposition of three magnetic hyperfine splitting components (sextets). The hyperfine parameters resulting from the least-squares fitting of the spectrum are presented in Table 1. The first minor sextet (orange subspectrum with the relative intensity I[FeFe] ∼ 5.9%) is assigned to the Fe cations in the ordered regions (clusters) of the mechanosynthesized Sr2FeMoO6 double perovskite with the regularly alternating corner-sharing FeO6 and MoO6 octahedra along the c-axis of its tetragonal crystal lattice. The value IS = 0.92(6) mm/s for this sextet corresponds to the state with a mixed valence of iron cations Fe2+/Fe3+ (between Fe2+ and Fe3+; sometimes formally denoted as Fe2.5+) (Greneche et al., 2001; Menéndez et al., 2004). This degenerate nature of the fundamental state of iron cations in Sr2FeMoO6 is the result of the existing equilibrium Fe3+ + Mo5+ ↔ Fe2+ + Mo6+, in which the itinerant down-spin electron is shared by both types of atoms (Sarma et al., 2000b; Lindén et al., 2000).
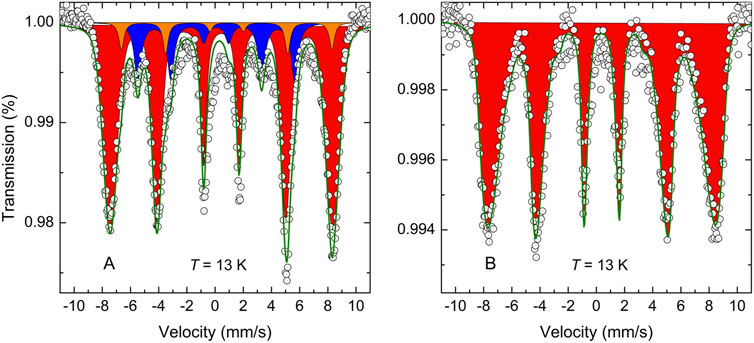
FIGURE 4. The low-temperature (13 K) 57Fe Mössbauer spectra of the 2 SrO + α-Fe + MoO3 mixture milled for 240 min (A) before and (B) after thermal treatment at 923 K for 30 min in air. The spectra correspond to (A) the partly synthesized Sr2FeMoO6 and (B) the completely synthesized Sr2FeMoO6. The orange and red subspectra are associated with Fe cations in the ordered and anti-site disordered regions of Sr2FeMoO6 double perovskite, respectively. The blue subspecrum corresponds to α-Fe.
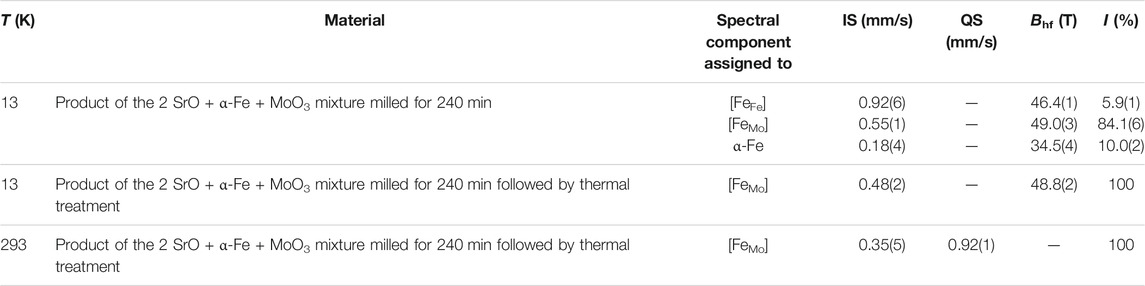
TABLE 1. Parameters obtained by fitting 57Fe Mössbauer spectra taken at 13 and 293 K for the 2 SrO + α-Fe + MoO3 mixture milled for 240 min before and after its thermal treatment. IS is the isomer shift; QS is the quadrupole splitting; Bhf is the magnetic hyperfine field; I is the relative intensity of the spectral component. Symbols [FeFe] and [FeMo] denote iron cations in octahedral coordination of oxygen anions in the ordered and anti-site disordered regions of Sr2FeMoO6, respectively.
The major component in the spectrum shown in Figure 4A (red subspectrum with the relative intensity I[FeMo] ∼ 84.1%) is ascribed to the high-spin Fe3+ cations in octahedral coordination of oxygen anions in the anti-site disordered regions of the mechanosynthesized Sr2FeMoO6, where Mo-sites are occupied by Fe3+ cations and vice versa. From the relative intensities I[FeMo] and I[FeFe], the degree of anti-site disorder in the mechanosynthesized Sr2FeMoO6 is estimated to be ASD ∼ 0.467. Thus, the mechanosynthesized Sr2FeMoO6 can be considered as a nonequilibrium, highly disordered double perovskite possessing the almost random distribution of the Fe and Mo cations over the sites of octahedral coordination provided by the double perovskite structure. It should be noted that the iron nuclei located on the anti-sites ([FeMo]) experience the most probable local magnetic hyperfine field Bhf = 49.0(3) T with a relatively broad field distribution of about 10 T (from about 45 to 55 T). This fact is a strong indication of both, the presence of a wide variation in the local chemical environment around Fe3+ cations from site to site and the presence of a strongly distorted geometry of FeO6 octahedra in the anti-site disordered clusters of the mechanosynthesized material. This is in contrast to the iron cations located on the “right” octahedral sites ([FeFe]), where the magnetic hyperfine field with the lower magnitude (Bhf = 46.4(1) T) and relatively narrow distribution of about 5 T is observed.
The Mössbauer active 57Fe nuclei provide a very sensitive probe for the estimation of the yield of a mechanochemical formation reaction of Fe-containing compounds (Šepelák et al., 2006; Da Silva et al., 2011). The presence of the third sextet, that is assigned to metallic α-Fe nanoparticles (blue subspectrum with the relative intensity Iα-Fe ∼ 10% in Figure 4A), indicates that the present mechanochemical reaction is not completed after 240 min; its degree of conversion is about 90%. Note that this sextet is not present in the corresponding room-temperature Mössbauer spectrum (see Figure 3, bottom), confirming the superparamagnetic state of α-Fe nanoparticles in the mixture of the milled reactants. Taking into account that the present heterogeneous mechanochemical reaction involving solid precursors and the gaseous phase (oxygen) proceeds inside the closed milling chamber, it can be assumed that the further progress of the mechanosynthesis of Sr2FeMoO6 was hampered by the shortage of oxygen inside the chamber, and the reaction stopped after the full consumption of oxygen. However, the subsequent thermal treatment of the sample at a fairly moderate temperature of 923 K for 30 min in air leads to the further reaction of remaining α-Fe nanoparticles in the reaction mixture, and results in the full (100%) conversion of the reactants into the double perovskite Sr2FeMoO6 phase. This is documented in Figure 4B, where the low-temperature 57Fe Mössbauer spectrum of the 2 SrO + α-Fe + MoO3 mixture milled for 240 min taken after its thermal treatment is shown. The hyperfine parameters of the as-prepared Sr2FeMoO6 are listed in Table 1. It should be emphasized that the 57Fe Mössbauer spectrum of the completely synthesized Sr2FeMoO6 consists of only one spectral component corresponding to the fully anti-site disordered Sr2FeMoO6 double perovskite with ASD = 0.5. This extraordinarily high value is, to the best of our knowledge, the highest value so far reported for the anti-site disordered Sr2FeMoO6 phase.
An interesting observation is that the moderate thermal tretament leads to the transformation of the small amount (∼5.9 wt.%) of the ordered Sr2FeMoO6 clusters into the anti-site disordered state. This unusual structural order-disorder transformation upon heating can be caused by the progression of concurrent reaction of remaining Fe0 (∼10 wt.%) in the reaction mixture. It seems that tiny metallic iron in superparamagnetic state with a large reaction surface and a high oxidation affinity plays an important role in the present mechanosynthesis of Sr2FeMoO6. Its mechanically induced steady presence in the reaction mixture of educts initiates/promotes the redox Fe0 → Fe3+ and Mo6+ → Mo5+ reactions.
To verify the valence state of the Fe and Mo ions in the as-prepared fully anti-site disordered double perovskite, information on its short-range structure, provided by the 57Fe Mössbauer spectroscopic technique, is complemented by an investigation of the electronic state of the constituent cations by means of X-ray photoelectron spectroscopy. Figure 5 displays both the room-temperature 57Fe Mössbauer and XPS spectra of the material. The 57Fe Mössbauer spectrum (Figure 5A) consists of the symmetric doublet that is well fitted by only one spectral component with isomer shift IS = 0.35(5) mm/s and quadrupole splitting QS = 0.92(1) mm/s. The latter values of hyperfine parameters are characteristic of Fe3+ cations in octahedral coordination of oxygen anions (Menil, 1985). The presence of the doublet also reveals that, even after its moderate thermal treatment, the as-prepared fully anti-site disordered Sr2FeMoO6 double perovskite exhibits superparamagnetism at room temperature (see doublets in Figure 2 (bottom) and Figure 5A). This demonstrates a fairly good stability of the superparamagnetic material at moderate temperatures, and simultaneously indicates that the thermal treatment applied does not bring about a substantial growth of Sr2FeMoO6 nanoparticles (see also the detailed HRTEM analysis below). It is also worth to mention that the value of quadrupole splitting (QS ∼ 0.9 mm/s) for the as-prepared double perovskite is significantly larger than that observed for other superparamagnetic fine Sr2FeMoO6 nanoparticles (Yarmolich et al., 2016; Suominen et al., 2007). This variation is associated with the presence of strongly deformed FeO6 octahedra in the mechanosynthesized perovskite, as it is derived from the low-temperature 57Fe Mössbauer spectra presented in Figure 4. Note that the broadly distorted geometry of the constituent structural units (building blocks) is typical for nanosized particles prepared by mechanochemical routes, and it has been evidenced by nuclear spectroscopic methods in numerous far-from-equilibrium complex oxides (Šepelák et al., 2009; Šepelák et al., 2013; Da Silva et al., 2021). This is also in line with previous work on Sr2FeMoO6, where an important crystallographic distortion around Fe3+ cations is postulated due to highly unspherical electric fields generated by anti-side disorder (Greneche et al., 2001) or, alternatively, due to the existence of Mo vacancies (Chmaissem et al., 2000).
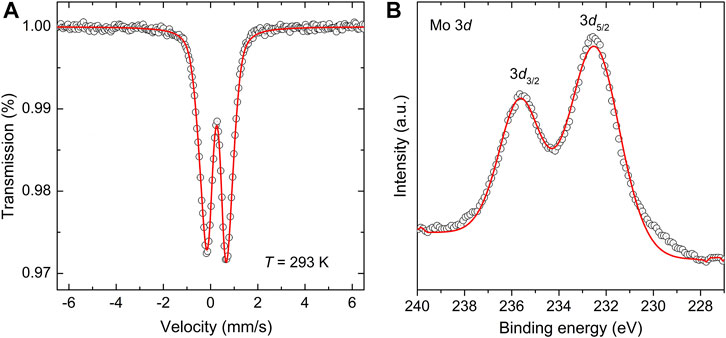
FIGURE 5. (A) The room-temperature 57Fe Mössbauer spectrum and (B) the Mo 3d core level XPS spectrum of the as-prepared Sr2FeMoO6.
The Mo 3d core level XPS spectrum of the as-prepared Sr2FeMoO6 is displayed in Figure 5B. In the XPS spectrum of the initial 2 SrO + Fe + MoO3 mixture (the spectrum not shown), the Mo 3d core electron level appears at the binding energies of 232.1 and 235.2 eV for 3d5/2 and 3d3/2 electronic states, respectively. These values are typical for the Mo6+ valence state in the MoO3 educt (Maiti et al., 2019). With increasing milling time, i.e., with increasing amount of the Sr2FeMoO6 phase in the reaction mixture, the 3d5/2 and 3d3/2 electron sublevels shift to sligtly higher binding energies. For the mechanosynthesized Sr2FeMoO6, the structureless 3d5/2 and 3d3/2 peaks are observed at 232.6 and 235.7 eV, respectively, (see Figure 5B). Using the literature values for different Mo oxides (Sarma et al., 2000b; Jalili et al., 2009), the observed Mo 3d5/2 and Mo 3d3/2 features are ascribed to arise solely from the Mo5+ oxidation state. Note that the similar values of binding energies (232.2 and 235.3 eV) have been reported for the Mo5+ state in nanoscale thin films of Sr2FeMoO6 (Jalili et al., 2009). Thus, it is clear from the analyses given hitherto that the present mechanochemical redox reaction can be written as: 2 SrO + α-Fe0 + Mo6+O3 + ½ O2 →
→ Sr2Fe3+Mo5+O6, where Mo6+ is reduced to Mo5+ and Fe0 is oxidized to Fe3+. To the best of our knowledge, the one-step mechanochemical synthesis accompanied by such a large extent of oxidation (Fe0 → Fe3+) has not yet been reported.
Taking into account that superparamagnetism, evidenced for the as-prepared materials by means of 57Fe Mössbauer spectroscopy (see above), is the particle size (the particle volume) dependent phenomenon (Néel, 1949), HRTEM investigations of both the partly synthesized Sr2FeMoO6 (the 2 SrO + Fe + MoO3 mixture milled for 240 min) and the completely synthesized Sr2FeMoO6 (the mixture milled for 240 min followed by its thermal treatment at 923 K for 30 min) were performed to uncover their morphology. The representative HRTEM micrographs of the as-prepared materials are shown in Figure 6. It is revealed that both materials consist of agglomerated fully crystalline nanoparticles. The partly synthesized Sr2FeMoO6 is found to exhibit a particle size distribution ranging from about 5 to 12 nm (an average particle size is estimated to be 9(2) nm) with the irregular particle shape and random orientation, whereas the completely synthesized Sr2FeMoO6 possesses a broader particle size distribution (7–28 nm) with the average particle size of about 21(4) nm and the roughly spherical particle shape. The relatively broad distribution of particle sizes can be a result of the heterogeneous nucleation-and-growth processes (Da Silva et al., 2011) of the product phase in the course of the mechanically induced formation reaction. In both cases, the high-resolution TEM images show lattice fringes corresponding to the crystallographic planes with Miller indices (012) (interplanar distance d(012) = 0.36 nm) (Figure 6A) and (200) (d(200) = 0.28 nm) (Figure 6B) of the Sr2FeMoO6 phase (ICDD PDF 70-8133). The lattice fringes cross the whole interior of particles demonstrating their single-crystalline character. Thus, it is evident now that the applied moderate thermal treatment of the partly synthesized Sr2FeMoO6 nanoparticles does not bring about their substantial growth; in the thermally treated sample, Sr2FeMoO6 nanoparticles are still sufficiently small to exhibit superparamagnetic state at room-temperature (see Figure 5A).
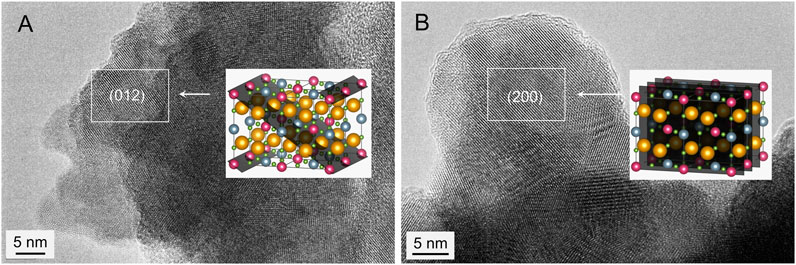
FIGURE 6. HRTEM images of (A) the partly synthesized Sr2FeMoO6 and (B) the completely synthesized Sr2FeMoO6. The lattice fringes correspond to the crystallographic planes (A) (012) with interplanar distance d(012) = 0.36 nm and (B) (200) with d(200) = 0.28 nm of the Sr2FeMoO6 phase (ICDD PDF 70-8133).
The temperature-dependent magnetization measurements were performed to confirm the superparamagnetic state of the as-prepared nanostructured Sr2FeMoO6. Figure 7A shows the zero-field-cooled (ZFC) and field-cooled (FC) magnetization curves of the nanomaterial measured in the temperature range from 5 to 300 K in an external magnetic field of 0.01 T. Above 5 K, the increase with temperature of the ZFC curve is an indicator of the appearance of superparamagnetism in Sr2FeMoO6 nanoparticles, which give rise to a single maximum at about Ts = 120 K (Hansen and Mørup, 1999). The latter is a critical point (the so-called blocking temperature) corresponding to the blocking (freezing of the magnetic moments) of the smallest nanoparticles in the double perovskite particulate system. The point at which the ZFC and FC curves start to coincide (TB ∼ 240 K) is associated with the blocking temperature of the biggest Sr2FeMoO6 nanoparticles. The relatively large difference between Ts and TB (∼120 K) indicates that the as-prepared double perovskite possesses a relatively broad distribution of particle sizes. The latter finding confirms the results of HRTEM investigations presented above. The presence of a single maximum in the ZFC curve points to a single-stage process of the nanoparticle blocking (Yu et al., 1999), which reflects a single-modal particle size distribution in the nanostructured material.
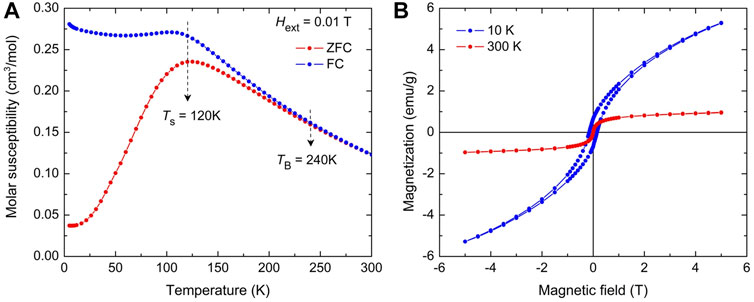
FIGURE 7. (A) Zero-field-cooled (ZFC) and field-cooled (FC) magnetization curves for the as-prepared Sr2FeMoO6 taken at external magnetic field Hext = 0.01 T. Ts and TB denote blocking temperatures of the smallest and the biggest Sr2FeMoO6 nanoparticles in the double perovskite particulate system, respectively. (B) Magnetization hysteresis loops for the as-prepared Sr2FeMoO6 measured at 10 K (blue line) and 300 K (red line).
There is a strong correlation between the octahedral cation anti-site disorder in Sr2FeMoO6 and its magnetism (Kobayashi et al., 1998; Ogale et al., 1999; Reyes et al., 2016). The magnetic ground state of the fully ordered Sr2FeMoO6 is usually considered as a ferrimagnetic collinear state with parallel magnetic moments of Fe3+ cations (μFe = 5 μB, μB is Bohr magneton; μB = 9.27408 × 10−21 emu), which are antiparallel to the moments of Mo5+ cations (μMo = 1 μB) (Saloaro et al., 2016). Taking into account the formula Sr2[FeFe↑]1-ASD[FeMo↓]ASD[MoMo↓]1-ASD[MoFe↑]ASDO6, which emphasizes the cation site occupancy and the spin alignment in the anti-site disordered Sr2FeMoO6, the effective magnetic moment μ per formula unit (f.u.) of the material may be written as:
Thus, this simplified approach taking into account the spin-only contribution of cations and omitting the itinerant character of spin-down electrons shows that μ linearly decreases with increasing ASD. The effective magnetic moment μ = 4 μB/f.u. corresponds to the fully ordered double perovskite with ASD = 0 and μ = 0 for the fully disordered double perovskite with ASD = 0.5. The linear decrease of the saturation magnetization of Sr2FeMoO6 with increasing ASD has also been predicted on the basis of Monte Carlo simulations (Ogale et al., 1999) and ab initio calculations (Reyes et al., 2016). The degradation of magnetic properties of Sr2FeMoO6 with increasing cation disorder has also been confirmed experimentally in numerous papers, see, e.g., (Suchaneck et al., 2020).
The magnetization hysteresis loops of the as-prepared nanostructured Sr2FeMoO6 measured at 300 and 10 K are depicted in Figure 7B. As expected for magnetization measurements above TB, the room-temperature hysteresis loop of the nanomaterial is dominated by the superparamagnetic relaxation effect, whereas a slight magnetic hysteresis is observed at 10 K. Thus, the room-temperature M(Hext) curve was analyzed in terms of superparamagnetic behavior using the Brillouin function. The effective magnetic moment derived from the analysis attains the value μ = 0.055 μB/f.u. Using the above given relation μ = (4 – 8 ASD) μB and the experimentally determined magnetic moment μ = 0.055 μB, the value of ASD for the as-prepared Sr2FeMoO6 is estimated to be about 0.493. This value derived from SQUID measurements is in excellent agreement with that obtained from the present Mössbauer and XRD data (see above). This extraordinarily high degree of anti-site disorder in the as-prepared nanostructured Sr2FeMoO6 corresponds to the completely random distribution of cations with a maximum configurational entropy. It should be emphasized that the nearly random distribution of cations has also been reported for spinel oxides prepared by mechanochemical routes (Šepelák et al., 2013).
Conclusion
The high-energy milling of the 2 SrO + α-Fe + MoO3 mixture at ambient temperature in air leads to the mechanochemical synthesis of the tetragonal double perovskite Sr2FeMoO6 with the lattice parameters a = 5.5856(5) Å and c = 7.8970(140) Å. The overall reaction path leading to Sr2FeMoO6 involves the formation of the intermediate SrMoO4 ternary phase, and can be expressed by the following sequence of two mechanochemical reactions: 2 SrO + α-Fe0 + Mo6+O3 + ½ O2 →
→ SrO + α-Fe0 + SrMo6+O4 + ½ O2 →
→ Sr2Fe3+Mo5+O6. The first one represents the mechanochemical synthesis of the intermediate SrMo6+O4 phase, which is not accompanied by the valence change of the constituent cations, whereas the subsequent mechanochemical redox reaction includes the reduction of Mo6+ cations and the oxidation of Fe0 atoms. This unique mechanochemical synthesis accompanied by such a large extent of oxidation (Fe0 → Fe3+) has not yet been reported. The degree of conversion of the present mechanochemical synthesis is estimated to be about 90%. The subsequent thermal treatment of the mechanically activated material at a fairly moderate temperature of 923 K for 30 min in air results in the full conversion of the reactants into the double perovskite Sr2FeMoO6 phase. Despite the latter processing, the completely synthesized Sr2FeMoO6 possesses the nanostructured nature characterized by a relatively broad particle size distribution (7–28 nm) with the average particle size of about 21(4) nm and the roughly spherical particle shape. The degree of anti-site disorder in the as-prepared Sr2FeMoO6 is found to be ASD = 0.5, demonstrating its far-from-equilibrium, highly disordered structural state possessing the completely random distribution of Fe3+ and Mo5+ cations over the sites of octahedral coordination provided by the double perovskite structure. It should be emphasized that this record-high value of ASD = 0.5, derived independently from the present experimental XRD, Mössbauer, and magnetization data, is, to the best of our knowledge, the highest value so far reported for the Sr2FeMoO6 phase. At the short-range scale, the iron nuclei located on the anti-sites ([FeMo]) experience a relatively broad distribution of magnetic hyperfine fields of about 10 T (from about 45 to 55 T) reflecting both, the presence of a wide variation in the local chemical environment around Fe3+ cations from site to site and the presence of a strongly distorted geometry of the constituent FeO6 octahedra. The nanostructured and fully anti-site disordered Sr2FeMoO6 exhibits the superparamagnetic behavior with the blocking temperature of about TB = 240 K and the effective magnetic moment μ = 0.055 μB/f.u.
Data Availability Statement
The original contributions presented in the study are included in the article/supplementary material, further inquiries can be directed to the corresponding author.
Author Contributions
VŠ: Principal Investigator of the project; Conceptualization; Supervision; Data interpretation; Resources; Writing−original draft. ET: Synthesis; Formal analysis; Writing−original draft. AD: Conceptualization; Synthesis. RW, RB, AS, and RK: Mössbauer measurements and analyses; Data interpretation; Software; Methodology. MS: Conceptualization; Methodology; Formal analysis. KD: Visualization; Software; Methodology; Formal analysis. DM: SQUID investigations; Data interpretation. VG: HRTEM investigations. MH and MB: XRD investigations. PM, SK, and JV: Raman investigations; Methodology; Data interpretation; Formal analysis. MK: XPS investigations; Data interpretation. HH: Supervision.
Funding
The present work is supported by the DFG within the projects SE 1407/4-2 and DU 1668/3-1. Partial support by the CAPES, the APVV (projects 19-0526 and 18-0357), the VEGA (2/0055/19 and 2/0112/22) and the COST Action CA18112 MechSustInd (www.mechsustind.eu) is gratefully acknowledged. ET, MS, and KD thank the Karlsruhe Institute of Technology (KIT) for supporting their research activities at KIT. We acknowledge support by the KIT-Publication Fund.
Conflict of Interest
Author MH was employed by the company Synthon s.r.o.
The remaining authors declare that the research was conducted in the absence of any commercial or financial relationships that could be construed as a potential conflict of interest.
The handling Editor declared a past co-authorship with one of the author MB.
Publisher’s Note
All claims expressed in this article are solely those of the authors and do not necessarily represent those of their affiliated organizations, or those of the publisher, the editors and the reviewers. Any product that may be evaluated in this article, or claim that may be made by its manufacturer, is not guaranteed or endorsed by the publisher.
References
Alvarado-Flores, J. J., Mondragón-Sánchez, R., Ávalos-Rodríguez, M. L., Alcaraz-Vera, J. V., Rutiaga-Quiñones, J. G., and Guevara-Martínez, S. J. (2021). Synthesis, Characterization and Kinetic Study of the Sr2FeMoO6-δ Double Perovskite: New Findings on the Calcination of One of its Precursors. Int. J. Hydrogen Energ. 46 (51), 26185–26196. doi:10.1016/j.ijhydene.2021.01.191
Amrute, A. P., De Bellis, J., Felderhoff, M., and Schüth, F. (2021). Mechanochemical Synthesis of Catalytic Materials. Chem. Eur. J. 27 (23), 6819–6847. doi:10.1002/chem.202004583
Andersen, J., and Mack, J. (2018). Mechanochemistry and Organic Synthesis: From Mystical to Practical. Green. Chem. 20 (7), 1435–1443. doi:10.1039/c7gc03797j
Avvakumov, G. V., Senna, M., and Kosova, N. V. (2001). Soft Mechanochemical Synthesis: A Basis for New Chemical Technologies. Boston: Kluwer Academic Publishers.
Baláž, P., Achimovičová, M., Baláž, M., Billik, P., Cherkezova-Zheleva, Z., Criado, J. M., et al. (2013). Hallmarks of Mechanochemistry: From Nanoparticles to Technology. Chem. Soc. Rev. 42 (18), 7571–7637. doi:10.1039/c3cs35468g
Bensch, W., and Breu, J. (2017). Editorial. Z. Krist.-Cryst. Mater. 232 (1−3), 1. doi:10.1515/zkri-2017-5001
Boldyrev, V. V. (2018). Mechanochemical Processes with the Reaction-Induced Mechanical Activation. Chemo-Mechanochemical Effect. Russ. Chem. Bull. 67 (6), 933–948. doi:10.1007/s11172-018-2162-z
Bolm, C., and Hernández, J. G. (2018). From Synthesis of Amino Acids and Peptides to Enzymatic Catalysis: A Bottom-Up Approach in Mechanochemistry. ChemSusChem 11 (9), 1410–1420. doi:10.1002/cssc.201800113
Chmaissem, O., Kruk, R., Dabrowski, B., Brown, D. E., Xiong, X., Kolesnik, S., et al. (2000). Structural Phase Transition and the Electronic and Magnetic Properties of Sr2FeMoO6. Phys. Rev. B 62 (21), 14197–14206. doi:10.1103/PhysRevB.62.14197
Da Silva, K. L., Menzel, D., Feldhoff, A., Kübel, C., Bruns, M., Paesano, A., et al. (2011). Mechanosynthesized BiFeO3 Nanoparticles with Highly Reactive Surface and Enhanced Magnetization. J. Phys. Chem. C 115 (15), 7209–7217. doi:10.1021/jp110128t
Da Silva, K. L., Trautwein, R. S., Da Silva, R. B., Fabián, M., Čižmár, E., Holub, M., et al. (2021). Suppression of the Cycloidal Spin Arrangement in BiFeO3 Caused by the Mechanically Induced Structural Distortion and its Effect on Magnetism. Front. Mater. 8, 717185. doi:10.3389/fmats.2021.717185
De Oliveira, P. F. M., Torresi, R. M., Emmerling, F., and Camargo, P. H. C. (2020). Challenges and Opportunities in the Bottom-Up Mechanochemical Synthesis of Noble Metal Nanoparticles. J. Mater. Chem. A. 8 (32), 16114–16141. doi:10.1039/d0ta05183g
El-Eskandarany, M. S., Al-Hazza, A., Al-Hajji, L. A., Ali, N., Al-Duweesh, A. A., Banyan, M., et al. (2021). Mechanical Milling: A Superior Nanotechnological Tool for Fabrication of Nanocrystalline and Nanocomposite Materials. Nanomaterials 11 (10), 2484. doi:10.3390/nano11102484
Farzin, Y. A., Babaei, A., and Ataie, A. (2020). Low-temperature Synthesis of Sr2FeMoO6 Double Perovskite; Structure, Morphology, and Magnetic Properties. Ceramics Int. 46 (10), 16867–16878. doi:10.1016/j.ceramint.2020.03.264
Friščić, T., Mottillo, C., and Titi, H. M. (2020). Mechanochemistry for Synthesis. Angew. Chem. Intl Edit 59 (3), 1018–1029. doi:10.1002/anie.201906755
Głowniak, S., Szczęśniak, B., Choma, J., and Jaroniec, M. (2021). Mechanochemistry: Toward Green Synthesis of Metal-Organic Frameworks. Mater. Today 46, 109–124. doi:10.1016/j.mattod.2021.01.008
Godočíková, E., Baláž, P., Boldižárová, E., Škorvánek, I., Kováč, J., and Choi, W. (2004). Mechanochemical Reduction of Lead Sulphide by Elemental Iron. J. Mater. Sci. 39 (16-17), 5353–5355. doi:10.1023/B:JMSC.0000039243.89535.ef
Gombotz, M., and Wilkening, H. M. R. (2021). Fast Li Ion Dynamics in the Mechanosynthesized Nanostructured Form of the Solid Electrolyte Li3YBr6. ACS Sust. Chem. Eng. 9 (2), 743–755. doi:10.1021/acssuschemeng.0c06694
Gomollón-Bel, F. (2019). Ten Chemical Innovations that Will Change Our World: IUPAC Identifies Emerging Technologies in Chemistry with Potential to Make Our Planet More Sustainable. Chem. Int. 41 (2), 12–17. doi:10.1515/ci-2019-0203
Greneche, J. M., Venkatesan, M., Suryanarayanan, R., and Coey, J. M. D. (2001). Mössbauer Spectrometry of A2FeMoO6 (A = Ca, Sr, Ba): Search for Antiphase Domains. Phys. Rev. B 63 (17), 174403. doi:10.1103/PhysRevB.63.174403
Hansen, M. F., and Mørup, S. (1999). Estimation of Blocking Temperatures from ZFC/FC Curves. J. Magn. Magn. Mater. 203 (1-3), 214–216. doi:10.1016/S0304-8853(99)00238-3
Harris, V. G., and Šepelák, V. (2018). Mechanochemically Processed Zinc Ferrite Nanoparticles: Evolution of Structure and Impact of Induced Cation Inversion. J. Magnetism Magn. Mater. 465, 603–610. doi:10.1016/j.jmmm.2018.05.100
Huan, Y., Li, Y., Yin, B., Ding, D., and Wei, T. (2017). High Conductive and Long-Term Phase Stable Anode Materials for SOFCs: A2FeMoO6 (A = Ca, Sr, Ba). J. Power Sourc. 359, 384–390. doi:10.1016/j.jpowsour.2017.05.079
Huang, Y. H., Lindén, J., Yamauchi, H., and Karppinen, M. (2004). Simple and Efficient Route to Prepare Homogeneous Samples of Sr2FeMoO6 with a High Degree of Fe/Mo Order. Chem. Mater. 16 (22), 4337–4342. doi:10.1021/cm0493288
Iranmanesh, M., Lingg, M., Stir, M., and Hulliger, J. (2016). Sol Gel and Ceramic Synthesis of Sr2FeMo1−xWxO6 (0 ≤ x ≤ 1) Double Perovskites Series. RSC Adv. 6 (48), 42069–42075. doi:10.1039/c6ra03923e
Jalili, H., Heinig, N. F., and Leung, K. T. (2009). X-ray Photoemission Study of Sr2FeMoO6 and SrMoO4 Films Epitaxially Grown on MgO(001): Near-Surface Chemical-State Composition Analysis. Phys. Rev. B 79 (17), 174427. doi:10.1103/PhysRevB.79.174427
Kalanda, N., Kim, D.-H., Demyanov, S., Yu, S.-C., Yarmolich, M., Petrov, A., et al. (2018). Sr2FeMoO6 Nanosized Compound with Dielectric Sheaths for Magnetically Sensitive Spintronic Devices. Curr. Appl. Phys. 18 (1), 27–33. doi:10.1016/j.cap.2017.10.018
Kalanda, N., Turchenko, V., Karpinsky, D., Demyanov, S., Yarmolich, M., Balasoiu, M., et al. (2019). The Role of the Fe/Mo Cations Ordering Degree and Oxygen Non‐Stoichiometry on the Formation of the Crystalline and Magnetic Structure of Sr2FeMoO6−δ. Phys. Status Solidi B 256 (5), 1800278. doi:10.1002/pssb.201800278
Kalanda, N., Yarmolich, M., Petrov, A., Raevski, I., Kubrin, S., Raevskaya, S., et al. (2020). The Influence of Cation Ordering and Oxygen Nonstoichiometry on Magnetic Properties of Sr2FeMoO6−x Around Curie Temperature. J. Magnetism Magn. Mater. 500, 166386. doi:10.1016/j.jmmm.2019.166386
Kobayashi, K.-I., Kimura, T., Sawada, H., Terakura, K., and Tokura, Y. (1998). Room-Temperature Magnetoresistance in an Oxide Material with an Ordered Double-Perovskite Structure. Nature 395 (6703), 677–680. doi:10.1038/27167
Kobayashi, M., Tanaka, K., Fujimori, A., Ray, S., and Sarma, D. D. (2007). Critical Test for Altshuler-Aronov Theory: Evolution of the Density of States Singularity in Double Perovskite Sr2FeMoO6 with Controlled Disorder. Phys. Rev. Lett. 98 (24), 246401. doi:10.1103/PhysRevLett.98.246401
Lapshin, O. V., Boldyreva, E. V., and Boldyrev, V. V. (2021). Role of Mixing and Milling in Mechanochemical Synthesis (Review). Russ. J. Inorg. Chem. 66 (3), 433–453. doi:10.1134/S0036023621030116
Li, X. Y., Yao, Z. F., Zhang, L. Y., Zheng, G. H., Dai, Z. X., and Chen, K. Y. (2019). Generation of Oxygen Vacancies on Sr2FeMoO6 to Improve its Photocatalytic Performance through a Novel Preparation Method Involving pH Adjustment and Use of Surfactant. Appl. Surf. Sci. 480, 262–275. doi:10.1016/j.apsusc.2019.02.115
Lindén, J., Yamamoto, T., Karppinen, M., Yamauchi, H., and Pietari, T. (2000). Evidence for Valence Fluctuation of Fe in Sr2FeMoO6−w Double Perovskite. Appl. Phys. Lett. 76 (20), 2925–2927. doi:10.1063/1.126518
Lunghammer, S., Düvel, A., Posch, P., Kunert, B., Resel, R., and Wilkening, H. M. R. (2019). Self-diffusion and Ionic Exchange in Mechanosynthesized, Nanocrystalline Solid Solutions of PbF2 and CaF2 – 19F 2D NMR Visualizes the Flourine Hopping Preferences. Solid State Ionics 343, 115067. doi:10.1016/j.ssi.2019.115067
Maiti, P., Guha, P., Hussain, H., Singh, R., Nicklin, C., and Satyam, P. V. (2019). Microscopy and Spectroscopy Study of Nanostructural Phase Transformation from β-MoO3 to Mo under UHV - MBE Conditions. Surf. Sci. 682, 64–74. doi:10.1016/j.susc.2018.12.008
Menéndez, N., García-Hernández, M., Sánchez, D., Tornero, J. D., Martínez, J. L., and Alonso, J. A. (2004). Charge Transfer and Disorder in Double Perovskites. Chem. Mater. 16 (18), 3565–3572. doi:10.1021/cm049305t
Menil, F. (1985). Systematic Trends of the 57Fe Mössbauer Isomer Shifts in (FeOn) and (FeFn) Polyhedra. Evidence of a New Correlation between the Isomer Shift and the Inductive Effect of the Competing Bond T-X (→ Fe) (Where X Is O or F and T Any Element with a Formal Positive Charge). J. Phys. Chem. Sol. 46 (7), 763–789. doi:10.1016/0022-3697(85)90001-0
Menzel, M., Šepelák, V., and Becker, K. D. (2001). Mechanochemical Reduction of Nickel Ferrite. Solid State Ionics 141-142, 663–669. doi:10.1016/S0167-2738(01)00802-5
Mestl, G., Ruiz, P., Delmon, B., and Knozinger, H. (1994). Oxygen-Exchange Properties of MoO3: An In Situ Raman Spectroscopy Study. J. Phys. Chem. 98 (44), 11269–11275. doi:10.1021/j100095a007
Michalchuk, A. A. L., Boldyreva, E. V., Belenguer, A. M., Emmerling, F., and Boldyrev, V. V. (2021). Tribochemistry, Mechanical Alloying, Mechanochemistry: What Is in a Name? Front. Chem. 9, 685789. doi:10.3389/fchem.2021.685789
Michalchuk, A. A. L., Tumanov, I. A., and Boldyreva, E. V. (2019). Ball Size or Ball Mass - what Matters in Organic Mechanochemical Synthesis? CrystEngComm 21 (13), 2174–2179. doi:10.1039/c8ce02109k
Mon, J. P. (1972). Spectres de diffusion Raman de l'oxyde de strontium. J. Phys. Chem. Sol. 33 (6), 1257–1260. doi:10.1016/S0022-3697(72)80164-1
Nakamura, S., and Oikawa, K. (2003). Precise Structure Analysis Consistent with Mössbauer Quadrupole Effect: A Case of the Ordered Double Perovskites Sr2FeMO6 (M = Mo and Re). J. Phys. Soc. Jpn. 72 (12), 3123–3127. doi:10.1143/JPSJ.72.3123
Navarro, J., Frontera, C., Rubi, D., Mestres, N., and Fontcuberta, J. (2003). Aging of Sr2FeMoO6 and Related Oxides. Mater. Res. Bull. 38 (9-10), 1477–1486. doi:10.1016/S0025-5408(03)00171-5
Néel, L. (1949). Théorie du traînage magnétique des ferromagnétiques en grains fins avec application aux terres cuites. Ann. de géophysique 5, 99–136.
Ogale, A. S., Ogale, S. B., Ramesh, R., and Venkatesan, T. (1999). Octahedral Cation Site Disorder Effects on Magnetization in Double-Perovskite Sr2FeMoO6: Monte Carlo Simulation Study. Appl. Phys. Lett. 75 (4), 537–539. doi:10.1063/1.124440
O’Neill, R. T., and Boulatov, R. (2021). The Many Flavours of Mechanochemistry and its Plausible Conceptual Underpinnings. Nat. Rev. Chem. 5 (3), 148–167. doi:10.1038/s41570-020-00249-y
Pickhardt, W., Grätz, S., and Borchardt, L. (2020). Direct Mechanocatalysis: Using Milling Balls as Catalysts. Chem. Eur. J. 26 (57), 12903–12911. doi:10.1002/chem.202001177
Porcheddu, A., Colacino, E., De Luca, L., and Delogu, F. (2020). Metal-Mediated and Metal-Catalyzed Reactions under Mechanochemical Conditions. ACS Catal. 10 (15), 8344–8394. doi:10.1021/acscatal.0c00142
Porodko, O., Fabián, M., Kolev, H., Lisnichuk, M., Zukalová, M., Vinarčíková, M., et al. (2021). A Novel High Entropy Spinel-type Aluminate MAl2O4 (M = Zn, Mg, Cu, Co) and its Lithiated Oxyfluoride and Oxychloride Derivatives Prepared by One-step Mechanosynthesis. Z. Phys. Chem., 000010151520213106. doi:10.1515/zpch-2021-3106
Putz, H., and Brandenburg, K. (2019). Diamond – Crystal and Molecular Structure Visualization. Version 4.6. Bonn: Crystal Impact.
Reyes, A. M., Arredondo, Y., and Navarro, O. (2016). Effect of Cationic Disorder on the Magnetic Moment of Sr2FeMoO6: Ab Initio Calculations. J. Phys. Chem. C 120 (7), 4048–4052. doi:10.1021/acs.jpcc.6b00100
Saloaro, M., Hoffmann, M., Adeagbo, W. A., Granroth, S., Deniz, H., Palonen, H., et al. (2016). Toward Versatile Sr2FeMoO6-Based Spintronics by Exploiting Nanoscale Defects. ACS Appl. Mater. Inter. 8 (31), 20440–20447. doi:10.1021/acsami.6b04132
Sarma, D. D., Mahadevan, P., Saha-Dasgupta, T., Ray, S., and Kumar, A. (2000b). Electronic Structure of Sr2FeMoO6. Phys. Rev. Lett. 85 (12), 2549–2552. doi:10.1103/PhysRevLett.85.2549
Sarma, D. D., Sampathkumaran, E. V., Ray, S., Nagarajan, R., Majumdar, S., Kumar, A., et al. (2000a). Magnetoresistance in Ordered and Disordered Double Perovskite Oxide, Sr2FeMoO6. Solid State. Commun. 114 (9), 465–468. doi:10.1016/S0038-1098(00)00079-X
Schlem, R., Burmeister, C. F., Michalowski, P., Ohno, S., Dewald, G. F., Kwade, A., et al. (2021). Energy Storage Materials for Solid‐State Batteries: Design by Mechanochemistry. Adv. Energ. Mater. 11 (30), 2101022. doi:10.1002/aenm.202101022
Šepelák, V., Becker, K. D., Bergmann, I., Suzuki, S., Indris, S., Feldhoff, A., et al. (2009). A One-step Mechanochemical Route to Core−Shell Ca2SnO4 Nanoparticles Followed by 119Sn MAS NMR and 119Sn Mössbauer Spectroscopy. Chem. Mater. 21 (12), 2518–2524. doi:10.1021/cm900590d
Šepelák, V., and Becker, K. D. (2000). Mössbauer Studies in the Mechanochemistry of Spinel Ferrites. J. Mater. Synth. Process. 8 (3-4), 155–166. doi:10.1023/A:1011355908538
Šepelák, V., Bégin-Colin, S., and Le Caër, G. (2012a). Transformations in Oxides Induced by High-Energy Ball-Milling. Dalton Trans. 41 (39), 11927–11948. doi:10.1039/c2dt30349c
Šepelák, V., Bergmann, I., Feldhoff, A., Heitjans, P., Krumeich, F., Menzel, D., et al. (2007). Nanocrystalline Nickel Ferrite, NiFe2O4: Mechanosynthesis, Nonequilibrium Cation Distribution, Canted Spin Arrangement, and Magnetic Behavior. J. Phys. Chem. C 111 (13), 5026–5033. doi:10.1021/jp067620s
Šepelák, V., Da Silva, K. L., Trautwein, R. S., Becker, K. D., and Hahn, H. (2021). Unusual Cation Coordination in Nanostructured Mullites. Z. Phys. Chem., 000010151520213101. doi:10.1515/zpch-2021-3101
Šepelák, V., Düvel, A., Wilkening, M., Becker, K.-D., and Heitjans, P. (2013). Mechanochemical Reactions and Syntheses of Oxides. Chem. Soc. Rev. 42 (18), 7507–7520. doi:10.1039/c2cs35462d
Šepelák, V., Feldhoff, A., Heitjans, P., Krumeich, F., Menzel, D., Litterst, F. J., et al. (2006). Nonequilibrium Cation Distribution, Canted Spin Arrangement, and Enhanced Magnetization in Nanosized MgFe2O4 Prepared by a One-step Mechanochemical Route. Chem. Mater. 18 (13), 3057–3067. doi:10.1021/cm0514894
Šepelák, V., Menzel, M., Becker, K. D., and Krumeich, F. (2002). Mechanochemical Reduction of Magnesium Ferrite. J. Phys. Chem. B 106 (26), 6672–6678. doi:10.1021/jp020270z
Šepelák, V., Myndyk, M., Fabián, M., Silva, K. L. D., Feldhoff, A., Menzel, D., et al. (2012b). Mechanosynthesis of Nanocrystalline Fayalite, Fe2SiO4. Chem. Commun. 48 (90), 11121–11123. doi:10.1039/c2cc36370d
Shimada, T., Nakamura, J., Motohashi, T., Yamauchi, H., and Karppinen, M. (2003). Kinetics and Thermodynamics of the Degree of Order of the B Cations in Double-Perovskite Sr2FeMoO6. Chem. Mater. 15 (23), 4494–4497. doi:10.1021/cm030409y
Skurikhina, O., Senna, M., Fabián, M., Witte, R., Tarasenko, R., Tkáč, V., et al. (2020). A Sustainable Reaction Process for Phase Pure LiFeSi2O6 with Goethite as an Iron Source. Ceramics Int. 46 (10), 14894–14901. doi:10.1016/j.ceramint.2020.03.016
Skutina, L., Filonova, E., Medvedev, D., and Maignan, A. (2021). Undoped Sr2MMoO6 Double Perovskite Molybdates (M = Ni, Mg, Fe) as Promising Anode Materials for Solid Oxide Fuel Cells. Materials 14 (7), 1715. doi:10.3390/ma14071715
Solares-Briones, M., Coyote-Dotor, G., Páez-Franco, J. C., Zermeño-Ortega, M. R., de la O Contreras, C. M., Canseco-González, D., et al. (2021). Mechanochemistry: A Green Approach in the Preparation of Pharmaceutical Cocrystals. Pharmaceutics 13 (6), 790. doi:10.3390/pharmaceutics13060790
Son, L. H., Phuc, N. X., Phuc, P. V., Hong, N. M., and Hong, L. V. (2001). Observation of Phase Decomposition of Sr2FeMoO6 by Raman Spectroscopy. J. Raman Spectrosc. 32 (10), 817–820. doi:10.1002/jrs.764
Suchaneck, G., Kalanda, N., Artsiukh, E., and Gerlach, G. (2020). Challenges in Sr2FeMoO6−δ Thin Film Deposition. Phys. Status Solidi B 257 (3), 1900312. doi:10.1002/pssb.201900312
Sujatha, R. A., Flower, N. A. L., Vinitha, G., Sharath, R. A., and Rahulan, K. M. (2019). Structural and Non-linear Optical Response of Er3+ Doped SrMoO4 Nanostructures. Appl. Surf. Sci. 490, 260–265. doi:10.1016/j.apsusc.2019.06.086
Suominen, T., Raittila, J., Salminen, T., Schlesier, K., Lindén, J., and Paturi, P. (2007). Magnetic Properties of Fine SFMO Particles: Superparamagnetism. J. Magnetism Magn. Mater. 309 (2), 278–284. doi:10.1016/j.jmmm.2006.07.016
Szczęśniak, B., Choma, J., and Jaroniec, M. (2021). Recent Advances in Mechanochemical Synthesis of Mesoporous Metal Oxides. Mater. Adv. 2 (8), 2510–2523. doi:10.1039/d1ma00073j
Tóthová, E., Tarasenko, R., Tkáč, V., Orendáč, M., Hegedüs, M., Danková, Z., et al. (2019). Microcrystalline Gd2MoO6 Prepared by Combined Mechanochemical/Thermal Process and its Magnetic Properties. J. Mater. Sci. 54 (8), 6111–6121. doi:10.1007/s10853-019-03331-z
Tóthová, E., Witte, R., Hegedüs, M., Senna, M., Hahn, H., Heitjans, P., et al. (2018). Mechanochemical Syntheses of LiFeGe2O6-Based Nanocomposite and Novel Nanoglassy LiFeTi2O6. J. Mater. Sci. 53 (19), 13530–13537. doi:10.1007/s10853-018-2405-2
Tsuzuki, T. (2021). Mechanochemical Synthesis of Metal Oxide Nanoparticles. Commun. Chem. 4 (1), 143. doi:10.1038/s42004-021-00582-3
Valdés, J., Reséndiz, D., Cuán, Á., Nava, R., Aguilar, B., Cortés-Romero, C. M., et al. (2021). Sol-Gel Synthesis of the Double Perovskite Sr2FeMoO6 by Microwave Technique. Materials 14 (14), 3876. doi:10.3390/ma14143876
Valenzuela, J. L., Soto, T. E., Lemus, J., Navarro, O., and Morales, R. (2014). Reaction Kinetics of the Double Perovskite Sr2FeMoO6 by Gas-Solid Reactions. Physica B: Condensed Matter 455, 10–13. doi:10.1016/j.physb.2014.07.034
Wilkening, M., Düvel, A., Preishuber-Pflügl, F., Da Silva, K., Breuer, S., Šepelák, V., et al. (2017). Structure and Ion Dynamics of Mechanosynthesized Oxides and Fluorides. Z. Krist.-Cryst. Mater. 232 (1−3), 107–127. doi:10.1515/zkri-2016-1963
Xi, X., Liu, J., Fan, Y., Wang, L., Li, J., Li, M., et al. (2021). Reducing d-p Band Coupling to Enhance CO2 Electrocatalytic Activity by Mg-Doping in Sr2FeMoO6−δ Double Perovskite for High Performance Solid Oxide Electrolysis Cells. Nano Energy 82, 105707. doi:10.1016/j.nanoen.2020.105707
Yarmolich, M., Kalanda, N., Demyanov, S., Fedotova, J., Bayev, V., and Sobolev, N. A. (2016). Charge Ordering and Magnetic Properties in Nanosized Sr2FeMoO6−δ Powders. Phys. Status Solidi B 253 (11), 2160–2166. doi:10.1002/pssb.201600527
Yu, S. C., Song, Y. Y., Kiss, L. F., and Vincze, I. (1999). Study on the Magnetic Behavior of Nanogranular Cu80Fe10Co10 Solid Solution. J. Magn. Magn. Mater. 203 (1-3), 316–318. doi:10.1016/S0304-8853(99)00214-0
Zhang, Q., and Saito, F. (2012). A Review on Mechanochemical Syntheses of Functional Materials. Adv. Powder Tech. 23 (5), 523–531. doi:10.1016/j.apt.2012.05.002
Keywords: mechanochemistry, mechanochemical synthesis, double perovskite, anti-site disorder, Mössbauer spectroscopy
Citation: Tóthová E, Düvel A, Witte R, Brand RA, Sarkar A, Kruk R, Senna M, Da Silva KL, Menzel D, Girman V, Hegedüs M, Baláž M, Makreski P, Kubuki S, Kaňuchová M, Valíček J, Hahn H and Šepelák V (2022) A Unique Mechanochemical Redox Reaction Yielding Nanostructured Double Perovskite Sr2FeMoO6 With an Extraordinarily High Degree of Anti-Site Disorder. Front. Chem. 10:846910. doi: 10.3389/fchem.2022.846910
Received: 31 December 2021; Accepted: 25 January 2022;
Published: 16 March 2022.
Edited by:
Elena Vladimirovna Boldyreva, Novosibirsk State University, RussiaReviewed by:
Martin Wilkening, Graz University of Technology, AustriaPaulo Filho Marques De Oliveira, University of São Paulo, Brazil
Copyright © 2022 Tóthová, Düvel, Witte, Brand, Sarkar, Kruk, Senna, Da Silva, Menzel, Girman, Hegedüs, Baláž, Makreski, Kubuki, Kaňuchová, Valíček, Hahn and Šepelák. This is an open-access article distributed under the terms of the Creative Commons Attribution License (CC BY). The use, distribution or reproduction in other forums is permitted, provided the original author(s) and the copyright owner(s) are credited and that the original publication in this journal is cited, in accordance with accepted academic practice. No use, distribution or reproduction is permitted which does not comply with these terms.
*Correspondence: Vladimír Šepelák, dmxhZGltaXIuc2VwZWxha0BraXQuZWR1