- 1Institute of Chemistry, Leiden University, Leiden, Netherlands
- 2National Research Centre for Carbohydrate Synthesis, Jiangxi Normal University, Nanchang, China
Pseudomonas aeruginosa, a pathogenic Gram-negative bacterium for which currently antibiotic resistance is posing a significant problem and for which no vaccines are available, protects itself by the formation of a biofilm. The Pel polysaccharide, a cationic polymer composed of cis-linked galactosamine (GalN), N-acetyl galactosamine (GalNAc), glucosamine (GlcN) and N-acetyl glucosamine (GlcNAc) monosaccharides, is an important constituent of the biofilm. Well-defined Pel oligosaccharides will be valuable tools to probe the biosynthesis machinery of this polysaccharide and may serve as diagnostic tools or be used as components of glycoconjugate vaccines. We here, report on the development of synthetic chemistry to access well-defined Pel-oligosaccharides. The chemistry hinges on the use of di-tert-butylsilylidene protected GalN and GlcN building blocks, which allow for completely cis-selective glycosylation reactions. We show the applicability of the chemistry by the assembly of a matrix of 3 × 6 Pel heptasaccharides, which has been generated from a single set of suitably protected Pel heptasaccharides, in which a single glucosamine residue is incorporated and positioned at different places along the Pel oligo-galactosamine chain.
Introduction
Pseudomonas aeruginosa is an opportunistic Gram-negative pathogen that can cause both acute and chronic infections in immunocompromised patients (Franklin et al., 2011; Ghafoor et al., 2011; Ma et al., 2012; Jennings et al., 2015; Marmont et al., 2017; Whitfield et al., 2020). P. aeruginosa can become resistant to antibiotics due to its ability to form a biofilm which complicates the treatment of its infections. As part of the biofilm three exopolysaccharides are synthesized, alginate, Pel and Psl (Franklin et al., 2011). Alginate is a negatively charged polymer of mannuronic and guluronic acid (Flo et al., 2002), while Psl is a neutral polysaccharide composed of a pentasaccharide repeat containing glucose, rhamnose and mannose (Jackson et al., 2004). Pel is a positively charged polymer, and although its structure has not been fully characterized it is thought to be composed of α-1,4-linked N-acetylgalactosamine (GalNAc) and N-acetyl-glucosamine (GlcNAc), both of which can be de-acetylated to give galactosamine (GalN) and glucosamine (GlcN) residues, respectively (Figure 1A). The GalN(Ac): GlcN(Ac) ratio has been reported to be ±6:1 (Jennings et al., 2015). Pel plays an important role in maintaining cell-cell interactions in biofilms and affords protection to the bacterium by enhancing resistance to aminoglycoside antibiotics (Colvin et al., 2011). Well-defined fragments of the Pel polymer can serve as powerful research tools in various interconnected fields of research. They may serve as synthetic antigens in the generation of potential pseudomonas vaccines and they can be used in elucidating biosynthesis pathways and characterizing the enzymes involved therein. This may open up avenues to interfere with the biosynthesis and eventually generate anti-bacterial compounds. Because of the seemingly random distribution of monosaccharides in Pel, it is impossible to isolate well defined fragments from natural sources and therefore organic synthesis is the method of choice to provide these.
The key to the assembly of Pel fragments is the stereoselective introduction of α-GalN, α-GalNAc, α-GlcN and α-GlcNAc linkages. We have previously described the successful application of the 4,6-O-di-tert-butylsilylidene (DTBS) directed α-galactosylation methodology, developed by Kiso’s group (Imamura et al., 2003; Imamura et al., 2005; Sato et al., 2009; Imamura et al., 2016; Kazakova et al., 2020; Wang et al., 2020), for the synthesis of the structurally related galactosaminogalactan (GAG) homo- and hetero-oligosaccharides that occur in the cell wall of Aspergillus fumigatus and that are composed of 1,4-linked α-Gal, α-GalN, α-GalNAc residues (Bamford et al., 2019; Le Mauff et al., 2019; Bamford et al., 2020; Zhang et al., 2020a). The application of 4,6-O-DTBS protected GalN3 and GalNHTCA donors resulted in glycosylations with high α-stereoselectivity to give a row of GAG fragments, having both GalN and GalNAc constituents. The high α-stereoselectivity of the glycosylations proved to be insensitive to the nature of the C-2-N-acyl group, capable of neighboring group participation. On the basis of these results, we selected DTBS-protected GalN donors as building blocks for the construction of α-GalN and α-GalNAc linkages in Pel. The formation of similar α-GlcN linkages is more challenging and substantial effort has been expended to develop a procedure for the stereoselective introduction of α-GlcN linkages (Benakli et al., 2001; Wei and Kerns, 2005; Manabe et al., 2006; Park et al., 2007; Geng et al., 2008; Olsson et al., 2008; Mensah et al., 2010; Ye and Geng, 2010; Ingle et al., 2013; van der Vorm et al., 2017; Zhang et al., 2020). Recently, we have reported on an effective synthetic strategy to assemble a Pel-type oligosaccharide, containing 1,4-linked GalNAc and GlcNAc residues (Wang et al., 2020). A [2 + 2 + 2] strategy was developed for the synthesis of a hexasaccharide in which the glucosamine linkages were constructed using a N-methyl-N-phenylformamide (MPF)-modulated glycosylation methodology. We have previously also systematically evaluated a set of glycosylation reactions between a series of 4,6-tethered glucosazide donors and a panel of acceptors to find that with increasing reactivity of the studied GlcN3-donors and decreasing nucleophilicity of the acceptors, the α-selectivity of the glycosylations increased (van der Vorm et al., 2017). The most reactive GlcN3 donor that was studied carried a 4,6-DTBS group and its reaction with the model nucleophile trifluoroethanol (TFE) gave the α-linked product exclusively. As the nucleophilicity of the C-4-OH in GalN moieties is relatively low, the DTBS-GlcN3 donors may represent promising building blocks for the construction of α-GlcN-(1→4)-GalN linkages.
We here describe the synthesis of a library of Pel fragments with DTBS-directed glycosylation methodology. A library of hetero-oligomers containing α-GalN/α-GalNAc and α-GlcN/α-GlcNAc residues at predetermined positions, was designed (Figure 1B). A set of heptamers, each of which contains one GlcN/GlcNAc-residue and six GalN/GalNAc residues, was selected because of the GalNAc:GlcNAc ratio that is present in naturally occurring Pel polysaccharides, while some of the residues have been deacetylated (Jennings et al., 2015). Also a spacer was incorporated at the reducing end of the heptamers for future conjugation purposes.
Results and Discussion
As the DTBS-directed α-galactosylation methodology is well established, attention was first paid to the formation of α-GlcN3-(1→4)-GalN3 linkages. A set of glycosylation reactions was investigated using GlcN3 donors 1–4 (van der Vorm et al., 2017; Revuelta et al., 2018; Wang et al., 2018) and GalN3 acceptors 5–7 (Wang et al., 2020; Zhang et al., 2020a) (Table 1). First, the additive N-methyl-N-phenylformamide (MPF) controlled α-glycosylation methodology was attempted to introduce the α-GlcN linkage. With this methodology, glycosylation of benzylated GlcN3 donor 1 with benzylated GalN3 acceptor 7 led to the disaccharide 8 with 7:1 α/β-selectivity, but the yield was only 47% (Table 1, entry 1). Using the same conditions, coupling of 4,6-DTBS-protected GlcN3 donor 4 with GalN3 7 afforded the dimer in a mere 5% yield (entry 2), owing to the low reactivity of the GalN3 C4-OH acceptor. Next, a pre-activation strategy, using GlcN3 donors 2 and 3 as donors was explored. Benzylidene-protected donor 2 reacted with acceptor 5, to afford disaccharide 9 in 38% yield and with a 3.5/1 α/β ratio (entry 3). When the more reactive DTBS-protected donor 3 was treated with 5, a slightly better α-selectivity was obtained (α/β = 5/1, entry 4). Condensation of donor 3 with 6-O-Bn protected acceptor 6 led to 11 (Wang et al., 2020) in excellent yield and α-selectivity (entry 5). By contrast, changing the linker of the acceptor to 3-buten-ol, which is more convenient for future conjugation purposes, gave no glycosylation product (entry 6). Condensation of GlcN3 donor 3 and acceptor 7, promoted by NIS and TfOH at −40°C, also failed to afford the product (entry 7). To further improve the reaction, imidate donor 4 was coupled with acceptor 5, under influence of TBSOTf, giving dimer 10 with moderate α-selectivity (α/β = 3.7/1, entry 8). Gratifyingly, performing the glycosylation of donor 4 and acceptor 7, at −10°C with TfOH as promotor, furnished the desired disaccharide 12 in 77% yield with excellent α-selectivity (>20:1, entry 9). Based on these model reactions, the DTBS-tethered GlcN3 donor 4 was chosen for the construction of α-GlcN3-(1→4)-GalN3 linkages, and the benzyl group was preferred for the protection of C6-OH of the GalN acceptors. Notably, the implementation of this strategy would match exceptionally well with the strategy we previously developed for the introduction of α-GalN and α-GalNAc linkages in the synthesis of the related GAG oligosaccharides.
With conditions in hand to construct the required α-GalN and α-GlcN linkages, attention was directed to the assembly of a library of Pel heptamers, consisting of (3 × 6) members, that can be achieved by the synthesis of six protected heptameric precursors and subjecting these to different deprotection procedures. The projected eighteen heptamers contain one GlcN or GlcNAc, differently positioned in the heptameric chain, while the remaining residues are all GalN, all GalNAc or alternating GalN and GalNAc (Figure 1B). The retrosynthesis of Pel heptamers A–C with either GlcN or GlcNAc at the second position from the reducing end of the heptamer is depicted in Figure 2. This retrosynthesis also applies to the remaining members of the projected library that can be accessed using the same strategy. The deprotected heptamers A–C are derived from protected heptamer D through different procedures for the removal of the protecting groups. In path a, the sequence of deprotection steps include DTBS removal, reduction of azido groups, and removal of Bn and TFA groups via Birch reduction to afford compound A, containing GalN and GlcN residues. Birch reduction is chosen to avoid reduction of C-C double bond in the linker (Zhang et al., 2019). Acetylation of free amine groups in A can furnish heptamer B. In path b, the C2-N-TFA groups are first removed, followed by desilylation and acetylation of the released amino groups, after which reduction the azido and Bn-groups should give the heptamer C. The common protected heptamer D can be constructed with GlcN3 donor 4, GalN3 donor 13 and GalNHTFA donor 14, which would serve as precursors for the GlcN, GlcNAc, GalN and GalNAc residues.
Table 2 summarizes the syntheses of the six fully protected Pel heptasaccharides (20, 26, 31, 35, 38, 40) with one GlcN3 residue at different positions. The elongation cycle consisted of the following three-steps: 1) Glycosylation using the donor of choice, 2) DTBS-removal with HF/pyridine and 3) selective benzylation of the primary alcohol group. The Bn group can be regioselectively introduced under the aegis of Taylor’s borinic acid catalyst (Chan and Taylor, 2011; Lee and Taylor, 2011; Lee et al., 2012). As can be seen from the Table, the heptasaccharide 20 (or D in Figure 2) with the GlcN3 moiety at the second position from the reducing end of the heptamer was first synthesized. Condensation of GlcN3 donor 4 and acceptor 7 led to the disaccharide 12 using a TfOH promoted condensation at −10°C (Table 1). Next the DTBS group was cleaved and the liberated 6-OH was benzylated selectively to form the desired 4-OH acceptor, which was reacted with GalNHTFA donor 14 giving the trisaccharide 16 in 73% yield over the three steps. However, the relatively moderate yield of the glycosylation for the tetra- and pentamer (56% for 17 and 51% for 18) was an incentive to optimize the glycosylation reaction conditions. It was found that implementation of a “reverse addition sequence” strategy, in which the acceptor and activator are mixed, after which the donor is slowly added, greatly improved the reaction yields (71% for 17 and 72% for 18). Elongation of the pentamer with another copy of the GalN3 donor 13 and subsequently the GalNHTFA building block 14, delivered heptasaccharide 20 in excellent yields. It should be noted that the desilylation reactions and regioselective benzylations all occurred with excellent chemo- and regioselectivity, showing the effectivity of these reactions to be independent on glycan length.
In an analogous way, the assembly of target heptasaccharides 26, 31, 35, 38, and 40 with a GlcN3 moiety at the positions 3-7 was accomplished with building blocks 4, 13 and 14. Repetition of the elongation cycle, comprising the same three steps as described above led to all target heptasaccharides. The glycosylation reactions proceeded efficiently providing the intermediate and target oligosaccharides (n = 2–7) with excellent stereoselectivity and good yields (50–79% yields for three steps). The mixed sequence structures were generated uneventfully, showing the chemistry developed to be applicable to any type of Pel-target.
With all six protected heptasaccharides in hand, deprotection conditions were explored to complete the assembly of all projected Pel oligomers (Scheme 1). First, the set of 7-mers containing solely α-GalN and α-GlcN moieties was generated. Removal of the DTBS-group in heptamers 20, 26, 31, 35, 38 and 40 was performed with HF/pyridine and the azido-groups could be reduced with HS(CH2)3SH, after which the Bn groups together with the TFA groups were cleaved using sodium in ammonia and THF, affording the 7-mers 41–46 in 48–85% yields. In the Birch reduction, allyl carbinol was used as a scavenger to prevent reduction of the linker alkene. A portion of the 7-mers 41–46 was chemoselectively acetylated to provide the second set of heptamers 47–52, composed of α-GalNAc and α-GlcNAc moieties. Furthermore, the heptamers 20, 26, 31, 35, 38 and 40 were transformed into the third set of GalN-, GalNAc and GlcN-containing heptamers 53–58. Similar to the first series, the silylidene groups were first removed. However, we then found that the TFA groups could not be cleaved even with strong basic/nucleophilic conditions and high temperatures (4M NaOH, 80°C). Also attempts to remove the TFA groups with the assistance of microwave failed (see experimental section, Supplementary Table S1). A solution for this problem was found by first removing the benzyl ethers and concomitant reduction of the azido groups using hydrogenation over Pd(OH)2/C, followed by the temporary protection of the generated free amino groups with a Boc group. At this stage, the TFA groups could be removed with NH3.H2O at 60°C, after which acetylation of generated amines and subsequent removal of the Boc groups with 30% TFA provided the heptamers 53–58 in 18–31% yields. Although this sequence of reactions sacrificed the alkene group in the linker moiety it did grant access to the last series of Pel-oligosaccharides.
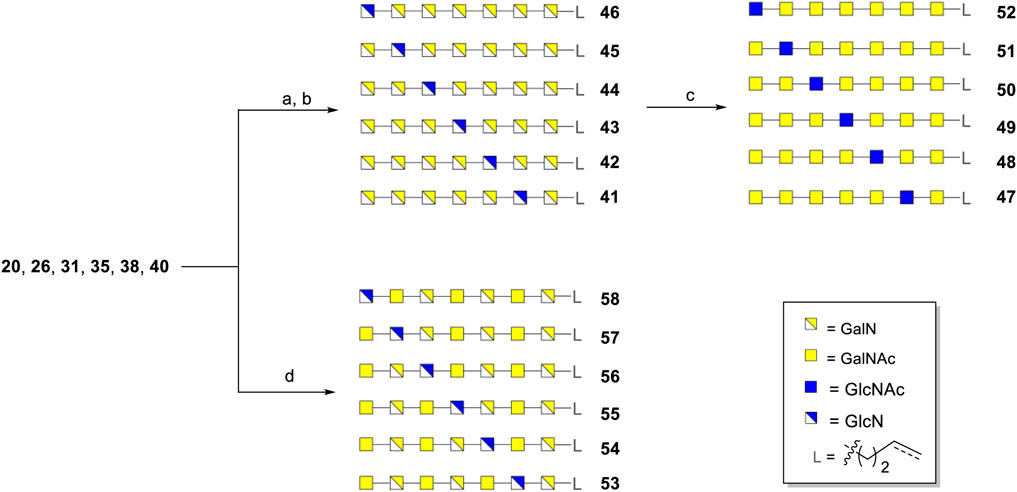
SCHEME 1. Deprotection of synthetic Pel heptasaccharides. a) i) HF/pyridine, THF, rt; ii) HS(CH2)3SH, Et3N, pyridine/H2O, rt. b) Na, NH3 (liq.), THF, t-BuOH, 3-buten-1-ol, −78°C, yields for 41: 69% (12/1 with:without C=C); 42: 48% (23/1); 43: 84% (19/1); 44: 53% (50/1); 45: 59% (25/1); 46: 85% (43/1). c) Ac2O, H2O, NaHCO3, rt, yields for 47: 90%; 48: 91% (11/1); 49: 91% (32/1); 50: 90% (32/1); 51: 89% (21/1); 52: 88% (12/1). d) i) HF/pyridine, THF, rt; ii) Pd(OH)2/C, H2, AcOH, THF/t-BuOH/H2O, rt; iii) Boc2O, NaHCO3, H2O, rt; iv) NH3.H2O, 60°C; v) Ac2O, NaHCO3, H2O, rt; vi) 30% TFA in H2O, L = (CH2)3CH3, yields for 53: 31%; 54: 25%; 55: 24%; 56: 18%; 57: 30%; 58: 18%.
Conclusion
In conclusion, synthetic methodology enabling the assembly of Pel fragments has been developed. Key features of the synthetic strategy include the use of DTBS-directed α-glycosylation methodology and a regioselective benzylation procedure. The DTBS-directed glycosylation was not only successfully applied for the construction of α-GalN3 and α-GalNTFA linkages, as already previously described, it also proved applicable for the synthesis of α-GlcN3 linkages. With the increasing length of the oligosaccharides, the glycosylation yields decreased significantly, owing to the reduced nucleophilicity of the acceptors. Application of a reverse-addition-sequence strategy adequately improved the yields of the glycosylations providing the longer oligosaccharides in good yield. Six protected heptamers with different composition were subjected to different deprotection protocols, providing three sets of heptamers containing α-GlcN-α-GalN, α-GlcNAc-α-GalNAc and α-GlcN-α-GalNAc-α-GalN combinations. Unexpectedly, it proved impossible to remove the N-TFA groups in the heptamers carrying benzyl protecting groups. Fortunately, a protocol in which the benzyl and azide groups were first reduced, after which the liberated amines were temporarily masked as Boc-carbamates, allowed for removal of the TFA groups, using aqueous ammonia hydroxide. The synthetic Pel heptamers will be valuable for the define establishment of the Pel structure, studies of the biosynthesis machinery and biofilm forming process as well as the development of vaccines and diagnostic tools to combat and monitor P. aeruginosa infections.
Data Availability Statement
The original contributions presented in the study are included in the article/Supplementary Material, further inquiries can be directed to the corresponding author.
Author Contributions
YZ and LW performed the experimental work. JC, GM, HO, LW, and YZ arranged and wrote the manuscript.
Funding
This work was supported by the European Research Council (ERC-CoG-726072-“GLYCONTROL”, to J.D.C.C.).
Conflict of Interest
The authors declare that the research was conducted in the absence of any commercial or financial relationships that could be construed as a potential conflict of interest.
Publisher’s Note
All claims expressed in this article are solely those of the authors and do not necessarily represent those of their affiliated organizations, or those of the publisher, the editors and the reviewers. Any product that may be evaluated in this article, or claim that may be made by its manufacturer, is not guaranteed or endorsed by the publisher.
Supplementary Material
The Supplementary Material for this article can be found online at: https://www.frontiersin.org/articles/10.3389/fchem.2022.842238/full#supplementary-material
References
Bamford, N. C., Le Mauff, F., Subramanian, A. S., Yip, P., Millán, C., Zhang, Y., et al. (2019). Ega3 from the Fungal Pathogen Aspergillus fumigatus Is an Endo-α-1,4-Galactosaminidase that Disrupts Microbial Biofilms. J. Biol. Chem. 294 (37), 13833–13849. doi:10.1074/jbc.RA119.009910
Bamford, N. C., Le Mauff, F., Van Loon, J. C., Ostapska, H., Snarr, B. D., Zhang, Y., et al. (2020). Structural and Biochemical Characterization of the Exopolysaccharide Deacetylase Agd3 Required for Aspergillus fumigatus Biofilm Formation. Nat. Commun. 11 (1), 2450. doi:10.1038/s41467-020-16144-5
Benakli, K., Zha, C., and Kerns, R. J. (2001). Oxazolidinone Protected 2-Amino-2-Deoxy-D-Glucose Derivatives as Versatile Intermediates in Stereoselective Oligosaccharide Synthesis and the Formation of α-Linked Glycosides. J. Am. Chem. Soc. 123 (38), 9461–9462. doi:10.1021/ja0162109
Chan, L., and Taylor, M. S. (2011). Regioselective Alkylation of Carbohydrate Derivatives Catalyzed by a Diarylborinic Acid Derivative. Org. Lett. 13 (12), 3090–3093. doi:10.1021/ol200990e
Colvin, K. M., Gordon, V. D., Murakami, K., Borlee, B. R., Wozniak, D. J., Wong, G. C. L., et al. (2011). The Pel Polysaccharide Can Serve a Structural and Protective Role in the Biofilm Matrix of Pseudomonas aeruginosa. Plos. Pathog. 7 (1), e1001264. doi:10.1371/journal.ppat.1001264
Flo, T. H., Ryan, L., Latz, E., Takeuchi, O., Monks, B. G., Lien, E., et al. (2002). Involvement of Toll-like Receptor (TLR) 2 and TLR4 in Cell Activation by Mannuronic Acid Polymers. J. Biol. Chem. 277 (38), 35489–35495. doi:10.1074/jbc.M201366200
Franklin, M. J., Nivens, D. E., Weadge, J. T., and Howell, P. L. (2011). Biosynthesis of the Pseudomonas aeruginosa Extracellular Polysaccharides, Alginate, Pel, and Psl. Front. Microbio. 2, 167. doi:10.3389/fmicb.2011.00167
Geng, Y., Zhang, L.-H., and Ye, X.-S. (2008). Pre-activation Protocol Leading to Highly Stereoselectivity-Controllable Glycosylations of Oxazolidinone Protected Glucosamines. Chem. Commun. (5), 597–599. doi:10.1039/b712591g
Ghafoor, A., Hay, I. D., and Rehm, B. H. A. (2011). Role of Exopolysaccharides in Pseudomonas aeruginosa Biofilm Formation and Architecture. Appl. Environ. Microbiol. 77 (15), 5238–5246. doi:10.1128/AEM.00637-11
Imamura, A., Ando, H., Ishida, H., and Kiso, M. (2005). Di-tert-butylsilylene-Directed α-Selective Synthesis of 4-Methylumbelliferyl T-Antigen. Org. Lett. 7, 4415–4418. doi:10.1021/ol051592z
Imamura, A., Ando, H., Korogi, S., Tanabe, G., Muraoka, O., Ishida, H., et al. (2003). Di-tert-butylsilylene (DTBS) Group-Directed α-selective Galactosylation Unaffected by C-2 Participating Functionalities. Tetrahedron Lett. 44 (35), 6725–6728. doi:10.1016/s0040-4039(03)01647-2
Imamura, A., Matsuzawa, N., Sakai, S., Udagawa, T., Nakashima, S., Ando, H., et al. (2016). The Origin of High Stereoselectivity in Di-tert-butylsilylene-directed α-Galactosylation. J. Org. Chem. 81 (19), 9086–9104. doi:10.1021/acs.joc.6b01685
Ingle, A. B., Chao, C.-S., Hung, W.-C., and Mong, K.-K. T. (2013). Tuning Reactivity of Glycosyl Imidinium Intermediate for 2-Azido-2-Deoxyglycosyl Donors in α-Glycosidic Bond Formation. Org. Lett. 15 (20), 5290–5293. doi:10.1021/ol402519c
Jackson, K. D., Starkey, M., Kremer, S., Parsek, M. R., and Wozniak, D. J. (2004). Identification of Psl , a Locus Encoding a Potential Exopolysaccharide that Is Essential for Pseudomonas aeruginosa PAO1 Biofilm Formation. J. Bacteriol. 186 (14), 4466–4475. doi:10.1128/JB.186.14.4466-4475.2004
Jennings, L. K., Storek, K. M., Ledvina, H. E., Coulon, C., Marmont, L. S., Sadovskaya, I., et al. (2015). Pel Is a Cationic Exopolysaccharide that Cross-Links Extracellular DNA in thePseudomonas Aeruginosabiofilm Matrix. Proc. Natl. Acad. Sci. USA 112 (36), 11353–11358. doi:10.1073/pnas.1503058112
Kazakova, E. D., Yashunsky, D. V., Krylov, V. B., Bouchara, J.-P., Cornet, M., Valsecchi, I., et al. (2020). Biotinylated Oligo-α-(1 → 4)-D-Galactosamines and Their N-Acetylated Derivatives: α-Stereoselective Synthesis and Immunology Application. J. Am. Chem. Soc. 142 (3), 1175–1179. doi:10.1021/jacs.9b11703
Le Mauff, F., Bamford, N. C., Alnabelseya, N., Zhang, Y., Baker, P., Robinson, H., et al. (2019). Molecular Mechanism of Aspergillus fumigatus Biofilm Disruption by Fungal and Bacterial Glycoside Hydrolases. J. Biol. Chem. 294 (28), 10760–10772. doi:10.1074/jbc.RA119.008511
Lee, D., and Taylor, M. S. (2011). Borinic Acid-Catalyzed Regioselective Acylation of Carbohydrate Derivatives. J. Am. Chem. Soc. 133 (11), 3724–3727. doi:10.1021/ja110332r
Lee, D., Williamson, C. L., Chan, L., and Taylor, M. S. (2012). Regioselective, Borinic Acid-Catalyzed Monoacylation, Sulfonylation and Alkylation of Diols and Carbohydrates: Expansion of Substrate Scope and Mechanistic Studies. J. Am. Chem. Soc. 134 (19), 8260–8267. doi:10.1021/ja302549c
Ma, L., Wang, J., Wang, S., Anderson, E. M., Lam, J. S., Parsek, M. R., et al. (2012). Synthesis of Multiple Pseudomonas aeruginosa Biofilm Matrix Exopolysaccharides Is post-transcriptionally Regulated. Environ. Microbiol. 14 (8), 1995–2005. doi:10.1111/j.1462-2920.2012.02753.x
Manabe, S., Ishii, K., and Ito, Y. (2006). N-Benzyl-2,3-oxazolidinone as a Glycosyl Donor for Selective α-Glycosylation and One-Pot Oligosaccharide Synthesis Involving 1,2-Cis-Glycosylation. J. Am. Chem. Soc. 128 (33), 10666–10667. doi:10.1021/ja062531e
Marmont, L. S., Whitfield, G. B., Rich, J. D., Yip, P., Giesbrecht, L. B., Stremick, C. A., et al. (2017). PelA and PelB Proteins Form a Modification and Secretion Complex Essential for Pel Polysaccharide-dependent Biofilm Formation in Pseudomonas aeruginosa. J. Biol. Chem. 292 (47), 19411–19422. doi:10.1074/jbc.M117.812842
Mensah, E. A., Yu, F., and Nguyen, H. M. (2010). Nickel-Catalyzed Stereoselective Glycosylation with C(2)-N-Substituted Benzylidene D-Glucosamine and Galactosamine Trichloroacetimidates for the Formation of 1,2-Cis-2-Amino Glycosides. Applications to the Synthesis of Heparin Disaccharides, GPI Anchor Pseudodisaccharides, and α-GalNAc. J. Am. Chem. Soc. 132 (40), 14288–14302. doi:10.1021/ja106682m
Olsson, J. D. M., Eriksson, L., Lahmann, M., and Oscarson, S. (2008). Investigations of Glycosylation Reactions with 2-N-Acetyl-2n,3o-Oxazolidinone-Protected Glucosamine Donors. J. Org. Chem. 73 (18), 7181–7188. doi:10.1021/jo800971s
Park, J., Kawatkar, S., Kim, J.-H., and Boons, G.-J. (2007). Stereoselective Glycosylations of 2-Azido-2-Deoxy-Glucosides Using Intermediate Sulfonium Ions. Org. Lett. 9 (10), 1959–1962. doi:10.1021/ol070513b
Revuelta, J., Fuentes, R., Lagartera, L., Hernáiz, M. J., Bastida, A., García-Junceda, E., et al. (2018). Assembly of Glycoamino Acid Building Blocks: a New Strategy for the Straightforward Synthesis of Heparan Sulfate Mimics. Chem. Commun. 54 (95), 13455–13458. doi:10.1039/C8CC08067D
Sato, T., Imamura, A., Ando, H., Ishida, H., and Kiso, M. (2009). Di-tert-butylsilylene-directed α-selective Synthesis of P-Nitrophenyl T-Antigen Analogues. Glycoconj. J. 26 (1), 83–98. doi:10.1007/s10719-008-9168-y
van der Vorm, S., Overkleeft, H. S., van der Marel, G. A., and Codée, J. D. C. (2017). Stereoselectivity of Conformationally Restricted Glucosazide Donors. J. Org. Chem. 82 (9), 4793–4811. doi:10.1021/acs.joc.7b00470
Wang, L., Overkleeft, H. S., van der Marel, G. A., and Codée, J. D. C. (2018). Reagent Controlled Stereoselective Synthesis of α-Glucans. J. Am. Chem. Soc. 140 (13), 4632–4638. doi:10.1021/jacs.8b00669
Wang, L., Zhang, Y., Overkleeft, H. S., van der Marel, G. A., and Codée, J. D. C. (2020). Reagent Controlled Glycosylations for the Assembly of Well-Defined Pel Oligosaccharides. J. Org. Chem. 85 (24), 15872–15884. doi:10.1021/acs.joc.0c00703
Wei, P., and Kerns, R. J. (2005). Factors Affecting Stereocontrol during Glycosidation of 2,3-Oxazolidinone-Protected 1-Tolylthio-N-Acetyl-D-Glucosamine. J. Org. Chem. 70 (10), 4195–4198. doi:10.1021/jo047812o
Whitfield, G. B., Marmont, L. S., Ostaszewski, A., Rich, J. D., Whitney, J. C., Parsek, M. R., et al. (2020). Pel Polysaccharide Biosynthesis Requires an Inner Membrane Complex Comprised of PelD, PelE, PelF, and PelG. J. Bacteriol. 202 (8). doi:10.1128/JB.00684-19
Ye, X.-S., and Geng, Y. (2010). Additive-Controlled Stereoselective Glycosylations of Oxazolidinone-Protected Glucosamine and Galactosamine Thioglycoside Donors Based on Preactivation Protocol. Synlett 2010 (16), 2506–2512. doi:10.1055/s-0030-1258557
Zhang, Q., Gimeno, A., Santana, D., Wang, Z., Valdés-Balbin, Y., Rodríguez-Noda, L. M., et al. (2019). Synthetic, Zwitterionic Sp1 Oligosaccharides Adopt a Helical Structure Crucial for Antibody Interaction. ACS Cent. Sci. 5 (8), 1407–1416. doi:10.1021/acscentsci.9b00454
Zhang, Y., Gómez‐Redondo, M., Jiménez‐Osés, G., Arda, A., Overkleeft, H. S., Marel, G. A., et al. (2020a). Synthesis and Structural Analysis of Aspergillus fumigatus Galactosaminogalactans Featuring α‐Galactose, α‐Galactosamine and α‐ N ‐Acetyl Galactosamine Linkages. Angew. Chem. Int. Ed. 59 (31), 12746–12750. doi:10.1002/anie.202003951
Zhang, Y., Zhang, H., Zhao, Y., Guo, Z., and Gao, J. (2020b). Efficient Strategy for α-Selective Glycosidation of D-Glucosamine and its Application to the Synthesis of a Bacterial Capsular Polysaccharide Repeating Unit Containing Multiple α-Linked GlcNAc Residues. Org. Lett. 22 (4), 1520–1524. doi:10.1021/acs.orglett.0c00101
Keywords: glycosylation, sereoselectivity, bacterial polysaccharides, pseudomonas aeruginosa, biofilm
Citation: Zhang Y, Wang L, Overkleeft HS, van der Marel GA and Codée JDC (2022) Assembly of a Library of Pel-Oligosaccharides Featuring α-Glucosamine and α-Galactosamine Linkages. Front. Chem. 10:842238. doi: 10.3389/fchem.2022.842238
Received: 23 December 2021; Accepted: 05 January 2022;
Published: 26 January 2022.
Edited by:
M. Carmen Galan, University of Bristol, United KingdomReviewed by:
Antonio Molinaro, University of Naples Federico II, ItalyTodd Lowary, University of Alberta, Canada
Copyright © 2022 Zhang, Wang, Overkleeft, van der Marel and Codée. This is an open-access article distributed under the terms of the Creative Commons Attribution License (CC BY). The use, distribution or reproduction in other forums is permitted, provided the original author(s) and the copyright owner(s) are credited and that the original publication in this journal is cited, in accordance with accepted academic practice. No use, distribution or reproduction is permitted which does not comply with these terms.
*Correspondence: Jeroen D. C. Codée, amNvZGVlQGNoZW0ubGVpZGVudW5pdi5ubA==