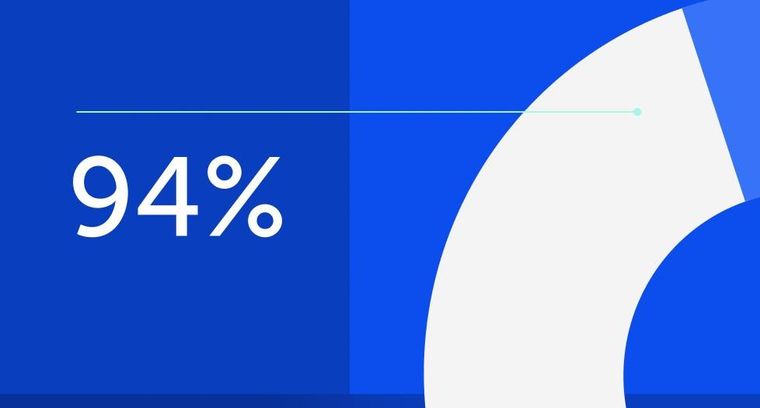
94% of researchers rate our articles as excellent or good
Learn more about the work of our research integrity team to safeguard the quality of each article we publish.
Find out more
ORIGINAL RESEARCH article
Front. Chem., 01 March 2022
Sec. Catalysis and Photocatalysis
Volume 10 - 2022 | https://doi.org/10.3389/fchem.2022.845274
Subnanometer noble metal clusters have attracted much attention because of abundant low-coordinated metal atoms that perform excellent catalytic activity in various catalytic processes. However, the surface free energy of metals increases significantly with decreasing size of the metal clusters, which accelerates the aggregation of small clusters. In this work, new Schiff base–imidazole-functionalized MOFs were successfully synthesized via the postsynthetic modification method. Highly dispersed Pd clusters with an average size of 1.5 nm were constructed on this functional MOFs and behaved excellent catalytic activity in the Suzuki coupling of phenyboronic acid and bromobenzene (yield of biaryl >99%) under mild reaction conditions. Moreover, the catalyst can be reused six times without loss of activity. Such catalytic behavior is found to closely related to the surface functional groups that promote the formation of small Pd0 clusters in the metallic state.
Subnanometer noble metal clusters (SNMCs) with dimensions in the range from a few to dozens of angstroms have drawn focused attention (Diez and Ras, 2011; Imaoka et al., 2017; Wang et al., 2020; Yamamoto et al., 2020; Dong et al., 2021). With most of the constitutional atoms exposed to the surface, which is an unsaturated coordination environment and have higher reactivity than those in the bulk, SNMCs perform excellent catalytic activity and selectivity when serving as catalysts, thus, dramatically increasing the utilization efficiency of noble metals. The catalytic activity of SNMCs will be further improved as the size decreases, due to the surface atomic structure, electronic structure, and defects will change significantly. However, because of high surface energy, a too tiny size could lead to aggregation and particle growth (Tang et al., 2017; Si et al., 2018; Xin et al., 2018). A solution to the critical issue is to apply a nanoporous supporting material, such as graphene (Gu et al., 2017; Liu et al., 2019), carbon nanofibers (Eid et al., 2019), C3N4 (Yang et al., 2021; Zhang et al., 2021), and porous SiO2 (Peng et al., 2019). Nevertheless, some disadvantages, such as leaching and aggregation of SNMCs, exist during the catalytic process by using these materials as support, which, thus, weaken the catalytic activity (Gu et al., 2017; Liu et al., 2019; Zhang et al., 2021). Therefore, it is of great importance to design supporting materials that cannot only make ultrafine SNMCs with uniformity and high dispersion but also endow the obtained catalysts with excellent catalytic activity.
Metal−organic frameworks (MOFs) are a fascinating family of porous crystalline materials assembled with inorganic metal nodes and organic linkers, possessing large internal surface areas, well-defined structures, and tunable chemical properties (Lee et al., 2009; Li et al., 2012; Furukawa et al., 2013). These features confer their popular applications in heterogeneous catalysis, particularly when they are used as support for noble metal (e.g., Pd, Au, Ru, and Pt) nanoparticles (MNPs) (He et al., 2018; Han et al., 2019; Chen et al., 2021; Khan et al., 2021). On the one hand, the permanent porosity and uniform channels of MOFs make them particularly suitable for the efficient immobilization of MNPs that protect the MNPs from sintering and aggregation without inhibiting the diffusion of the reactants and products. On the other hand, the organic linkers or the metal nods of MOFs can also be used as unique functional moieties, and the interaction between MOFs and MNPs can trigger excellent synergistic effects, affording properties that are superior to the individual components (Fang et al., 2018; He et al., 2018; Mouarrawis et al., 2018; Han et al., 2019; Khan et al., 2021). Su et al. constructed a series of novel MOFs based on redox-active tetrathiafulvalene (TTF) organic linkers. Ultra-small noble metal (Ag, Pd, and Au) nanoparticles were successfully generated in situ and stabilized in the MOF cavity due to the reductive TTF moieties (Su et al., 2020). Recently, Guo and coworkers synthesized highly dispersed ultrafine platinum (Pt) particles with a size of <1.5 nm anchored onto amino group-functionalized MOFs (NH2–Ce–MOFs). It was found that the presence of –NH2 groups in NH2–Ce–MOFs played a crucial role in anchoring Pt species with high dispersion on the MOF framework (Guo et al., 2021). Based on these reports, the development of SNMC/MOF materials for noble metal catalysis will exert a significant impact on conventional chemical industrial processes.
In this work, we constructed a novel Schiff base–imidazole-functionalized MOFs and apply the MOFs as support to anchor Pd0. Pd0 clusters were uniformly dispersed in the MOFs with an average size of 1.5 nm, which is a very small size by using MOFs as support, as we know. The sub-1.5 nm Pd0 cluster-supported MOFs performed excellent activity in the Suzuki coupling reactions and behaved with high stability.
All chemicals and reagents were obtained from commercial sources and used as received. Specifically, zirconium chloride (ZrCl4), anhydrous N,N-dimethylformamide (DMF), 2-aminoterephthalic acid (H2BDC–NH2), 4-imidazolecarboxaldehyde (Im-CHO), and palladium acetate (Pd(OAc)2) were purchased from Aladdin.
UIO-66–NH2 was prepared following a procedure previously reported (Zhang et al., 2016) with slight modifications. In a typical synthetic process, ZrCl4 (0.24 g, 1.029 mmol) was dissolved in DMF (60 ml) by sonication for 5 min. Then the ligand H2BDC–NH2 (0.186 g, 1.029 mmol) and deionized water (0.15 ml) successively dropped into the mixture, and stirring was initiated until all precursors were completely dissolved. The obtained mixture was transferred into a 100-ml Teflon-lined stainless-steel autoclave, and kept at 393 K for 24 h under static conditions. After cooled to room temperature (r.t.), the obtained yellow precipitate was separated by centrifugation at 10,000 rpm for 5 min and thoroughly washed with DMF and methanol three times, respectively. Finally, the yellow powder was freeze dried in a freeze dryer prior to experiments.
The Schiff base–imidazole-functionalized MOFs was prepared by a postmodification strategy. Typically, UIO-66–NH2 (0.175 g, 0.1 mmol) was dispersed in 30 ml of ethanol by sonication for 5 min. Then Im-CHO (0.144 g, 1.5 mmol) was added into the mixture, and the resulting mixture was refluxed at 80°C for 24 h. The formed yellow solid was filtered and washed with abundant ethanol three times and then freeze dried in a freeze dryer.
The acetone solution of Pd(OAc)2 was first prepared by dissolving Pd(OAc)2 (0.02 g) in 5 ml of acetone. Then UIO-66-SB-Im (0.2 g) was added into the above solution, and the mixture was subsequently stirred at room temperature (r.t.) for 24 h. Afterward, the precipitate was collected by centrifugation and washed with abundant acetone three times. The as-synthesized sample was freeze dried in a freeze dryer and denoted as Pd2+@UIO-66–SB–Im. The Pd2+ in the as-synthesized sample Pd2+@UIO-66–SB–Im was subsequently reduced to Pd0 in a stream of 5% H2/N2 (75 cm3/min), with the temperature ramped from 20°C to 200°C (at 3°C/min) and held at 200°C for 2 h to obtain Pd0@UIO-66–SB–Im. For contrast, Pd0@UIO-66–NH2 was prepared through a similar process by changing the support to UIO-66–NH2.
Pd contents were estimated on an OPTMA 20,000-V inductive coupled plasma mass spectroscopy (ICP). Elemental analyses were performed on a CHN elemental analyzer Vario EL cube. Power X-ray diffraction (PXRD) patterns were recorded on a Panalytical X’Pert3 Powder diffractometer with Cu Kα radiation (λ = 1.5406 Å) from 2θ = 5°–80°, at a scan rate of 0.2° s−1, with the beam voltage and current of 45 kV and 200 mA, respectively. Scanning electron microscopy (SEM) images were recorded on an FEI Nova Nano SEM 450 Prime scanning electron microscope. Transmission electron microscopy (TEM) images were obtained from a JOEL JEM-1400plus transmission electron microscope at an accelerating voltage of 200 kV. Fourier transform infrared spectroscopy (FTIR) spectra in the wavenumber range of 4,000–800 cm−1 were recorded on an Agilent Cary 660 FTIR Spectrometer. The nitrogen (N2) sorption isotherms were measured at the temperature of liquid nitrogen (77 K) by using a BELSORP-MINI analyzer with the samples being degassed at 100°C for 3 h before analysis. The surface area and pore-size distribution curves were calculated by the Brunauer–Emmett–Teller (BET) and density functional theory (DFT) method, respectively. X-ray photoelectron spectroscopy (XPS) measurement was performed on ESCALAB 250Xi with a monochromatic Mg-Kα source operated at 20 kV. All binding energies were calibrated by using the contaminant carbon (C1s = 284.8 eV) as the reference.
The Pd-catalyzed Suzuki coupling reactions of phenyboronic acid and bromobenzene were carried out in a 25-ml Schlenk tube. Generally, phenyboronic acid (3 mmol), bromobenzene (2 mmol), NaHCO3 (4 mmol), Pd0@UIO-66–SB–Im (0.103 mol% Pd based on bromobenzene), dimethylacetamide (DMA, 5 ml), and 550 µl of diethyeneoxide (internal standard) were successively added into a 25-ml Schlenk tube. The tube was closed and placed in a preheated oil bath. The reaction was conducted under stirring at 90°C for 7 h. After the reaction, the tube was cooled on ice to quench the reaction, and Pd0@UIO-66–SB–Im was separated from the reaction medium by centrifugation and the products were analyzed by gas chromatograph [GC: Agilent GC6890N with a flame ionization detector (FID) and an HP-5 column (30 m, 0.25 mm inner diameter)]. The yield was determined with a calibration curve method.
For investigating the heterogeneous nature of the catalyst Pd0@UIO-66–SB–Im, a hot filtration test was applied. A mixture of phenyboronic acid (3 mmol), bromobenzene (2 mmol), NaHCO3 (4 mmol), dimethylacetamide (DMA, 5 ml) and the catalyst Pd0@UIO-66-SB-Im (0.103 mol% Pd based on bromobenzene) was stirred at 90°C. After 1.5 h, the catalyst was separated by centrifugation, the reaction solution was still stirred for 4.5 h, and the products were detected by GC at the reaction times of 0.5, 1.5, 2.5, 3.5, and 4.5 h.
In order to test the durability of the Pd0@UIO-66–SB–Im in the Suzuki coupling reaction system, the Pd0@UIO-66–SB–Im was recovered from the reaction mixture by centrifugation after the completion of previous catalytic reaction cycle, and washed with deionized water and ethanol, freeze dried in a freeze dryer, and then directly charged to the next run.
The preparation of UIO-66–SB–Im and immobilization of Pd0 species to UIO-66–SB–Im are illustrated in Scheme 1. Briefly, UIO-66–NH2 was hydrothermally synthesized through the coordination of metal source (ZrCl4) and organic linker (2-aminoterephthalic acid, H2BDC-NH2), followed by an aldimine condensation process with 4-imidazolecarboxaldehyde (Im-CHO) to yield UIO-66–SB–Im support. Pd0@UIO-66–SB–Im catalyst was prepared by incorporating the precursor Pd(OAc)2 into the Schiff base–imidazole-functionalized MOFs via coordination and subsequent H2 reduction. Elemental analyses indicate that the N contents of UIO-66–NH2 and UIO-66–SB–Im are 4.76% and 10.23%, respectively, implying the integration of Im-CHO moieties into the UIO-66–NH2 matrix (Table 1). Pd2+@UIO-66–SB–Im and Pd0@UIO-66–SB–Im have a similar N content on the MOF skeleton, suggesting the stability of the surface groups and framework of UIO-66–SB–Im during the Pd immobilization process (Table 1). ICP analysis shows that Pd0@UIO-66–SB–Im contains a high Pd content of 2.3 wt%.
Power X-ray diffraction (PXRD) was used to characterize the crystallinity of the prepared samples. As shown in Figure 1, the experimental PXRD pattern of the synthesized UiO-66–NH2 matches well with the simulated one (Gang et al., 2018), indicating the successful preparation of UiO-66–NH2. After the aldimine condensation, the resulting product UIO-66–SB–Im performs the same PXRD pattern with UiO-66–NH2, suggesting that the crystallinity and structure of MIL-101 are well retained after the postsynthesis. The crystallinity and structure are still retained after loading Pd(OAc)2 and subsequent H2 reduction. Moreover, the diffraction peak of Pd nanoparticles (NPs) at 2θ = 40.1° was not detected in Pd0@UIO-66–SB–Im, revealing that Pd NPs could be small.
FIGURE 1. Power X-ray diffraction (PXRD) diffraction patters of UIO-66–NH2, UIO-66–SB–Im, Pd2+@UIO-66–SB–Im, and Pd0@UIO-66–SB–Im.
Scanning electron microscopy (SEM) imaging shows that UIO-66–SB–Im (Figure 2A) performs similar morphology with UIO-66–NH2, which is composed of nanometer-leveled particles with the size in range of 100–150 nm. After loading Pd species, the morphology of Pd2+@UIO-66–SB–Im and Pd0@UIO-66–SB–Im were retained (Figures 2B, C), implying that UIO-66–NH2 is stable after functionalization. Elemental (Zr, N, and Pd) mapping images of Pd2+@UIO-66–SB–Im further prove the successful introduction of Pd species (Figure 2D). The TEM images and Pd size distribution of Pd0@UIO-66–SB–Im are given in Figure 3E. From the picture, the Pd clusters were uniformly distributed with average size of 1.5 nm, belonging to sub-2-nanometer size. Apart from this, the lattice spacing of Pd clusters was about 0.22 nm, ascribed to the Pd(111) crystal face (Zhao et al., 2014), which is active for Suzuki coupling reactions.
FIGURE 2. Scanning electron microscopy (SEM) images of (A) UIO-66–NH2, (B) UIO-66–SB–Im, (C) Pd2+@UIO-66–SB–Im, (D) Pd0@UIO-66–SB–Im, (E) EDS mapping images for elements Zr, N, Pd elements of Pd2+@UIO-66–SB–Im, transmission electron microscopy (TEM) images of (F) Pd0@UIO-66–SB–Im.
FIGURE 3. (A) Nitrogen (N2) adsorption–desorption isotherms and (B) pore size distribution curves of different samples.
Quantitative porous properties of UIO-66–NH2, UIO-66–SB–Im, Pd2+@UIO-66–SB–Im, and Pd0@UIO-66–SB–Im are established by the N2 sorption analysis. Figure 3 illustrates the N2 sorption isotherms (Figure 3A) and corresponding pore size distribution curves (Figure 3B). The UIO-66–SB–Im exhibits similar N2 sorption isotherms with UIO-66–NH2 than a typical I type isotherm with a dramatic increase in nitrogen uptake in the partial pressure range of p/p0<0.1, characteristic of classical microporosity (Chen et al., 2018). The BET (Brunauer–Emmett–Teller) surface area and the total pore volume of UIO-66–SB–Im are 537 m2 g−1 and 0.3 cm3 g−1, respectively (Table 1). The decrease in the surface area and pore volume of UIO-66–SB–Im, in comparison with those of UIO-66–NH2 (709 m2 g−1 with a volume of 0.40 cm3 g−1), may be ascribed to the partial occupation of the cavities in NH2–UIO-66 by the Schiff base and imidazole groups. The pore-size distribution curve calculated by the DFT (discrete Fourier transform) method further reveals the existence of micropores with a narrow pore size distribution in UIO-66–SB–Im. After loading Pd, Pd2+@UIO-66–SB–Im and Pd0@UIO-66–SB–Im performs similar sorption isotherms with their parent UIO-66–SB–Im, revealing the preservation of porosity during the Pd-loading step. The BET surface areas of Pd2+@UIO-66–SB–Im and Pd0@UIO-66–SB–Im is 428 and 452 m2g−1, respectively, which are smaller than that of UIO-66–SB–Im due to the partial pore filling by the deposited Pd species and the weakly decreased proportion of porous structure after Pd loading (Wang et al., 2016; Liu et al., 2021). The BET surface area and pore volume of Pd0@UIO-66–SB–Im increased slightly with subsequent H2 reduction, which could be ascribed to the removal of OAc− from the precursor during the reduction process (Liu et al., 2021).
The structure of the samples and the relationship between Pd and the support UIO-66–SB–Im were further analyzed by Fourier transform infrared spectroscopy (FTIR). As displayed in Figure 4, the FTIR spectrum of UIO-66–NH2 shows a peak at 1,340 cm−1 that attributed to the C−N stretch vibration on benzene ring (Lin et al., 2012), while those at 3,478 and 3,371 cm−1 could be assigned to the asymmetric and symmetric vibrations of −NH2, which further demonstrate the successful synthesis of UIO-66–NH2 (Lin et al., 2012). After the aldimine condensation with Im-CHO, several peaks appeared in the FTIR spectrum of UIO-66-SB-Im compared with that of UIO-66–NH2. The new peak at 1,648 cm−1 could be ascribed to the stretch vibration of C=N and featured band of imidazole (Lin et al., 2012; Gang et al., 2018), indicating the successful introduction of Im-CHO and successful formation of Schiff base. After anchoring Pd(OAc)2 on UIO-66–SB–Im, the peak at 1,648 cm−1 corresponding to the Schiff base and imidazole groups is shifted to 1,675 cm−1, suggesting the interaction of the Schiff base and imidazole groups in UIO-66–SB–Im with Pd(OAc)2 (Wang et al., 2015; Liu et al., 2017). A similar phenomenon occurred after H2 reduction, implying the interaction of Schiff base and imidazole groups in UIO-66–SB–Im with Pd clusters.
FIGURE 4. Fourier transform infrared spectroscopy (FTIR) spectra of UIO-66–NH2, UIO-66–SB–Im, Pd2+@UIO-66–SB–Im, and Pd0@UIO-66–SB–Im.
Surface analyses of UIO-66–NH2, UIO-66–SB–Im, Pd2+@UIO-66–SB–Im, and Pd0@UIO-66–SB–Im were carried out using X-ray photoelectron spectroscopy (XPS), and the results are displayed in Figure 5. Three signals were observed at the binding energies of 181.5 eV (Zr3d), 228.4 eV (C1s), and 399.1 eV (N1s) in the survey scan XPS spectrum of UIO-66–SB–Im, manifesting the existence of Zr, C, and N elements (Figure 5A) (Gang et al., 2018). The high-resolution Zr3d XPS spectrum was deconvoluted into four peaks at 182.9 and 185.3 eV, which are ascribed to Zr3d5/2 and Zr3d3/2, respectively (Figure 5B) (Gang et al., 2018). The high-resolution N1s XPS spectrum was fitted with three peaks at 398.2, 399.5, 400.6 eV, which correspond to the imine groups (−N=CH−) and imidazole N (Wang et al., 2015; Gang et al., 2018), respectively. After loading Pd, a new signal at binding energy of 338.7 eV (Pd3d) appeared in the survey scan XPS spectra of Pd2+@UIO-66–SB–Im and Pd0@UIO-66–SB–Im, further proving the successful introduction of Pd species into the support (Figure 5A). The high-resolution Zr3d XPS spectra of Pd2+@UIO-66–SB–Im and Pd0@UIO-66–SB–Im fitted likewise the two peaks with no other oxidation state of Zr peaks, indicating that the Zr was chemically stable during the functionalization of the linker and anchoring of Pd precursor (Gang et al., 2018). Moreover, compared with UIO-66–SB–Im, the peaks of Zr in Pd2+@UIO-66–SB–Im and Pd0@UIO-66–SB–Im was slightly shifted to lower energy. The phenomenon implies that the oxidation state of Zr4+ in Pd2+@UIO-66–SB–Im and Pd0@UIO-66–SB–Im was reduced, which may be due to the electron transfer from Pd to Zr on the support (Gang et al., 2018). The high-resolution NIs XPS spectra of Pd2+@UIO-66–SB–Im was fitted with three peaks at 398.5, 399.8, and 400.9 eV, which shift positively by 0.3 eV compared with UIO-66–SB–Im. The phenomenon indicates the interaction of Schiff base and imidazole with Pd (Liu et al., 2017). The high-resolution Pd3d XPS spectrum of Pd2+@UIO-66–SB–Im exhibits two peaks at 338.1 and 343.5 eV, assignable to Pd2+3d5/2 and Pd2+3d3/2, respectively, indicating that the surface Pd species of Pd2+@UIO-66–SB–Im are in a Pd2+ state (Figure 5D) (Liu et al., 2017; Liu et al., 2018). After reduction, Pd0@UIO-66–SB–Im exhibits only two Pd03d peaks at 335.5 (3d5/2) and 340.8 eV (3d3/2), implying that Pd species of Pd0@UIO-66–SB–Im are all in the metallic state (Figure 5D) (Liu et al., 2021).
FIGURE 5. (A) Survey scan, (B) Zr 3d, (C) N 1s, and (D) Pd 3d X-ray photoelectron spectroscopy (XPS) spectra of UIO-66–NH2, UIO-66–SB–Im, Pd2+@UIO-66–SB–Im, and Pd0@UIO-66–SB–Im.
The catalytic performance of Pd0@UIO-66–SB–Im was assessed in the Suzuki coupling reaction between bromobenzene and phenylboronic acid at mild conditions. The activities of different catalysts were parallel investigated under the same reaction conditions, and the results are summarized in Table 2. The reaction does not proceed in the absence of catalysts or catalyzed by the pure support UIO-66–NH2 or UIO-66–SB–Im (Table 2, entries 1–3). The material Pd0@UIO-66–SB–Im behaved with 95.3% yield of biphenyl, indicating that the Pd0 species are the active species in the reaction (Table 2, entry 4). Moreover, the contrast catalyst Pd0@UIO-66–NH2 was synthesized and investigated in the reaction. Pd0@UIO-66–NH2 only performed 89.4% yield of biphenyl (Table 2, entry 5). The results show that the Pd0@UIO-66–NH2 is the best catalyst for the reaction.
The Suzuki coupling of bromobenzene and phenylboronic acid to biphenyl was further investigated by using the catalyst Pd0@UIO-66–SB–Im under different reactions with respect to the amount of catalyst, the amount of base, the reaction time, and the reaction temperature (Figure 6). Moreover, Pd0@UIO-66–NH2 was also parallel investigated for comparison (Figure 6). The yield as the function of the catalyst amount exhibits a “volcanic” type curve. Initially, the yield initially increases gradually when the catalyst amount increases (Figure 6A). At up to 0.063 mol%, the yield reaches the highest value of 99.4%. A further increase in the catalyst amount causes a slight decrease in yield that could be attributed to the over-oxidation of the product along with the reaction (Lin et al., 2012). For Pd0@UIO-66–NH2, more catalyst dosage (0.093 mol%) was needed to reach the highest biphenyl yield, and the yield is only 98.9%. Fixing the catalyst amount of Pd0@UIO-66–SB–Im at 0.063 mol%, the base NaHCO3 amount was studied (Figure 6B). A higher number of base favors higher biphenyl yield. In view of environmental impact, 6 mmol is suitable. For Pd0@UIO-66–NH2, more amount of base is needed (>8 mmol) to reach the yield of 99%, which will cause more waste of chemicals and is environmentally unfriendly (Figure 6B). The survey of the reaction time and temperature suggests that moderate reaction time (6 h) and temperature (90°C) benefit high yield of biphenyl because of the over-oxidation with elongating time or higher temperature (Figures 5E, F). The above investigation shows that Pd0@UIO-66–SB–Im is highly efficient for the Suzuki coupling reaction under mild condition. Compared with Pd0@UIO-66–NH2, the superior activity of Pd0@UIO-66–NH2–MC is probably ascribed to the cooperation between Pd0 clusters and the Schiff base–imidazole-functionalized UIO-66–SB–Im.
FIGURE 6. The relationship between the activity and (A) catalyst amount, (B) base amount, (C) reaction time, and (D) reaction temperature in the Suzuki coupling reaction. Pd0@UIO-66–SB–Im catalyzed reaction conditions: Pd0@UIO-66–SB-Im (0.06 mol% based on bromobenzene), bromobenzene (2 mmol), phenylboronic acid (3 mmol), NaHCO3 (6 mmol), DMA (5 ml), 6 h, 90°C. For each plot, a specific parameter is changed. Pd0@UIO-66–NH2 catalyzed reaction conditions: Pd0@UIO-66–NH2 (0.09 mol% based on bromobenzene), bromobenzene (2 mmol), phenylboronic acid (3 mmol), NaHCO3 (8 mmol), DMA (5 ml), 8 h, 120°C. For each plot, a specific parameter is changed.
The scope of Pd0@UIO-66–SB–Im is further extended to the Suzuki coupling of various aryl halides with phenylboronic acid (Table 3). Pd0@UIO-66–SB–Im-catalyzed coupling of bromobenzene with electron-withdrawing groups (nitro, aldehyde, or methoxy group) performed high yields of the corresponding biphenyl products (Table 3, entries 1–3), while coupling of bromobenzene bearing electron-donating groups (methyl group) behaved with lower yields to the coupling products (Table 3, entries 4–6), implying that the withdrawing groups in bromobenzene is beneficial to the reaction, while the electron-donating groups in bromobenzene is harmful (Shang et al., 2014). Coupling of aryl iodobenzene with phenylboronic acid produced >99% yield of biphenyl (Table 3, entry 7), while coupling of chlorobenzene only obtained 37% yield of biphenyl (Table 3, entry 8) because the C–X (X = Cl, Br, I) bond dissociation energy is C–Cl > C–Br > C–I (Lin et al., 2012; Shang et al., 2014).
TABLE 3. Suzuki coupling reactions of various aryl halide with phenylboronic acid using Pd0@UIO-66–SB–Im as the catalyst.
In order to investigate the heterogeneous nature of Pd0@UIO-66–SB–Im, a hot filtration test was carried out and is shown in Figure 7A. A reaction catalyzed by Pd0@UIO-66–SB–Im was prematurely stopped at 1.5 h (i.e., 51.6% biphenyl yield by GC analysis), and then the solid catalyst was removed by hot filtration. The filtrate was engaged in the additional reaction for an additional 4.5 h, and no more biphenyl was produced as analyzed by GC, confirming that the reaction proceeds on the solid surface of the catalyst and excludes the contribution of the possible leached Pd species. By simple filtration, the catalyst can be facilely separated and reused in the next time. As shown in Figure 7B, the catalyst Pd0@UIO-66–SB–Im could be reused for six times without significant decrease in performance, while the recycled catalyst Pd0@UIO-66–NH2 performed obvious decrease, further implying the well stability of Pd0 species anchored on the Schiff base–imidazole-functionalized UIO-66–SB–Im. As demonstrated by the XRD and FTIR spectra of the spent catalyst Pd0@UIO-66–SB–Im after six runs, the crystalline structure and the polymeric network were preserved (Supplementary Figures S1 and S2). TEM images show that the Pd0 clusters of Pd0@UIO-66-SB-Im6th have grown, which may lead to the slight decrease in catalytic performance (Supplementary Figure S3).
FIGURE 7. (A) Hot filtration test of Pd0@UIO-66–SB–Im catalyzed Suzuki coupling reaction. (B) Reusability of Pd catalysts in the catalytic Suzuki coupling reaction. (Pd0@UIO-66–SB–Im-catalyzed reaction conditions: Pd0@UIO-66–SB–Im (0.06 mol% based on bromobenzene), bromobenzene (2 mmol), phenylboronic acid (3 mmol), NaHCO3 (6 mmol), DMA (5 ml), 6 h, 90°C. Pd0@UIO-66–NH2 catalyzed reaction conditions: Pd0@UIO-66–NH2 (0.09 mol% based on bromobenzene), bromobenzene (2 mmol), phenylboronic acid (3 mmol), NaHCO3 (8 mmol), DMA (5 ml), 8 h, 120°C).
A reaction route of the Suzuki coupling catalyzed by Pd0@UIO-66–SB–Im is proposed by combining the catalytic results and previous mechanism studies (Lin et al., 2012; Shang et al., 2014). As shown in Scheme 2, in the first step, Pd0 clusters stabilized by the Schiff base and imidazole groups on the surface of UIO-66–SB–Im interact with the electrophilic reagent bromobenzene to form organo-palladium species (I) by oxidative addition. Then the organo-palladium species (I) reacts with the other reactant phenylboronic acid through transmetalation in the presence of a base to produce an intermediate (II). Finally, biphenyl is produced, and Pd(II) is reduced to the original Pd0 state through the reductive elimination of (II).
Highly dispersed Pd0 clusters anchored on MOFs was successfully synthesized by postsynthetic modification. The surface groups of Schiff base and imidazole groups that grafted on the MOFs are found to play a vital role in the control of small Pd clusters with an average size of only 1.5 nm. The catalyst Pd0@UIO-66–SB–Im exhibited excellent performance in the Suzuki coupling reaction of halogenated benzene with phenylboronic acid. Moreover, the catalyst behaved well with substrate compatibility and good reusability.
All datasets generated for this study are included in the article/Supplementary Material.
YL and JS contributed to the nanomaterials’ entire design and synthesis. LF and QX guided the idea, analyzed the data, and drafted the manuscript. All authors read and improved the manuscript. All authors read and approved the final manuscript.
The authors thank the National Natural Science Foundation of China (21802119), National Key Research and Development Program (2016YFC0209203), the Joint Open Fund of Jiangsu Collaborative Innovation Center for Ecological Building Material and Environmental Protection Equipment, and Key Laboratory for Advanced Technology in Environmental Protection of Jiangsu Province (JH201806), and Funding for school-level research projects of Yancheng Institute of Technology (xjr2019005).
LF was employed by Yancheng Lanfeng Environmental Engineering Technology Co., Ltd.
The remaining authors declare that the research was conducted in the absence of any commercial or financial relationships that could be construed as a potential conflict of interest.
All claims expressed in this article are solely those of the authors and do not necessarily represent those of their affiliated organizations, or those of the publisher, the editors, and the reviewers. Any product that may be evaluated in this article, or claim that may be made by its manufacturer, is not guaranteed nor endorsed by the publisher.
The Supplementary Material for this article can be found online at: https://www.frontiersin.org/articles/10.3389/fchem.2022.845274/full#supplementary-material
Chen, M. J., Chang, G. G., Chen, L. Y., Huang, K. X., Pu, C., Li, D., et al. (2021). Multifunctional Pd/MOFs@MOFs Confined Core‐Shell Catalysts with Wrinkled Surface for Selective Catalysis. Chem. Asian J. 16, 3743–3747. doi:10.1002/asia.202100922
Chen, X., Shen, K., Ding, D., Chen, J. Y., Chen, J., Wu, R. F., et al. (2018). Solvent-Driven Selectivity Control to Either Anilines or Dicyclohexylamines in Hydrogenation of Nitroarenes over a Bifunctional Pd/MIL-101 Catalyst. ACS Catal. 8, 10641–10648. doi:10.1021/acscatal.8b01834
Díez, I., and Ras, R. H. A. (2011). Fluorescent Silver Nanoclusters. Nanoscale 3, 1963–1970. doi:10.1039/C1NR00006C
Dong, Y., Ying, J., Xiao, Y. X., Chen, J. B., and Yang, X. Y. (2021). Highly Dispersed Pt Nanoparticles Embedded in N‐Doped Porous Carbon for Efficient Hydrogen Evolution. Chem. Asian J. 16, 1878–1881. doi:10.1002/asia.202100438
Eid, K., Sliem, M. H., Eldesoky, A. S., Al-Kandari, H., and Abdullah, A. M. (2019). Rational Synthesis of One-Dimensional Carbon Nitride-Based Nanofibers Atomically Doped with Au/Pd for Efficient Carbon Monoxide Oxidation. Int. J. Hydrogen Energ. 44, 17943–17953. doi:10.1016/j.ijhydene.2019.05.105
Fang, X. Z., Shang, Q. C., Wang, Y., Jiao, L., Yao, T., Li, Y. F., et al. (2018). Single Pt Atoms Confined Into a Metal-Organic Framework for Efficient Photocatalysis. Adv. Mater. 30, 1705112. doi:10.1002/adma.201705112
Furukawa, H., Cordova, K. E., O’Keeffe, M., and Yaghi, O. M. (2013). The Chemistry and Applications of Metal-Organic Frameworks. Science 341, 6149. doi:10.1126/science.1230444
Gu, Y., Chen, X., Cao, Y., Zhuang, G., Zhong, X., and Wang, J. (2017). Atomically Dispersed Pd Catalysts in Graphyne Nanopore: Formation and Reactivity. Nanotechnology 28, 295403. doi:10.1088/1361-6528/aa7764
Guo, Z., You, Q., Song, L., Sun, G., Chen, G., Li, C., et al. (2021). Highly Dispersed Pt Species Anchored onto NH2-Ce-MOFs and Their Derived Mesoporous Catalysts for CO Oxidation. Nanoscale 13, 117–123. doi:10.1039/D0NR05626J
Han, Y., Xu, H., Su, Y., Xu, Z.-l., Wang, K., and Wang, W. (2019). Noble Metal (Pt, Au@Pd) Nanoparticles Supported on Metal Organic Framework (MOF-74) Nanoshuttles as High-Selectivity CO2 Conversion Catalysts. J. Catal. 370, 70–78. doi:10.1016/j.jcat.2018.12.005
He, T., Chen, S., Ni, B., Gong, Y., Wu, Z., Song, L., et al. (2018). Zirconium-porphyrin-based Metal-Organic Framework Hollow Nanotubes for Immobilization of Noble-metal Single Atoms. Angew. Chem. Int. Ed. 57, 3493–3498. doi:10.1002/anie.201800817
Imaoka, T., Akanuma, Y., Haruta, N., Tsuchiya, S., Ishihara, K., Okayasu, T., et al. (2017). Platinum Clusters with Precise Numbers of Atoms for Preparative-Scale Catalysis. Nat. Commun. 8, 688. doi:10.1038/s41467-017-00800-4
Khan, F. U., Mehmood, S., Liu, S., Xu, W., Shah, M. N., Zhao, X., et al. (2021). A P-N Heterojunction Based Pd/PdO@ZnO Organic Frameworks for High-Sensitivity Room-Temperature Formaldehyde Gas Sensor. Front. Chem. 9, 742488. doi:10.3389/fchem.2021.742488
Lee, J., Farha, O. K., Roberts, J., Scheidt, K. A., Nguyen, S. T., and Hupp, J. T. (2009). Metal-organic Framework Materials as Catalysts. Chem. Soc. Rev. 38, 1450–1459. doi:10.1039/B807080F
Li, J.-R., Sculley, J., and Zhou, H.-C. (2012). Metal-organic Frameworks for Separations. Chem. Rev. 112, 869–932. doi:10.1021/cr200190s
Lin, Y., Kong, C., and Chen, L. (2012). Direct Synthesis of Amine-Functionalized MIL-101(Cr) Nanoparticles and Application for CO2 Capture. RSC Adv. 2, 6417–6419. doi:10.1039/c2ra20641b
Liu, J., Ma, Q., Huang, Z., Liu, G., and Zhang, H. (2019). Recent Progress in Graphene-Based noble-metal Nanocomposites for Electrocatalytic Applications. Adv. Mater. 31, 1800696. doi:10.1002/adma.201800696
Liu, Y., Wang, K., Hou, W., Shan, W., Li, J., Zhou, Y., et al. (2018). Mesoporous Poly(ionic Liquid) Supported Palladium(II) Catalyst for Oxidative Coupling of Benzene Under Atmospheric Oxygen. Appl. Surf. Sci. 427, 575–583. doi:10.1016/j.apsusc.2017.08.019
Liu, Y., Zhou, Y., Li, J., Wang, Q., Qin, Q., Zhang, W., et al. (2017). Direct Aerobic Oxidative Homocoupling of Benzene to Biphenyl Over Functional Porous Organic Polymer Supported Atomically Dispersed Palladium Catalyst. Appl. Catal. B: Environ. 209, 679–688. doi:10.1016/j.apcatb.2017.03.029
Liu, Y., Zou, C., Wang, K., Bian, Z., Jiang, S., Zhou, Y., et al. (2021). Palladium Clusters on Dicarboxyl-Functional Hypercrosslinked Porous Polymers for Oxidative Homocoupling of Benzene with O2. Mol. Catal. 505, 111487. doi:10.1016/j.mcat.2021.111487
Mouarrawis, V., Plessius, R., van der Vlugt, J. I., and Reek, J. N. H. (2018). Confinement Effects in Catalysis Using Well-Defined Materials and Cages. Front. Chem. 6, 623. doi:10.3389/fchem.2018.00623
Peng, H., Dong, T., Zhang, L., Wang, C., Liu, W., Bao, J., et al. (2019). Active and Stable Pt-Ceria Nanowires@silica Shell Catalyst: Design, Formation Mechanism and Total Oxidation of CO and Toluene. Appl. Catal. B: Environ. 256, 117807. doi:10.1016/j.apcatb.2019.117807
Shang, N., Gao, S., Zhou, X., Feng, C., Wang, Z., and Wang, C. (2014). Palladium Nanoparticles Encapsulated inside the Pores of a Metal-Organic Framework as a Highly Active Catalyst for Carbon-Carbon Cross-Coupling. RSC Adv. 4, 54487–54493. doi:10.1039/c4ra10065d
Si, J., Xiao, S., Wang, Y., Zhu, L., Xia, X., Huang, Z., et al. (2018). Sub-nanometer Co3O4 Clusters Anchored on TiO2(B) Nano-Sheets: Pt Replaceable Co-catalysts for H2 Evolution. Nanoscale 10, 2596–2602. doi:10.1039/C7NR07336D
Su, J., Yuan, S., Wang, T., Lollar, C. T., Zuo, J.-L., Zhang, J., et al. (2020). Zirconium Metal-Organic Frameworks Incorporating Tetrathiafulvalene Linkers: Robust and Redox-Active Matrices for In Situ Confinement of Metal Nanoparticles. Chem. Sci. 11, 1918–1925. doi:10.1039/C9SC06009J
Tang, Q., Lee, Y., Li, D.-Y., Choi, W., Liu, C. W., Lee, D., et al. (2017). Lattice-Hydride Mechanism in Electrocatalytic CO2 Reduction by Structurally Precise Copper-Hydride Nanoclusters. J. Am. Chem. Soc. 139, 9728–9736. doi:10.1021/jacs.7b05591
Wang, J., Yang, M., Dong, W., Jin, Z., Tang, J., Fan, S., et al. (2016). Co(ii) Complexes Loaded into Metal-Organic Frameworks as Efficient Heterogeneous Catalysts for Aerobic Epoxidation of Olefins. Catal. Sci. Technol. 6, 161–168. doi:10.1039/c5cy01099c
Wang, X., Li, J., Chen, G., Guo, Z., Zhou, Y., and Wang, J. (2015). Hydrophobic Mesoporous Poly(ionic Liquid)s towards Highly Efficient and Contamination-Resistant Solid-Base Catalysts. ChemCatChem 7, 993–1003. doi:10.1002/cctc.201402995
Wang, Y., Su, Y.-Q., Hensen, E. J. M., and Vlachos, D. G. (2020). Finite-temperature Structures of Supported Subnanometer Catalysts Inferred via Statistical Learning and Genetic Algorithm-Based Optimization. ACS Nano 14, 13995–14007. doi:10.1021/acsnano.0c06472
Xin, P., Li, J., Xiong, Y., Wu, X., Dong, J., Chen, W., et al. (2018). Revealing the Active Species for Aerobic Alcohol Oxidation by Using Uniform Supported Palladium Catalysts. Angew. Chem. Int. Ed. 57, 4642–4646. doi:10.1002/anie.201801103
Xiong, G., Chen, X.-L., You, L.-X., Ren, B.-Y., Ding, F., Dragutan, I., et al. (2018). La-Metal-Organic Framework Incorporating Fe3O4 Nanoparticles, post-synthetically Modified with Schiff Base and Pd. A Highly Active, Magnetically Recoverable, Recyclable Catalyst for C C Cross-Couplings at Low Pd Loadings. J. Catal. 361, 116–125. doi:10.1016/j.jcat.2018.02.026
Yamamoto, K., Imaoka, T., Tanabe, M., and Kambe, T. (2020). New Horizon of Nanoparticle and Cluster Catalysis with Dendrimers. Chem. Rev. 120 (2), 1397–1437. doi:10.1021/acs.chemrev.9b00188
Yang, C., Zhao, Z., and Liu, Q. (2021). Mechanistic Insight into the Dispersion Behavior of Single Platinum Atom on Monolayer G-C3n4 in Single-Atom Catalysts from Density Functional Theory Calculations. Appl. Surf. Sci. 566, 150697. doi:10.1016/j.apsusc.2021.150697
Zhang, F., Zheng, S., Xiao, Q., Zhong, Y., Zhu, W., Lin, A., et al. (2016). Synergetic Catalysis of Palladium Nanoparticles Encaged within Amine-Functionalized UiO-66 in the Hydrodeoxygenation of Vanillin in Water. Green. Chem. 18, 2900–2908. doi:10.1039/c5gc02615f
Zhang, X., Zhang, X., Yang, P., and Jiang, S. P. (2021). Pt Clusters Embedded in G-C3n4 Nanosheets to Form Z-Scheme Heterostructures with Enhanced Photochemical Performance. Surf. Inter. 27, 101450. doi:10.1016/j.surfin.2021.101450
Keywords: Pd clusters, subnanometer size, Schiff base, Suzuki coupling reaction, metal organic frameworks
Citation: Liu Y, Sun J, Fan L and Xu Q (2022) Pd Clusters on Schiff Base–Imidazole-Functionalized MOFs for Highly Efficient Catalytic Suzuki Coupling Reactions. Front. Chem. 10:845274. doi: 10.3389/fchem.2022.845274
Received: 29 December 2021; Accepted: 20 January 2022;
Published: 01 March 2022.
Edited by:
Xiao-Yu Yang, Wuhan University of Technology, ChinaReviewed by:
Hexing Li, Shanghai Normal University, ChinaCopyright © 2022 Liu, Sun, Fan and Xu. This is an open-access article distributed under the terms of the Creative Commons Attribution License (CC BY). The use, distribution or reproduction in other forums is permitted, provided the original author(s) and the copyright owner(s) are credited and that the original publication in this journal is cited, in accordance with accepted academic practice. No use, distribution or reproduction is permitted which does not comply with these terms.
*Correspondence: Qi Xu, eWN4cXN0ZXZlQDE2My5jb20=
†These authors have contributed equally to this work
Disclaimer: All claims expressed in this article are solely those of the authors and do not necessarily represent those of their affiliated organizations, or those of the publisher, the editors and the reviewers. Any product that may be evaluated in this article or claim that may be made by its manufacturer is not guaranteed or endorsed by the publisher.
Research integrity at Frontiers
Learn more about the work of our research integrity team to safeguard the quality of each article we publish.