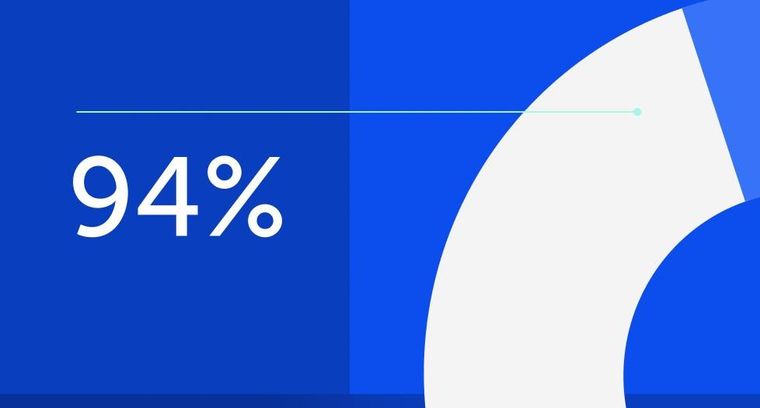
94% of researchers rate our articles as excellent or good
Learn more about the work of our research integrity team to safeguard the quality of each article we publish.
Find out more
ORIGINAL RESEARCH article
Front. Chem., 05 May 2022
Sec. Inorganic Chemistry
Volume 10 - 2022 | https://doi.org/10.3389/fchem.2022.829538
This article is part of the Research TopicWomen in Science: Chemistry 2021View all 14 articles
Metal clusters have gained a lot of interest for their remarkable photoluminescence and catalytic properties. However, a major drawback of such materials is their poor stability in air and humidity conditions. Herein we describe a versatile method to synthesize luminescent Cu(I) clusters inside the pores of zeolites, using a sublimation technique with the help of high vacuum and high temperature. The porous materials play an essential role as a protecting media against the undesirable and easy oxidation of Cu(I). The obtained clusters show fascinating luminescence properties, and their reactivity can be triggered by insertion in the pores of organic monodentate ligands such as pyridine or triphenylphosphine. The coordinating ligands can lead to the formation of Cu(I) complexes with completely different emission properties. In the case of pyridine, the final compound was characterized and identified as a cubane-like structure. A thermochromism effect is also observed, featuring, for instance, a hypsochromic effect for a phosphine derivative at 77K. The stability of the encapsulated systems in zeolites is rather enthralling: they are stable and emissive even after several months in the air.
Currently, rare-earth-based phosphors are frequently used as emitters in lighting applications. (Bünzli and Piguet, 2005; Eliseeva and Bünzli, 2010). They are commercially available materials that are sufficiently photostable upon UV or blue excitation and meet all the industrial requirements regarding emission colors and efficiency. However, their very high cost and limited natural availability led researchers to find valuable alternatives. In this regard, various materials such as quantum dots based on zinc and cadmium selenides, (Raman et al., 1996; Frecker et al., 2016), have been developed with favorable spectral characteristics to replace rare-earth-based phosphors. Unfortunately, these are usually highly toxic and expensive, (Lewinski et al., 2008), and their use is not desirable. A valuable, cheaper, and a more environmentally friendly alternative is the use of metal complexes (Armaroli et al., 2007; Costa et al., 2012; Keller et al., 2018; Weber et al., 2018) and in this respect, silver has resulted in excellent luminophores with outstanding emission quantum yields when the clusters are protected by the environment. (Fenwick et al., 2016; Baekelant et al., 2019; Baekelant et al., 2020).
Amongst the use of cheaper and more abundant metals, copper is an interesting choice and many compounds have been investigated for their outstanding emission properties. (Armaroli et al., 2007; Costa et al., 2012; Keller et al., 2018; Weber et al., 2018). Indeed, M. Thompson et al. recently reported Cu(I) complexes reaching almost quantitative emission quantum yields. (Hamze et al., 2019; Shi et al., 2019). Nevertheless, their stability is still a critical issue and easy oxidation as well as distortion of their geometry in the excited state are the main drawbacks of such systems. (Itoh et al., 2002; Li et al., 2006; Brühwiler et al., 2009). A possible solution to decrease the non-radiative decay is to take advantage of a pronounced thermally activated delayed luminescence exhibited by some of these complexes. (Palmer and McMillin, 1987; Deaton et al., 2010; Linfoot et al., 2014; Baranov et al., 2020). This process consists in the back population of the lowest excited singlet state from the triplet luminescent level. This process could also solve the long-lived radiative emission decay for the T1→S0 transitions of Cu(I) compounds, typically in the range of hundreds of μs up to ms. (Armaroli et al., 2007; Costa et al., 2012; Keller et al., 2018; Weber et al., 2018). Usually, compounds with such a long excited-state lifetime would not be well suitable as emitters in devices or in solution since quenching processes such as e.g., triplet-triplet annihilation or bimolecular deactivation can easily occur.
In addition, simple compounds such as Cu(I) clusters, (Kyle et al., 1991), containing halides as bridging ligands between the Cu(I) ions, are of great potential as emitters. Still, their formation and existence are restricted to particular conditions. From such versatile species, it is possible to generate a series of emissive compounds by simple substitution of the halide with coordinating ligands such as pyridines or phosphines. (Liu et al., 2012; Parmeggiani and Sacchetti, 2012; Benito et al., 2014).
Indeed, previous work has shown that three main products from reactions of CuI and pyridine, namely [CuI(py)]2 [CuI(py)2]2, and [CuI(py)]4 (py = pyridine), can be formed, showing blue, green, and orange emission, respectively, in the solid-state at room temperature. (Ford et al., 1999). Phosphine molecules have also been investigated as strong ligands in the synthesis of metal clusters due to the excellent coordination properties of phosphine that can stabilize metal clusters. (Perruchas et al., 2011). In general, a way to stabilize transition metal complexes (i.e., preventing oxidation and distortion) can be performed by their encapsulation inside porous nanocontainers. For instance, the successful insertion of transition metal complexes inside mesoporous silica nanoparticles was already performed by De Cola et al., Costa et al. and Hofkens et al., using Ir(III) (de Barros e Silva Botelho et al., 2011; Ezquerro et al., 2019) Pt (II) (Atoini et al., 2018) and Cu(I)complexes (Donato et al., 2018) and Ag(I) clusters. (Fenwick et al., 2016).
This work presents an alternative strategy to stabilize the Cu(I) clusters by their encapsulation in inert silica-based porous materials. We demonstrate that a straightforward sublimation of copper iodide into the pores leads to the formation of luminescent clusters. In addition, the diffusion of a ligand inside the pores results in fast coordination to the Cu(I) ion and a change in the emission color are observed.
Porous silica-based materials such as zeolite L, zeolite Y, MCM-41, and SBA-15 were selected as convenient nanocontainers to be investigated as hosts for the formation of luminescent copper iodide clusters. The different materials differ in crystallinity, alumina content, charge, pore size, and order. For this work, the most relevant feature is the pore size that can influence the size and stability of the resulting clusters and their loading. Moreover, it should also play a role in the stability of the Cu(I) species since bigger pores might allow faster oxidation of the metal cation into Cu(II) due to faster oxygen diffusion inside the pores. Zeolites L (disk-shaped, 1 µm) were first filled by CuI salt by sublimation in a closed system (see experimental section) at high vacuum (10–9 bar) and 200 °C for 2 h (Scheme 1). Upon cooling, we observed a deep red luminescence coming from the zeolite L crystals.
This preliminary observation rules out the emission of the CuI salt since its weak emission is centered at 415 nm (Supplementary Figure S1). On the other hand, the copper clusters containing iodine as bridging ligand display a red emission similar to the one we recorded. It is important to note that the material displays the same emission in vacuo and after air exposure. As previously mentioned, the porous material plays a role as a stabilizing agent against the oxidation of Cu(I) into Cu(II). Moreover, the sublimation technique is not effective with complexes and clusters based on heavier transition metal ions, such as for instance platinum (II) or iridium (III), even with a longer heating time. Therefore, we can point out the quasi exclusivity of this approach for copper-based species.
In order to gain more insight into the photophysical properties of the species entrapped in the pores, excitation and emission spectra of CuI@ZeoL were recorded in the solid-state. The emission is characterized by a broad, non-structured band, with maximum of 710 nm (Figure 1) and an emission quantum yield of 14%.
FIGURE 1. Excitation (λem = 700 nm) and Emission (λexc = 414 nm) spectra of CuI@ZeoL in solid-state condition; inset is the photograph of CuI@ZeoL inside the glass tube. The peak at 410 nm is an artifact.
The origin of the emission can only be assigned to a triplet “cluster-centered” (3CC) excited state, which has mixed iodide-to-metal charge transfer (3XMCT) and metal-centered transfer (3MC: d10 → d9S1Cu). The excitation spectrum recorded at λem = 710 nm shows a broad absorption at about 350 nm. The excited-state lifetime is a few microseconds (see Table 1), with complex multiple decay components pointing out the phosphorescence behavior of the material.
Confocal microscopy images (Supplementary Figure S2) clearly show that the emission originates from the zeolite crystals, emphasizing the successful encapsulation of CuI inside ZeoL, and the formation of a new emissive species. Moreover, different ratios in weight of CuI and ZeoL were studied (see supporting information) obtained by subliming different amounts of CuI in the same amount of zeolite (100 mg). In particular the ratios 10:100, CuI@ZeoL-A; 60:100 CuI@ZeoL-B; 100:100 CuI@ZeoL-C, after indicated only as CuI@ZeoL, and 200:100 CuI@ZeoL-D were prepared and the emission spectra of the obtained materials are depicted in Figure 2A. These data show that the emission energy is independent from the amount of CuI added to the zeolite.
FIGURE 2. Structure and TEM image of the different porous materials used in this work (top); emission spectra of CuI@ZeoL with different ratios of CuI:ZeoL (CuI@ZeoL-A red line; CuI@ZeoL-B: blue line; CuI@ZeoL-C: orange line; CuI@ZeoL-D: green line) (A); effect of host/pore size to the emission spectra (B) in solid-state condition. On top of the graphs is depicted the representation of the host used in Figure 2B λexc = 414 nm.
Through the SEM images we can see that the morphology of the zeolite and silica material did not change before and after loading the copper source (Supplementary Figure S3), indicating that the loading of CuI did not destroy the porous structure of our materials. Meanwhile, we have obtained a red emission from the loaded CuI inside each host, which indicated that the copper source was successfully loaded inside the porous material. The photophysical properties of the resulting hybrid materials are discussed below.
To prove the confinement effect dictated by the porous containers, needed to obtain such a red emissive material, copper iodide powder was treated alone in the same manner (high vacuum and temperature), and after the treatment, we could not observe any red luminescence from this material. Moreover, the same procedure was performed with bulk, non-porous silica (Supplementary Figure S1). We clearly see the same emission as CuI alone, i.e., an emission maximum at around 415 nm. This emphasizes the importance of the pores rather than the substrate to obtain emissive Cu(I) clusters. On the side, we performed nitrogen sorption experiments to show that the zeolites are indeed filled.
A comparison between the zeolites before and after sublimation of copper iodide reveals a decrease of the pore width and confirms a certain loading of the zeolites.
To understand the effect of the pore size, we have sublimed 100 mg of CuI inside materials featuring bigger pores, namely Zeolite Y (1.2 nm pore), MCM-41 (2 nm pore size), and SBA-15 (10 nm pore size), see Supplementary Materials section. The MCM-41, having 2 nm pore size and 100 nm in diameter, (Trewyn et al., 2007), and the SBA-15 mesoporous silica (SujandiPrasetyanto and Park, 2008) were synthesized following a typical sol-gel micelle-templated process. All those sublimation processes lead to the same deep red luminescence. (Table 1; Figure 2B). These results indicate the similarity of the cluster’s structure, without any dependence on the host used. However, it is essential to note that the cluster inside bigger pores is less stable toward degradation since it allows a more important penetration of oxygen. In the two extreme cases, i.e., zeolite L and SBA 15, the red luminescence of the former does not decrease after several months, while it only takes a few days for the latter to lose its luminescence due to the oxidation of the Cu(I). The loading of the two materials: CuI@ZeoL and CuI@ZeoY (both of them with a ratio CuI:Zeo 100:100), was determined using the ICP-MS technique. The loading of the latter (CuI@ZeoY, 28%) is higher than that of the former (CuI@ZeoL, 13%), and this difference can be easily explained by the difference in the pore size, larger in the case of ZeoY. However, we could not detect evident changes in the emission maximum or excited state lifetimes suggesting that the luminescent species are the same (see Table1).
Knowing that Cu(II) based species are not luminescent, we could assume that the Cu(I) cluster was not oxidized since our material is strongly luminescent. However, the hypothesis of undesirable partial oxidation cannot be disproved only by the use of photophysical studies. In order to ensure there is no Cu(II) in our material, the oxidation state of Cu was then investigated by X-ray photoelectron spectroscopy (XPS) measurements (Supplementary Figure S5). As a control, pure CuI gives us the Cu2p3/2 peak at 932.9eV and I3d5/2 at 620.0eV. The spectra from Cu clusters show the Cu2p3/2 peak at 933.5eV and I3d5/2 at 620.9eV. The binding energy of copper stays in the Cu(I) region compared to the other copper halogen compounds. But more importantly, the spectrum does not feature any signal around 960eV, typical for Cu(II) (satellite signal of Cu(II)).
Both the Cu and I peaks shifted to a little bit higher binding energy in the clusters, which is due to the interactions with the oxygen atoms on the zeolite channels. All the signals present only one contribution in agreement with only one chemical environment, indicating that there is no oxidation of Cu(I).
To assess the structure of the CuI cluster, the species formed inside the zeolite were analyzed by powder X-ray diffraction (PXRD), and the results were determined by Total Pattern Analysis Solutions (TOPAS) software using advanced Rietveld refinement. (Coelho, 2003). Quantitative phase analysis QPA in the TOPAS software is based on the method first described by Hill and Howard. (Hill and Howard, 1987). This method is based on the assumption that 1) all phases in the specimen are identified, 2) all phases are crystalline, and 3) the crystal structures of all phases are known. By comparing the experimental data, calculated data, and its residual (Figure 3), we found that the Cu(I) clusters inside the zeolite have cubic type crystal structure with F-43m space group and a = b = c = 6.056 Å, featuring a Rietveld weigh profile (Rwp) value of 7.8. By performing calculations, we obtained the crystal symmetry, lattice parameter, and we could also determine the atomic composition of the cluster. Finally, we were able to reconstruct the crystal structure of the CuI cluster, as shown in Supplementary Figure S6. The position of the copper cluster inside the zeolite Y was then reconstructed and shown in Supplementary Figure S7.
FIGURE 3. TOPAS calculation result for CuI@ZeoL. The black curve shows the measured XRD data, the red curve shows the calculated Rietveld fitting, and the blue curve shows the difference between the former and the latter.
Two different organic ligands, i.e., pyridine and triphenyl-phosphine, were inserted in CuI@porous materials. As explained here above, porous hosts have small pores. In other words, zeolites are better candidates for stability reasons.
In this work, zeolites L present too small channels to host the organic ligands. For these reasons, the material chosen for the following of this work is zeolite Y, most likely the best candidate for such a purpose. (Yu et al., 2014; Łukarska et al., 2017). After the addition of the organic ligands into CuI@ZeoY, the characterization of the resulting materials was performed. In order to determine the structure of the resulting CuI cluster formed in zeolites, an interesting approach was to use PXRD analysis by comparing.
One of the materials we obtained with a simulation of the addition of zeolite Y and the already.
Reported (C5H5CuIN)4 cubane cluster (Supplementary Figure S8). (Raston and White, 1976). PXRD studies showed a high degree of crystallinity of the materials, and again by using TOPAS software, (Coelho, 2003), we were able to identify our guest cluster as a cubane-like structure (Scheme 2). It is important to mention the matching between the experimental and the measured patterns, and the Rwp found is 6.5, which is a very good value for such studies.
SCHEME 2. Schematic representation of CuI cluster functionalized with pyridine ligands featuring a cubane-like structure. (Ford et al., 1999).
To confirm this result, the compound was isolated from the zeolites by breaking the latter by means of a sonication process. The resulting species was characterized by 1H NMR, DOSY NMR, and HR ESI-MS. NMR spectra of the CuIPy cluster were compared with that of pyridine. While 1H NMR of both molecules, i.e., CuIPy cluster and pyridine, have the same protons, they display similar spectra, featuring three signals in the aromatic region; the main difference relies on a downfield shift of the signals referring to CuIPy cluster, by around 0.5 ppm (Supplementary Figure S9).
DOSY NMR spectra (Supplementary Figure S10, Supplementary Table S1) show a diffusion coefficient of 1.46 nm s−1 and 2.93 nm s−1 for CuIPy cluster and pyridine, respectively. Hence, the molecular volumes determined for pyridine and the CuIPy cluster are respectively 21 Å3 and 169 Å3.
HR ESI-MS spectrum (Supplementary Figure S11) shows an m/z value of 1,075.51, matching perfectly the theoretical predictions of Cu4I4(C6H5N)4. Thus, the isolated molecule was confirmed to be (CuIPy)4.
Copper-iodine clusters were intensively studied by Peter C. Ford. In this regard, a cubic copper-iodine bearing a pyridine on each copper atom was characterized. (Liu et al., 2012). Inline of Ford's studies, our sublimation process, followed by pyridine addition, aims to obtain the same cluster inside the zeolites Y pores.
It has been noticed that both clusters containing organic ligands, i.e., CuIPy@ZeoY and CuIPPh3@ZeoY, feature a thermochromic behavior. In fact, in thermochromic clusters, the luminescent thermochromism is caused by a relative intensity change of two distinct emission bands. (Kitagawa et al., 2010; Perruchas et al., 2010; Fenwick et al., 2016; Tsuge et al., 2016; Baekelant et al., 2019; Baekelant et al., 2020). Hence, the emission of the materials was also measured at 77K (Figure 4), and a correlation could be found between the temperature and the emission profiles. Indeed, the high energy emission band at around 400–450 nm is dominant at low temperature, assigned to a halogen-to-ligand charge transfer (XLCT) transition state.
The low energy emission band at around 575 nm for CuIPy@ZeoY and 695 nm for CuIPPh3@ZeoY is dominant at room temperature, which is referred to a cluster-centered excited state (3CC) and can be affected by outer environments such as solid/solution state, temperature, or pressure. Regarding CuIPy@ZeoL, the photophysical data match perfectly with the cluster previosuly described eliminate in Peter Ford studies, this allows us to confirm the cubane like structure, referring to the following chemical formula: (Cu4I4Py4). (Kyle et al., 1991; Ford et al., 1999).
When the tube was taken out from liquid nitrogen and exposed to the irradiation of a UV lamp (365 nm), the interesting reversible thermochromic luminescence from yellow (298 K) to blue (77 K) can be easily distinguished by the naked eye or recorded by a digital camera. Solid-state luminescence performed from 298 to 77 K is in accordance with the thermochromic luminescence and the variation of luminescent intensity. At room temperature, the maximum of the single emission is found at 575 nm with an excited state lifetime featuring a bi-exponential decay, 11 and 2.37 μs, and an emission quantum yield of 34% (Figure 4; Table 1). The long excited-state lifetime, in the microsecond range, indicates the phosphorescence behavior, which is not surprising coming from such clusters. With the decrease of temperature from room temperature to 77 K, the emission band is progressively blue-shifted from 575 to 435 nm, which is accompanied by a widening of the bandwidth and a decrease in emission intensity. This dramatic hypsochromic shift of about 140 nm makes the detection of the color change by the naked eye easier. Without the presence of an organic ligand, the origin of the emission can only be assigned to a triplet “cluster-centered” (3CC) excited state, which has mixed iodide-to-metal charge transfer (3XMCT) and metal-centered transfer (3MC: d10 → d9S1Cu). However, in the case of Cu4I4L4, other transitions such as organic-ligand-related excited states, i.e., halide to ligand charge transfers (XLCT), must be considered.
Moreover, it has been shown that the thermochromic luminescence is due to a significant change in Cu⋯Cu distances that decreases together with the temperature. (Ford et al., 1999). Indeed, at lower temperatures, Cu⋯Cu distances become shorter, and the bonding character becomes more and more obvious. The transition of the LT phase to the HT phase resulted in some shortening of Cu⋯Cu distances. The origin of thermochromic luminescence in CuI cluster is quite different from cubane Cu4I4L4 cluster-based compounds, where relative intensities of 3CC and XLCT emissions with the temperature are responsible for the thermochromic luminescence. (Tard et al., 2008).
In conclusion, we have synthesized luminescent copper iodide inside porous nanomaterials, with a relatively high photoluminescence quantum yield. The nanomaterial allow high stability due to its protection from oxygen, preventing the oxidation of Cu(I) into Cu(II). A certain correlation was also found between the pore size and the stability of the Cu(I) cluster. By adding an organic monodentate ligand after sublimation of CuI, i.e., pyridine or triphenylphosphine, we are able to tune the luminescence energy, the resulting luminescence featuring an important bathochromic shift. The materials also showed a thermochromic behavior, which led to a significant hypsochromic emission shift of 140 nm. Thanks to several characterizations, among them ESI-HRMS, DOSY NMR, and PXRD experiment followed by Rietveld refinement and simulation, we were able to identify Cu(I) cluster and CuIPy cluster, the latter having a cubane like structure. This finding may lead to the use of copper, much cheaper than its transition metals counterparts, as alternative materials in applications such as lighting devices.
The original contributions presented in the study are included in the article/Supplementary Material, further inquiries can be directed to the corresponding authors.
All authors listed have made a substantial, direct, and intellectual contribution to the work and approved it for publication.
European Union FP7-SACS Project (Grant agreement no. 2012-310651). Ministry of Research, Technology and Higher Education of the Republic of Indonesia Scheme for Academic Mobility and Exchange (SAME)—“PHC-Nusantara.
The authors declare that the research was conducted in the absence of any commercial or financial relationships that could be construed as a potential conflict of interest.
All claims expressed in this article are solely those of the authors and do not necessarily represent those of their affiliated organizations, or those of the publisher, the editors and the reviewers. Any product that may be evaluated in this article, or claim that may be made by its manufacturer, is not guaranteed or endorsed by the publisher.
Cyril Antheaume and Bruno Vincent are gratefully thanked for their contribution on NMR and MS analysis.
The Supplementary Material for this article can be found online at: https://www.frontiersin.org/articles/10.3389/fchem.2022.829538/full#supplementary-material
Armaroli, N., Accorsi, G., Cardinali, F., and Listorti, A. (2007). in Photochemistry and Photophysics of Coordination Compounds I. Editors V. Balzani, and S. Campagna (Springer Berlin Heidelberg), 280, 69–115.
Atoini, Y., Prasetyanto, E. A., Chen, P., Silvestrini, S., Harrowfield, J., and De Cola, L. (2018). Luminescence of Amphiphilic Pt II Complexes Controlled by Confinement. Chem. Eur. J. 24, 12054–12060. doi:10.1002/chem.201802743
Baekelant, W., Aghakhani, S., Fron, E., Martin, C., Woong-Kim, C., Steele, J. A., et al. (2019). Luminescent Silver-Lithium-Zeolite Phosphors for Near-Ultraviolet LED Applications. J. Mater. Chem. C 7, 14366–14374. doi:10.1039/c9tc04674g
Baekelant, W., Romolini, G., Sun, L., De Ras, M., Fron, E., Moreira, T., et al. (2020). Tunable white Emission of Silver-Sulfur-Zeolites as Single-phase LED Phosphors. Methods Appl. Fluoresc. 8, 024004. doi:10.1088/2050-6120/ab7169
Baranov, A. Y., Berezin, A. S., Samsonenko, D. G., Mazur, A. S., Tolstoy, P. M., Plyusnin, V. F., et al. (2020). New Cu(I) Halide Complexes Showing TADF Combined with Room Temperature Phosphorescence: the Balance Tuned by Halogens. Dalton Trans. 49, 3155–3163. doi:10.1039/d0dt00192a
Benito, Q., Le Goff, X. F., Maron, S., Fargues, A., Garcia, A., Martineau, C., et al. (2014). Polymorphic Copper Iodide Clusters: Insights into the Mechanochromic Luminescence Properties. J. Am. Chem. Soc. 136, 11311–11320. doi:10.1021/ja500247b
Brühwiler, D., Calzaferri, G., Torres, T., Ramm, J. H., Gartmann, N., Dieu, L.-Q., et al. (2009). Nanochannels for Supramolecular Organization of Luminescent Guests. J. Mater. Chem. 19, 8040–8067. doi:10.1039/b907308f
Bünzli, J.-C. G., and Piguet, C. (2005). Taking Advantage of Luminescent Lanthanide Ions. Chem. Soc. Rev. 34, 1048–1077. doi:10.1039/b406082m
Coelho, A. A. (2003). Indexing of Powder Diffraction Patterns by Iterative Use of Singular Value Decomposition. J. Appl. Cryst. 36, 86–95. doi:10.1107/s0021889802019878
Costa, R. D., Ortí, E., Bolink, H. J., Monti, F., Accorsi, G., and Armaroli, N. (2012). Luminescent Ionic Transition-Metal Complexes for Light-Emitting Electrochemical Cells. Angew. Chem. Int. Ed. 51, 8178–8211. doi:10.1002/anie.201201471
de Barros e Silva Botelho, M., Fernandez-Hernandez, J. M., de Queiroz, T. B., Eckert, H., De Cola, L., and de Camargo, A. S. S. (2011). Iridium(iii)-surfactant Complex Immobilized in Mesoporous Silica via Templated Synthesis: a New Route to Optical Materials. J. Mater. Chem. 21, 8829–8834. doi:10.1039/c1jm10878f
Deaton, J. C., Switalski, S. C., Kondakov, D. Y., Young, R. H., Pawlik, T. D., Giesen, D. J., et al. (2010). E-type Delayed Fluorescence of a Phosphine-Supported Cu2(μ-NAr2)2 Diamond Core: Harvesting Singlet and Triplet Excitons in OLEDs. J. Am. Chem. Soc. 132, 9499–9508. doi:10.1021/ja1004575
Donato, L., Atoini, Y., Prasetyanto, E. A., Chen, P., Rosticher, C., Bizzarri, C., et al. (2018). Selective Encapsulation and Enhancement of the Emission Properties of a Luminescent Cu(I) Complex in Mesoporous Silica. Helv. Chim. Acta 101, e1700273. doi:10.1002/hlca.201700273
Eliseeva, S. V., and Bünzli, J.-C. G. (2010). Lanthanide Luminescence for Functional Materials and Bio-Sciences. Chem. Soc. Rev. 39, 189–227. doi:10.1039/b905604c
Ezquerro, C., Fresta, E., Serrano, E., Lalinde, E., García-Martínez, J., Berenguer, J. R., et al. (2019). White-emitting Organometallo-Silica Nanoparticles for Sun-like Light-Emitting Diodes. Mater. Horiz 6, 130–136.
Fenwick, O., Coutiño-Gonzalez, E., Grandjean, D., Baekelant, W., Richard, F., Bonacchi, S., et al. (2016). Tuning the Energetics and Tailoring the Optical Properties of Silver Clusters Confined in Zeolites. Nat. Mater 15, 1017–1022. doi:10.1038/nmat4652
Ford, P. C., Cariati, E., and Bourassa, J. (1999). Photoluminescence Properties of Multinuclear Copper(I) Compounds. Chem. Rev.Compounds 99, 3625–3648. doi:10.1021/cr960109i
Frecker, T., Bailey, D., Arzeta-Ferrer, X., McBride, J., Rosenthal, S. J., and Ecs, J. (2016). Review-Quantum Dots and Their Application in Lighting, Displays, and Biology. ECS J. Solid State. Sci. Technol. 5, R3019–R3031. doi:10.1149/2.0031601jss
Hamze, R., Peltier, J. L., Sylvinson, D., Jung, M., Cardenas, J., Haiges, R., et al. (2019). Eliminating Nonradiative Decay in Cu(I) Emitters: >99% Quantum Efficiency and Microsecond Lifetime. Science 363, 601–606. doi:10.1126/science.aav2865
Hill, R. J., and Howard, C. J. (1987). Quantitative Phase Analysis from Neutron Powder Diffraction Data Using the Rietveld Method. J. Appl. Cryst. 20, 467–474. doi:10.1107/s0021889887086199
Itoh, T., Yano, K., Inada, Y., and Fukushima, Y. (2002). Photostabilized Chlorophyll a in Mesoporous Silica: Adsorption Properties and Photoreduction Activity of Chlorophyll a. J. Am. Chem. Soc. 124, 13437–13441. doi:10.1021/ja0203059
Keller, S., Prescimone, A., Bolink, H., Sessolo, M., Longo, G., Martínez-Sarti, L., et al. (2018). Luminescent Copper(i) Complexes with Bisphosphane and Halogen-Substituted 2,2′-bipyridine Ligands. Dalton Trans. 47, 14263–14276. doi:10.1039/c8dt01338a
Kitagawa, H., Ozawa, Y., and Toriumi, K. (2010). Flexibility of Cubane-like Cu4I4 Framework: Temperature Dependence of Molecular Structure and Luminescence Thermochromism of [Cu4I4(PPh3)4] in Two Polymorphic Crystalline States. Chem. Commun. 46, 6302–6304. doi:10.1039/c0cc01434f
Kyle, K. R., Ryu, C. K., Ford, P. C., and DiBenedetto, J. A. (1991). Photophysical Studies in Solution of the Tetranuclear Copper(I) Clusters Cu4I4L4 (L = Pyridine or Substituted Pyridine). J. Am. Chem. Soc. 113, 2954–2965. doi:10.1021/ja00008a026
Łukarska, M., Jankowska, A., Gapiński, J., Valable, S., Anfray, C., Ménard, B., et al. (2017). Synthesis of Fluorescein by a Ship-In-A-Bottle Method in Different Zeolites. New J. Chem. 41, 9969–9976.
Lewinski, N., Colvin, V., and Drezek, R. (2008). Cytotoxicity of Nanoparticles. Small 4, 26–49. doi:10.1002/smll.200700595
Li, S., Song, H., Li, W., Ren, X., Lu, S., Pan, G., et al. (2006). Improved Photoluminescence Properties of Ternary Terbium Complexes in Mesoporous Molecule Sieves. J. Phys. Chem. B 110, 23164–23169. doi:10.1021/jp064509d
Linfoot, C. L., Leitl, M. J., Richardson, P., Rausch, A. F., Chepelin, O., White, F. J., et al. (2014). Thermally Activated Delayed Fluorescence (TADF) and Enhancing Photoluminescence Quantum Yields of [CuI(diimine)(diphosphine)]+ Complexes-Photophysical, Structural, and Computational Studies. Inorg. Chem. 53, 10854–10861. doi:10.1021/ic500889s
Liu, Z., Djurovich, P. I., Whited, M. T., and Thompson, M. E. (2012). Cu4I4 Clusters Supported by P∧N-type Ligands: New Structures with Tunable Emission Colors. Inorg. Chem. 51, 230–236. doi:10.1021/ic2015226
Palmer, C. E. A., and McMillin, D. R. (1987). Singlets, Triplets, and Exciplexes: Complex, Temperature-dependent Emissions from (2,9-Dimethyl-1,10-Phenanthroline)bis(triphenylphosphine)copper(1+) and (1,10-phenanthroline)(triphenylphosphine)copper(1+). Inorg. Chem. 26, 3837–3840. doi:10.1021/ic00270a004
Parmeggiani, F., and Sacchetti, A. (2012). Preparation and Luminescence Thermochromism of Tetranuclear Copper(I)-Pyridine-Iodide Clusters. J. Chem. Educ. 89, 946–949. doi:10.1021/ed200736b
Perruchas, S., Le Goff, X. F., Maron, S., Maurin, I., Guillen, F., Garcia, A., et al. (2010). Mechanochromic and Thermochromic Luminescence of a Copper Iodide Cluster. J. Am. Chem. Soc. 132, 10967–10969. doi:10.1021/ja103431d
Perruchas, S., Tard, C., Le Goff, X. F., Fargues, A., Garcia, A., Kahlal, S., et al. (2011). Thermochromic Luminescence of Copper Iodide Clusters: the Case of Phosphine Ligands. Inorg. Chem. 50, 10682–10692. doi:10.1021/ic201128a
Raman, N. K., Anderson, M. T., and Brinker, C. J. (1996). Template-Based Approaches to the Preparation of Amorphous, Nanoporous Silicas. Chem. Mater. 8, 1682–1701. doi:10.1021/cm960138+
Raston, C. L., and White, A. H. (1976). Crystal Structure of the Copper(I) Iodide-Pyridine (1/1 ) Tetramer. J. Chem. Soc. Dalton Trans., 2153–2156. doi:10.1039/dt9760002153
Shi, S., Jung, M. C., Coburn, C., Tadle, A., Sylvinson, M. R. D., et al. (2019). Highly Efficient Photo- and Electroluminescence from Two-Coordinate Cu(I) Complexes Featuring Nonconventional N-Heterocyclic Carbenes. J. Am. Chem. Soc. 141, 3576–3588. doi:10.1021/jacs.8b12397
Sujandi, , Prasetyanto, E. A., and Park, S.-E. (2008). Synthesis of Short-Channeled Amino-Functionalized SBA-15 and its Beneficial Applications in Base-Catalyzed Reactions. Appl. Catal. A: Gen. 350, 244–251. doi:10.1016/j.apcata.2008.08.020
Tard, C., Perruchas, S., Maron, S., Le Goff, X. F., Guillen, F., Garcia, A., et al. (2008). Thermochromic Luminescence of Sol−Gel Films Based on Copper Iodide Clusters. Chem. Mater. 20, 7010–7016. doi:10.1021/cm801780g
Trewyn, B. G., Slowing, I. I., Giri, S., Chen, H.-T., and Lin, V. S.-Y. (2007). Synthesis and Functionalization of a Mesoporous Silica Nanoparticle Based on the Sol-Gel Process and Applications in Controlled Release. Acc. Chem. Res. 40, 846–853. doi:10.1021/ar600032u
Tsuge, K., Chishina, Y., Hashiguchi, H., Sasaki, Y., Kato, M., Ishizaka, S., et al. (2016). Luminescent Copper(I) Complexes with Halogenido-Bridged Dimeric Core. Coord. Chem. Rev. 306, 636–651. doi:10.1016/j.ccr.2015.03.022
Weber, M. D., Fresta, E., Elie, M., Miehlich, M. E., Renaud, J.-L., Meyer, K., et al. (2018). Rationalizing Fabrication and Design toward Highly Efficient and Stable Blue Light-Emitting Electrochemical Cells Based on NHC Copper(I) Complexes. Adv. Funct. Mater. 28, 1707423–1707435. doi:10.1002/adfm.201707423
Keywords: luminescence, confined space, copper clusters, zeolites, Cu(I), color tunability
Citation: Prasetyanto EA, Atoini Y, Donato L, Hsu C-W and De Cola L (2022) The Role of a Confined Space on the Reactivity and Emission Properties of Copper(I) Clusters. Front. Chem. 10:829538. doi: 10.3389/fchem.2022.829538
Received: 06 December 2021; Accepted: 15 March 2022;
Published: 05 May 2022.
Edited by:
Valeria Conte, University of Rome Tor Vergata, ItalyReviewed by:
Wai-Pong To, The University of Hong Kong, Hong Kong SAR, ChinaCopyright © 2022 Prasetyanto, Atoini, Donato, Hsu and De Cola. This is an open-access article distributed under the terms of the Creative Commons Attribution License (CC BY). The use, distribution or reproduction in other forums is permitted, provided the original author(s) and the copyright owner(s) are credited and that the original publication in this journal is cited, in accordance with accepted academic practice. No use, distribution or reproduction is permitted which does not comply with these terms.
*Correspondence: Eko Adi Prasetyanto, cHJhc2V0eWFudG9AYXRtYWpheWEuYWMuaWQ=; Luisa De Cola, bHVpc2EuZGVjb2xhQHVuaW1pLml0
†These authors have contributed equally to this work
Disclaimer: All claims expressed in this article are solely those of the authors and do not necessarily represent those of their affiliated organizations, or those of the publisher, the editors and the reviewers. Any product that may be evaluated in this article or claim that may be made by its manufacturer is not guaranteed or endorsed by the publisher.
Research integrity at Frontiers
Learn more about the work of our research integrity team to safeguard the quality of each article we publish.