- Department of Chemistry, College of Sciences, Shiraz University, Shiraz, Iran
This research introduces an oil-in-water (O/W) nano-emulsion (oil-water-
Introduction
Natural gas condensates (NGCs) often involve serious impurities such as chloride ion
In fact, the
Although different methodologies have been proposed for the
Briefly, dicopper chloride trihydroxide is considered as an appropriate compound that is useful as an effective pesticide for various plants and trees, especially pistachio (Brühl et al., 2021). The general synthetic process of this compound is often based on the reaction between copper salts and chloride compounds at the basic condition (Jackson and Fulton, 1996; Lubej et al., 2004). However, the efficiency of the formation of this compound is seriously limited due to some different side reactions. The attribution of these reactions leads to the formation of some impurities such as Cu(OH)2 and CuCl2, besides some various coordination compounds such as CuCl3− and CuCl42−. (Scott, 2000; Hussain et al., 2019; FAO/WHO, 2021). These producers are often generated on the basis of different mechanisms such as coprecipitation and crystal-based complex formation.
Besides the abovementioned problems, the lack control on other factors like size distribution and the morphology is also other problems, owing to the harness of the control of the relative supper saturation and the absence of any suitable template and capping agents (Luo et al., 2005; Lu et al., 2010; Chen and Zhong, 2022; Pathania et al., 2022). Fortunately, the introduced oil-in-water (O/W) emulsion plays significant roles as almost all of the abovementioned reagents for reaching to pure, size-controllable, and high-efficient pesticide. Therefore, the novelty of this study is brief: Not only is the Cl− and Hg(0) removal from the NGC but also is accessing an important and applicable compound. To the best of knowledge, no applicable methodology has been introduced for solving the current problem, especially at the industrial scale.
Experimental
Materials
The necessity reagents related to the nano-emulsion syntheses have been from Merck Company; their information and the NGC sample data were given in Supplementary Material S2.1. How to prepare this nano-emulsion as an O/W medium was presented in detail in Supplementary Material S2.2. Stable homogeneity (Chen and Zhong, 2022; Pathania et al., 2022) of the synthesized O/W nano-emulsion was confirmed for a long time (up to 24 h), which could be re-homogenized simply via vigorously shaking for a few seconds (maximum 10 s).
Nano-Emulsion Preparation (Standard Extracting Medium)
The HCFC-22 (R22)–treated oil was prepared using descriptions methodology in the previous study (Mohammadi et al., 2016; Banan Khorshid et al., 2021). Briefly, the stock nano-emulsion medium with oil concentration of 20.80 g L−1, as the extracting medium, was simply prepared by weighting 1,040.0 mg of this oil sample. Then, it was mixed with the triply distilled water (20.0 ml) during stirring using a magnet stirrer for a few minutes (3.0 min) at 400 ± 2 rpm using a hotplate stirrer (RT2, Denville Scientific Inc., American Cleanstat, LLC, Phenix Research Products Inc., Candler, NC, USA). In this synthetic process, the stability of the R22-treated oil for a long time. The results exhibited no significant desorption of the R22 from the oil matrix. This practically revealed no toxicity of the R22-treated oil using this procedure.
The R22 was then purged with flow rate of 5.00 ± 0.01 ml min−1 for 10.0 min using a mass flow controller (MFC, Bronkhorst high-tech. B.V., England). After that, it was transferred into a 50.0-ml glass volumetric flask (Thermo Scientific™ Nalgene™ Class B Polypropylene Copolymer Volumetric Flasks with Closure) and diluted to the mark using the triply distilled water. Then, it was vigorously shaken for about 1 min to form the homogenous nano-emulsion. The synthesized nano-emulsion was visualized using a fluorescence optical microscope (Olympus IX70, UK).
Daily dilution of the stock nano-emulsion was achieved via sampling a fixed volume of the stock solution using an “Eppendorf” pipette (Eppendorf® Research® Plus Pipettes; Sigma-Aldrich) and transferring into a glass volumetric flask. Afterward, it was stirred for 1 min and sonicated in a sonication bath (Ultrasonic cleaner, 400 W, 9.5 L, 40 kHz, C250X, MEGA Lab, UK) for 30.0 s at room temperature. Finally, it was diluted to the mark using the triply distilled water. As is expected, direct imaging the nano-emulsion, multiple circular phases revealed the existence of plenty of efficient interfaces for direct interaction with the NGC sample during simultaneous
It also should be noted that the concentration of the nano-emulsion was expressed in grams per liter. This was because of 1) better dealing with the R22 gas, 2) dependency of the concentration to the temperature, and 3) the lack of knowledge about the precise gram formula weights of each oil and the NGC. Consequently, it was impossible to introduce the composite of the synthesized nano-emulsion based on mole fraction. Therefore, in this experiment, it was decided to focus on some general terms such as flow rate, volume, and purging time for the reproducible synthesis of the nano-emulsions. Nevertheless, about other reagents such as
Liquid–Liquid Extraction
Complete detail of the LLE method is given in Supplementary Material S2.3.
Analysis Process
Experimental detail about the semi-quantitative test for the
Formation of Dicopper Chloride Trihydroxide Nanoparticles
Along the accomplishing the extraction step,
Results and Discussion
Evidence About the Morphology of the Synthetized Nano-Emulsion
In the synthesized nano-emulsions, often, these compounds are evaluated indirectly via following their chemical stability. In this nano-emulsion, because of its Brownian motion, besides its thermal stability, often, it is impossible to directly observe them even by cryo–transmission electron microscopy (TEM) imaging. Hence, in the synthesized nano-emulsion, the characterization was limited only to the evaluation of its mechanical stability for a long time, for instance, 24 h, which could be re-homogenized simply via vigorously shaking for a few seconds (maximum 10 s). This characterization was considered as appropriate and acceptable methodology for selection of the term “Nano-Emulsion” about this system (Chen and Zhong, 2022; Pathania et al., 2022).
Chloride and Mercury Removal From the NGC
The
During the optimization process (see Supplementary Materials S3.2 and S3.3), maximum insoluble inorganic particles (colloids) were observed, when using the
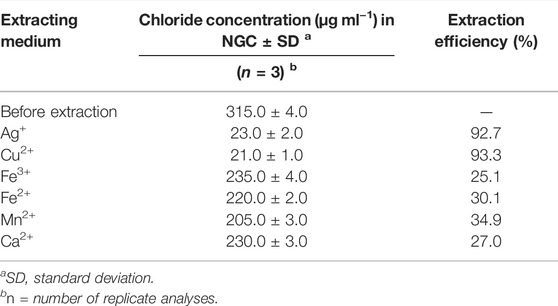
TABLE 1. Effect of nano-emulsion (oil, 5,200.0 μg ml−1) containing different cations on chloride removal from the NGC sample at 25°C.
On the basis of the results (Table 1), only the nano-emulsion, containing the
Optimization Process
Detailed study about the optimization of effective factors by on one-factor-at-a-time method such as
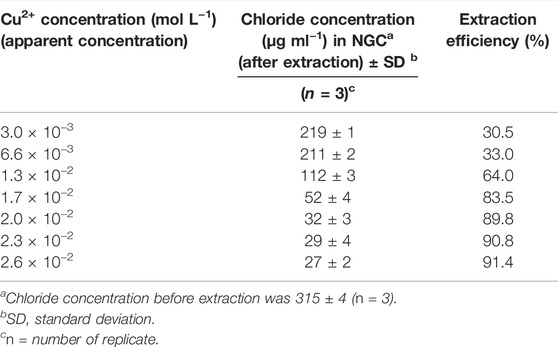
TABLE 2. Effect of Cu2+ concentration in nano-emulsion (oil, 5,200.0 μg ml−1) on chloride extraction efficiency.
On the basis of the results (Table 2), concatenation of Cu2+ was strongly dependent on the oil concentration. In another word, optimum concentration of oil simply led to decrease the amount of Cu2+ that was needed for playing role as both complex forming and de-emulsifying agent. This condition not only did not provide major change(s) in the matrix of the NGC sample during the extraction process but also majorly reduced the probable side effect of residual Cu2+ medium, possibly present inside the NGC after applying the extraction process. Therefore, it was needed to have full confidence about the optimum Cu2+ concentration in the presence of a fixed amount of oil solution using another independent analysis such as direct visualization of the AgCl particles formations (Hong et al., 2006) inside the NGC after introducing different amounts of Cu2+.
For this purpose, six nano-emulsion samples were prepared with the same oil concentration (oil, 10,400.0 μg ml−1; which was optimized in the previous section) in the presence of different Cu2+ concentrations (lower concentrations): 1.3 × 10−2, 9.9 × 10−3, 6.6 × 10−3, 3.3 × 10−3, 2.0 × 10−3, and 1.0 × 10−3 mol L−1 (apparent concentration). After performing the extraction process between 5.0 ml of NGC and the same volume according to the recommended procedure, the quantity of the generated AgCl particles was evaluated precisely according to the image photographs shown in Figure 1.
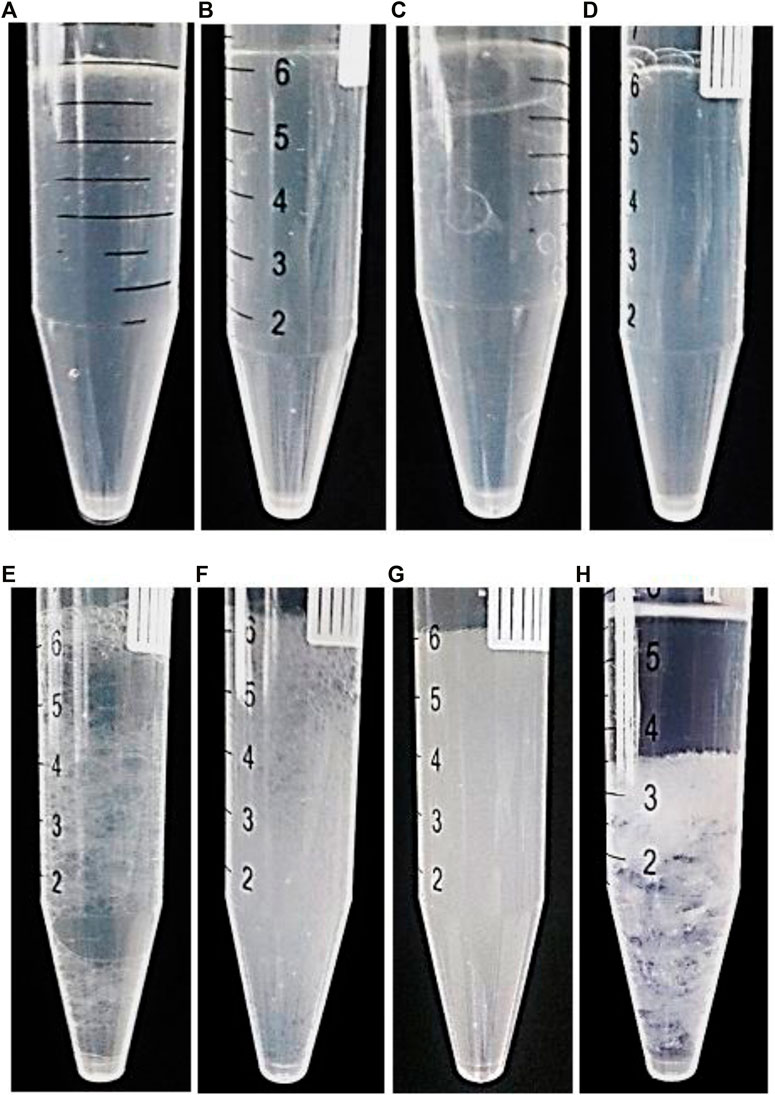
FIGURE 1. Re-optimization of Cu2+ concentration; AgCl formation after extraction by nano-emulsion in same oil concentration (10,400.0 μg ml−1) and different Cu2+ concentrations (apparent concentration) of (A) 2.0 × 10−2, (B) 1.3 × 10−2, (C) 9.9 × 10−3, (D) 6.6 × 10−3, (E) 3.3 × 10−3, (F) 2.0 × 10−3, (G) 1.0 × 10−3, and (H) 0.0 mol L−1.
On the basis of the results (Figure 1), Cu2+ (6.6 × 10−3 mol L−1 ; apparent concentration) and oil (10,400.0 μg ml−1) showed acceptable effect with chloride removal efficiency to around 100% (only by a single step). Therefore, this value was selected as optimum Cu2+ concentration.
Ionic Strength in Extractant Nano-Emulsion
Utilizing Cu(NO3)2 (6.6 × 10−3 mol L−1; apparent concentration) in extractant medium resulted in having ionic strength as large as around 20 mmol L−1. However, it should be noted that, to prevent NGC contamination, it was tried not to use any further salts to further controlling the ionic strength. In addition, more ionic strength led to have strong influence on the commercial cost and industrial application of the NGC fluids. Fortunately, at this condition, acceptable chloride removal efficiency was evaluated for the NGC samples.
Effect of Temperature on the Extraction Process
Clearly, besides the thermodynamic equilibrium constant, the kinetics of the reactions strongly depends on the temperature of the reactions. However, in a flammable and volatile sample such as NGC, high temperature was not so safe for the laboratory experiments. Therefore, for more safety and for more simplicity, it was recommended to operate the removal process at room temperature. Providentially, the chloride removal efficiency in this study was so high that small fluctuation in the temperature seemed to have no significant effect(s) on the chloride removal efficiency of the NGC samples.
Figures of Merit
The CV-AAS method was utilized to determine the concentration of Hg(0) the same as 231 ± 15 ng ml−1 (n = 3) in the raw NGC. This result pointed to the serious effect(s) of Hg(0) in the condensate. Hence, after treating the NGC with the nano-emulsion at the optimum condition, extraction (mass transfer) of the Hg(0) from the NGC phase to the aqueous one was followed during applying the de-emulsification process. At this condition, change in the oxidation number of the mercuric species from zero to the Hg22+ and/or Hg2+ using oxidants like (for instance H2O2) could seriously limit the toxicity and danger of mercury inside the receiving aqueous solution.
The IEC analysis also revealed the Hg2+ concentration in the aqueous phase to be 228 ± 6 ng ml−1 (n = 3). The result was in good agreement with the Hg2+ concentration, estimated using the CV-AAS (218 ± 7 ng ml−1, n = 3). However, for more confidence, the CV-AAS analytical process on the treated NGC revealed the residual Hg concentration to be below 10.0 ng ml−1.
For the uncertainty of the Hg content in the NGC, this value is related to the reproducibility of the mercuric element. For the efficiency, we deal with repeatability (for single NGC sample). In addition, for this analysis, we handle the CV-AAS, as a reliable method with detection limit less than ∼2 ng ml−1 and precision (reproducibility, RSD %) below around 4.0%. In addition, the statistical test such as (t-test) points to the presence or absence of any the Hg levels. Hence, the selected method for mercury removal showed removal efficiency between 90% and around 100% (deepening on the nature of the NGC). This pointed to its effective capability for removal of toxic reagents such as Hg(0) from the NGC samples along with their transfer and protected into a viscose fluid like oil medium.
Nevertheless, to confirm this claim, the IEC analyses also pointed to small concentration of the
Reusability of the Synthesized Nano-Emulsion
The reusability of the synthesized nano-emulsion was also evaluated. The experiments showed that the synthesized nano-emulsion at the optimum
Selectivity of the Extraction Process
The selectivity of this method was evaluated during extraction and separation of different kinds of anions such as Cl−, Br−, and S2− and trace quantity of RS−, presented in the NGC matrix according to chronograms shown in Figure 2.
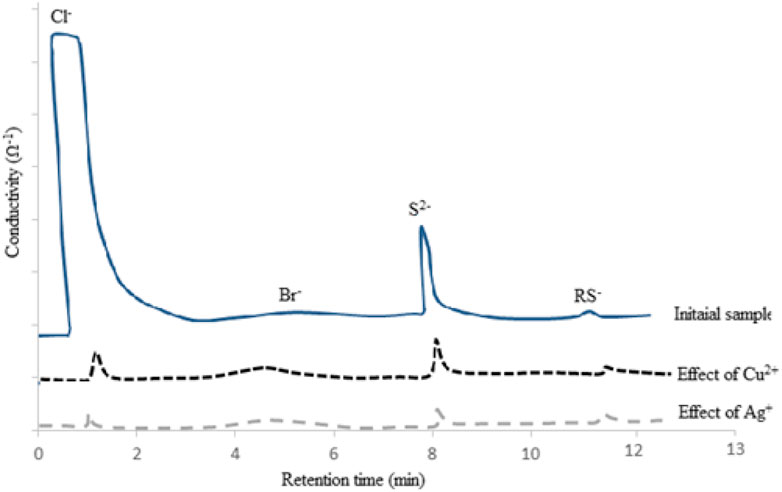
FIGURE 2. Ion-exchange chromatograms showing selectivity as well as effects of Cu2+ and Ag+ on Cl− and S2− removal.
On the basis of the IECs, not only the capability of the method was investigated for chloride removal purposes but also the effective role of de-emulsifying agents such as Cu2+ and Ag+ has been studied. On the basis of the results, the synthesized nano-emulsion had enough capability for removal of anionic species such as Cl− and S2−. For the Br− and RS− ions, probably large sizes and trace quantity of these anions limited their removal by using this LLE technique.
Non-corrosive Property of the NGC
Corrosion test was performed to investigate the
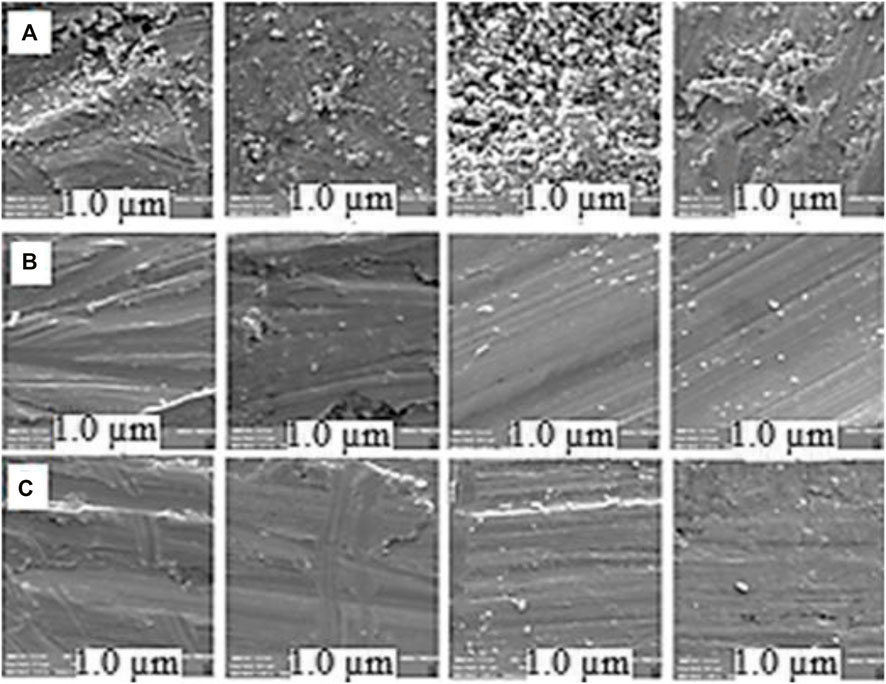
FIGURE 3. Row FE-SEM images of iron fragments, placed inside row (A) pristine NGC and row (B) treated condensate. Row (C) FE-SEM images of fresh iron fragments as blanking control.
As shown (Figure 3), no significant change was observed on the surface structure of the treated Fe fragments versus the untreated and control ones. In addition, no major weight loss was observed in the Fe fragments, contacted to the treated NGC, opposed to the control ones, which were introduced to the pristine NGC under similar conditions.
According to the weight loss analysis, the non-corrosion efficiency was also defined as the mass gradient (weight loss) of the Fe fragment before and after contact with the NGC at a fixed time interval vs. the mass of the fresh fragment, × 100. In this experiment, this value was estimated to be >90%. Therefore, the performed extraction methodology on the pristine NGC was successful for simultaneous removing of both
Proposed Mechanisms
Because of the sophisticated matrix of the condensate, it was not easy to report certain mechanism for the Hg(0) and the
In Figure 4, we adopted the IEC system that utilized suppressor. This module played role as multiple (at least four) ports: 1) memory effect elimination, 2) pH controlling system, 3) ionic strength controlling, and 4) a transient value for the transport of the samples toward the EC detector. These features were automatically arranged via controlling the mass transfer of different liquid media such as buffer solution and solvent. In this system, for the retention time of 20.0 min, the sensitivity of the system for the Cl− removal (at large scale) was adjusted via setting the mass transfer broadening of the analyte using the suppressor. This was achieved to rapidly control the mass transfer of the analyte at the interface (dead volume) between the column and the EC detector. This caused to more reliably analyze the responsible species as maximum as possible. That is why, in Figure 4, as clearly seen, the onset peak at 20 min was tilted backward toward the Y axis. This process was consequently general in the chromatographic systems. On the basis of this result, the synthesized nano-emulsion was considered as suitable intermediate medium for effective interaction between the
For this purpose, the molar concentrations of the species were estimated at different chloride concentrations based on the mole fractions and their thermodynamic constants at the aqueous solution at room temperature. This process was provided using a program the written Visual Basic 6. This program was based on the related mass/charge balance equations (Zhang et al., 2014) (see Embedded Visual Basic 6, VB6 program, Zip Files). Equations of formation copper(II) chloride complexes and their formation constants are shown in Eqs. 1–(4 (Zhang et al., 2014). In addition, other prediction and some different theoretical calculations for the estimated mole fractions of each probably generated species majorly promoted this proposed mechanism.
On the basis of the results, molar ratio of species such as CuCl2, CuCl3−, and CuCl42− was very low (order of below 10−15 mol L−1). Whereas molar concentrations of species such as Cu2+ and CuCl+ had been estimated at the order of about 10−4 mol L−1. This result was probably in good agreement with the probable formation of CuCl+, estimated by the IEC as shown in Figure 4.
However, this result also pointed to the effective role of the
Nevertheless, precisely talking about the mechanism of extraction needs confidence knowledge about other factors such as role of R22, behavior of -NH2 functional group in oil and/or formation of other intermediates like ion-pairing. These were considered as a good topic for future studies in this field. The proposed mechanism for the effect of the -NH2 functional group is shown in Eq. 5. In addition, the proposed mechanism for the effect of ion-pairing formation is shown in Eq. 6, where Mn+ is ion pair of Cl− in the NGC.
Formation of Dicopper Chloride Trihydroxide Nanoparticles
Another part of this study was related to the formation of the Cu2(OH)3Cl nanoparticles by the precipitation process after addition of NH3 solution (25%) for controlling the acidity value to ∼7.5. The formation of these nanoparticles was achieved after applying the extraction process on the NGC samples according to the recommended procedure. On the basis of Eq. 4.7 (Tanimizu et al., 2007), removed chloride ion in the presence of Cu2+ and OH− led to synthesize Cu2(OH)3Cl particles based on the following reaction (Eq. 7):
Effective factors such as Cu2+ concentration and the acidity were considered as the most important factor during the synthesis of Cu2(OH)3Cl nanoparticles.
Effect of Acidity
At pH values higher than ∼7.5, Cu2(OH)3Cl was unstable and could be converted to Cu(OH)2 (Eq. 8) (Frost et al., 2002; Boita et al., 2014). Therefore, pH controlling was very important factor in the workup step. Figure 5 shows the precipitate in different pH values.
Consequently, a pH value as large as ∼7.5 (that was adjusted by commercial NH3 solution, purity 25.0%, W/W) was selected. On the basis of the images, the promotion of pH from ∼7.5 to ∼9.0 led to have dark color precipitation due to the formation of Cu(OH)2 according to Eq. 8:
Effect of Different Bases on the Sedimentation Process
The effects of different bases such as NaOH and NH3 were studied. Sedimentation was occurred in both cases, but NH3 was adopted for pH controlling in the workup step for facilitation of the procedure. This was due to the effectiveness of NH3 for buffering the solution. However, the buffer capacity of NH3 was low at pH ∼7.5, but some side effects like formation of by-product precipitation of other buffer compounds such as phosphate limited the use of other buffer species.
Effect of Cu2+ as de-emulsifying Reagent on the Formation of Dicopper Chloride Trihydroxide Nanoparticles
To form the precipitation, after extraction step by nano-emulsion, the extractant medium (nano-emulsion) was separated and Cu(NO3)2 salt was added to it to increase the Cu2+ concentration up to about 1.7 × 10−2 mol L−1. Then, it was centrifuged for 5 min at 3,000 rpm to demulsify the nano-emulsion and to remove oil from solution. Figure 6A shows the nano-emulsion (oil, 6.6 mmol L−1 Cu2+ and 10,400.0 μg ml−1) before extraction. Whereas, Figure 6B shows the nano-emulsion after extraction (before demulsifying step). Figure 6C exhibits the solution after applying the de-emulsification process on the NGC samples (i.e., after removing oil from internal wall of sample tube). This was therefore ready for addition of NH3 and formation the sedimentation.
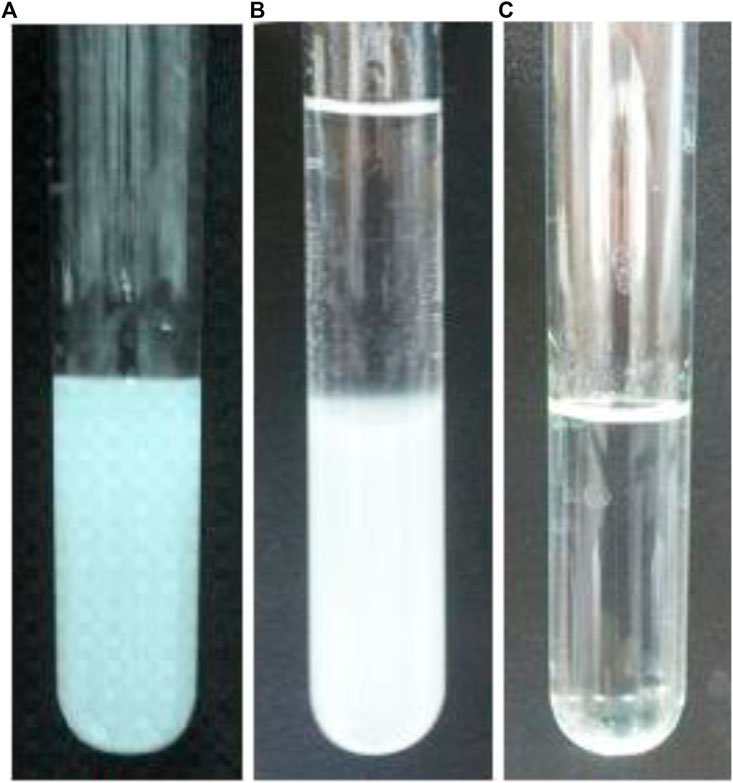
FIGURE 6. Photographic images showing (A) nano-emulsion (oil, 6.6 × 10−3 mol L−1 Cu2+ and 10,400.0 μg ml−1) before extraction, (B) nano-emulsion after extraction (before demulsifying step), and (C) solution after applying the de-emulsification process.
The addition of NH3 should be occurred after applying the de-emulsification during introducing Cu2+. At this condition, Cu2(OH)3Cl nanoparticles were observed in the aqueous phase. On the basis of the direct observation (Figure 6), at least 1.7 × 10−2 mol L−1 concentration of Cu2+ was needed for this purpose during centrifuging for 5 min at 3,000 rpm. This result was in good agreement with Cu2+ concentration that was needed for the Cu2(OH)3Cl formation. It should be noted that increasing Cu2+ concentration decreased time and rate of centrifuging step but formed Cu(OH)2 and Cu2(OH)3Cl precipitate. Therefore, these condition was selected as optimum procedure during formation of Cu2(OH)3Cl nanoparticles.
Stability of Dicopper Chloride Trihydroxide Nanoparticles: Effect of Drying Step
Solid-state product was unstable in humidity condition after ∼24 h. This was because this product had probably been converted to Cu(OH)2. Therefore, it was recommended to air dry the synthesized sediments by a fan for
Characterization of Dicopper Chloride Trihydroxide
In this study, the formation of Cu2(OH)3Cl was confirmed by the following analytical techniques. Because of the presence of competition between the formation of Cu2(OH)3Cl and Cu(OH)2, sometimes, characters of Cu2(OH)3Cl were needed to be compared to the Cu(OH)2. On the basis of the literature, there are several reactions in which Cu2(OH)3Cl and Cu(OH)2 can compete with each other. Some of the reactions are shown in Eqs 9–12 (Frost et al., 2002; Boita et al., 2014):
According these equations, the structures of Cu(OH)2 and Cu2(OH)3Cl should be comprised in detail.
Comparison Between the Structures of Dicopper Chloride Trihydroxide and Copper Hydroxide
For precise evaluation of the difference between Cu2(OH)3Cl and Cu(OH)2, the structures of these two compounds were compared to each other (Frost et al., 2002; Boita et al., 2014). On the basis of these references, the Cu2(OH)3Cl compound consisted of functional groups such as Cu-O, O-H, and Cu-Cl. Cu2+ had been coordinated with -OH and -Cl. In addition, the O-H bonds have been bridged through the hydrogen bonding; whereas, for the Cu(OH)2, there were some functional groups such as Cu-O and O-H bonds. Consequently, compared to the Cu2(OH)3Cl, the difference between the functional groups was only related to the presence of Cu-Cl functional group in the Cu2(OH)3Cl compound. Far Fourier transform infrared (FT-IR) spectra of Cu2(OH)3Cl and Cu(OH)2 are shown in Figure 7.
No significant difference was observed during compassion between the Far FT-IR spectra of Cu2(OH)3Cl and Cu(OH)2. However, small difference was exhibited between the Cu-Cl functional groups at frequencies of 245–250 cm−1. The presence of several shoulders at this frequency range pointed to the presence of different Cu-Cl bonds in the synthesized nanoparticles vs. other copper species such as Cu(OH)2. However, for characterization of the sample with more detail, middle FT-IR spectrometry was utilized (Figure 8).
On the basis of the middle FT-IR spectra, the observation of strong peaks at frequencies of 473 and ∼825 cm−1 was related to the Cu-O functional group of Cu(OH)2; whereas the observation of significant shift to higher frequencies was probably related to the presence of hydrogen bond in the Cu2(OH)3Cl compound. Compared to the Cu(OH)2, the presence of two split absorption bonds at frequency of around 3,420 cm−1 clearly pointed to the presence of hydrogen bonds in Cu2(OH)3Cl.
Raman spectroscopy was also considered as another applicable tool for characterization of Cu2(OH)3Cl, as shown in Figure 9 (Thermo Nicolet FT-Raman, 670). The presence of significant peak at frequency of around 250 cm−1 was attributed to the Cu-Cl functional group, which was in good agreement with that estimated in literature (Frost et al., 2002; Boita et al., 2014). Compared to Cu(OH)2, major shift was observed on the Raman spectra at frequencies between 450 and 500 cm−1. This result again differentiated the formation of Cu2(OH)3Cl from Cu(OH)2.
The X-ray powder diffraction (XRD; Bruker’s X-ray Diffraction D8-Discover instrument) pattern of the synthesized
The size of Cu2(OH)3Cl structure was also evaluated; for this purpose, the peak width (W1/2) positioned at 2θ = 27° was selected and the crystalline size of the Cu2(OH)3Cl. This was estimated using Debye–Scherrer equation (Frost et al., 2002). On the basis of this equation, the average size of Cu2(OH)3Cl was calculated to be ∼150 nm, which is in good agreement with the high-resolution TEM (HR-TEM, Zeiss EM10) and FE-SEM images shown in Figure 11.
The formation of Cu2(OH)3Cl was also confirmed using X-ray photoelectron spectroscopy (XPS) analysis (Figure 12). In accordance with the XPS spectrum, the strong peaks, positioned at 952 and 933 eV, were related to the Cu 2P ½ and Cu 2P3/2, respectively, which agreed with those reported in the literature (Boita et al., 2014).
The X-ray fluorescence (XRF) was considered as another important and effective analytical spectroscopy that can be used to estimate the elemental percentages of Cu2(OH)3Cl. On the basis of the XRF analysis (XRF, Rigaku ZSX Primus II model, rhodium X-ray tube; 4.0-kW maximum power), the weight percentages of the elements presented in the analyzed structure are shown in Table 3. These results pointed to the accuracy of the structure of the analyzed sample during the Cl− removal process.
In addition, CHN-S-Cl elemental analysis was performed to know the molar ratio of elements. The results showed 3.61:2.89:1.03 ratios of H:O:Cl. Therefore, it was hypothesized that precipitation formula was Cu2(OH)3Cl, not Cu(OH)2(H2O)4 that was predicated with 5.21:3.12 ratios for H:O.
All these characterizations therefore revealed the catalytic property, as well as the nano-template effect of the synthesized nano-emulsion for selective synthesis of the
Evaluation of the Introduced Method
As explained before, the selectivity of this method was strongly evidenced on the basis of abovementioned documents that revealed high purity of the synthetic product. In addition, the acceptable removal efficiency and the lack of significant memory effect inside the nan-emulsion during its several reusability (at least four times) pointed to the sensitivity of this method. Simple and direct reaction process was also considered as other advantages of this process. Although, the main basis of the synthesized nano-emulsion was the commercial oil; nevertheless, the usability of this extractant strongly reveals the cost-effectiveness of this compound. However, the total price of this compound is not comparable with the economic value of the NGC, especially when resulting in synthesizing a suitable pesticide. Fast reaction time and non-significant toxicity (almost due to the viscose nature of the synthesized nano-emulsion) also made it suitable for such industrial applications.
Fortunately, this synthetic process was not comparable with the previous reports; as (to the best of knowledge) those methods were often suffered from problems such as 1) high cost, 2) small efficiency, 3) long synthetic time, and/or 4) hard conditions such as high temperature and pressure (Frost et al., 2002; Boita et al., 2014).
Conclusion
This report introduces an O/W nano-emulsion as the reusable extractant phase using LLE methodology for the removal efficiency of Cl− and Hg(0). The removal efficiency was estimated between 90% and ∼100%, deepening on the nature of the NGC at a brief separation time (<3.0 min). Consequently, serious impurities in condensate were removed by the scalable introduced methodology. In this method, the synthesized nano-emulsion as the extractant phase in the LLE methodology has significant advantages, especially 1) elemental mercury and chloride ion removal and 2) selective synthesis of the
Data Availability Statement
The original contributions presented in the study are included in the article/Supplementary Material, further inquiries can be directed to the corresponding author.
Ethics Statement
This studied was admitted and approved by the ethics committee of the Shiraz University Consul.
Author Contributions
MD directed the research group, supported the necessary methods, and edited the manuscript; ZBK consulted the project, analyzed the data, and edited the manuscript. She also performed all the chemical experiments, analyzed the data, and wrote the manuscript.
Funding
This study was financially supported by Shiraz University (grant no. 164116-131).
Conflict of Interest
The authors declare that the research was conducted in the absence of any commercial or financial relationships that could be construed as a potential conflict of interest.
Publisher’s Note
All claims expressed in this article are solely those of the authors and do not necessarily represent those of their affiliated organizations or those of the publisher, the editors, and the reviewers. Any product that may be evaluated in this article, or claim that may be made by its manufacturer, is not guaranteed or endorsed by the publisher.
Acknowledgments
The authors wish to acknowledge the support of this work by the Shiraz University Research Council.
Supplementary Material
The Supplementary Material for this article can be found online at https://www.frontiersin.org/articles/10.3389/fchem.2022.823357/full#supplementary-material
References
Abai, M., Atkins, M. P., Hassan, A., Holbrey, J. D., Kuah, Y., Nockemann, P., et al. (2015). An Ionic Liquid Process for Mercury Removal from Natural Gas. Dalton Trans. 44, 8617–8624. doi:10.1039/C4DT03273J
Argyle, M., and Bartholomew, C. (2015). Heterogeneous Catalyst Deactivation and Regeneration: A Review. Catalysts 5, 145–269. doi:10.3390/catal5010145
Banan Khorshid, Z., Mahdi Doroodmand, M., and Abdollahi, S. (2021). UV-vis. Spectrophotometric Method for Oil and Grease Determination in Water, Soil and Different Mediates Based on Emulsion. Microchemical J. 160, 105620. doi:10.1016/j.microc.2020.105620
Bingham, M. D. (1990). Field Detection and Implications of Mercury in Natural Gas. SPE Prod. Eng. 5, 120–124. doi:10.2118/19357-pa
Boita, J., do Carmo Martins Alves, M., and Morais, J. (2014). A Reaction Cell for Time-Resolvedin situXAS Studies during Wet Chemical Synthesis: the Cu2(OH)3Cl Case. J. Synchrotron Radiat. 21, 254–258. doi:10.1107/S1600577513028786
Brühl, C. A., Bakanov, N., Köthe, S., Eichler, L., Sorg, M., Hörren, T., et al. (2021). Direct Pesticide Exposure of Insects in Nature Conservation Areas in Germany. Scientific Rep. 11, 24144–24154.
Chen, H., and Zhong, Q. (2022). Physical and Antimicrobial Properties of Self-Emulsified Nanoemulsions Containing Three Synergistic Essential Oils. Int. J. Food Microbiol. 365, 109557. doi:10.1016/j.ijfoodmicro.2022.109557
D'Alfonso, C., Lanzalunga, O., Lapi, A., and Vadalà, R. (2014). Comparing the Catalytic Efficiency of Ring Substituted 1-hydroxybenzotriazoles as Laccase Mediators. Tetrahedron 70, 3049–3055. doi:10.1016/j.tet.2014.02.068
D’Acunzo, F., and Galli, C. (2003). First Evidence of Catalytic Mediation by Phenolic Compounds in the Laccase-Induced Oxidation of Lignin Models. Europ. J. Biochem. 270, 3634–3640.doi:10.1046/j.1432-1033.2003.03752.x
Ergun, M., and Turan, A. Y., Pitting Potential and protection Potential of Carbon Steel for Chloride Ion and the Effectiveness of Different Inhibiting Anions, Corrosion Sci. 32 (1991) 1137–1142. doi:10.1016/0010-938X(91)90098-A
FAO/WHO (2021). FAO/WHO Meeting on Pesticide Residues by the 2021 Meeting, 6-17 September; 4 and 7 October 2021.
Frost, R. L., Martens, W. N., Rintoul, L., Mahmutagic, E., and Kloprogge, J. T. (2002). Raman Spectroscopic Study of Azurite and Malachite at 298 and 77 K. J. Raman Spectrosc. 33, 252–259. doi:10.1002/jrs.848
Hong, R., Pan, T., Qian, J., and Li, H., Synthesis and Surface Modification of ZnO Nanoparticles, Chem. Eng. J. 119 (2006) 71–81. doi:10.1016/j.cej.2006.03.003
Hou, C. H., Huang, C. Y., and Hu, C. Y. (2013). Application of Capacitive Deionization Technology to the Removal of Sodium Chloride from Aqueous Solutions. Int. J. Environ. Sci. Technol. 10, 753–760. doi:10.1007/s13762-013-0232-1
Hussain, A., AlAjmi, M. F., Rehman, M. T., Amir, S., Husain, F. M., Alsalme, A., et al. (2019). Copper(II) Complexes as Potential Anticancer and Nonsteroidal Anti-inflammatory Agents: In Vitro and In Vivo Studies. Sci. Rep. 9, 5237–5254. doi:10.1038/s41598-019-41063-x
Jackson, K., and Fulton, J. L. (1996). Microemulsions in Supercritical Hydrochlorofluorocarbons. Langmuir 12, 5289–5295. doi:10.1021/la960210i
Jiang, X., Nešić, S., Kinsella, B., Brown, B., and Young, D. (2013). Electrochemical Investigation of the Role of Cl−on Localized Carbon Dioxide Corrosion Behavior of Mild Steel. Corrosion 69, 15–24. doi:10.5006/0620
Lavela, P., Macías, C., Ovín Ania, M. C., Rasines, G., Tirado, J. L., and Zafra, M. C. (2015). Mesoporous Carbon Black-Aerogel Composites with Optimized Properties for the Electro-Assisted Removal of Sodium Chloride from Brackish Water. J. Electroanal. Chem. 50, 42–50. doi:10.1016/j.jelechem.2015.01.016
Liu, D., Li, C., Wu, J., and Liu, Y. (2020). Novel Carbon-Based Sorbents for Elemental Mercury Removal from Gas Streams: A Review. Chem. Eng. J. 391, 123514. doi:10.1016/j.cej.2019.123514
Lu, L., Wang, R. L., Zhang, Z. J., Steward, F. A., Luo, X., and Liu, B. (2010). Effect of Dietary Supplementation with Copper Sulfate or Tribasic Copper Chloride on the Growth Performance, Liver Copper Concentrations of Broilers Fed in Floor Pens, and Stabilities of Vitamin E and Phytase in Feeds. Biol. Trace Elem. Res. 138, 181–189. doi:10.1007/s12011-010-8623-3
Lubej, A., Koloini, T., and Pohar, C. (2004). Industrial Precipitation of Cupric Hydroxy-Salts. Acta Chim. Slov. 51, 751–768.
Luo, X. G., Ji, F., Lin, Y. X., Steward, F. A., Lu, L., Liu, B., et al. (2005). Effects of Dietary Supplementation with Copper Sulfate or Tribasic Copper Chloride on Broiler Performance, Relative Copper Bioavailability, and Oxidation Stability of Vitamin E in Feed. Poult. Sci. 84, 888–893. doi:10.1093/ps/84.6.888
Milazzo, G., Caroli, S., and Braun, R. D. (1978). Tables of Standard Electrode Potentials. J. Electrochem. Soc. 125, 261C. doi:10.1149/1.2131790
Mohammadi, A., Doroodmand, M. M., and Sadegh, M. M. R. (2016). Selective Speciation of Cr(III) and Cr(VI) by Micro-emulsion Using UV-Vis. Spectrophotometry. J. Textile Sci. Eng. 6, 1–6. doi:10.4172/2165-8064.1000243
Pathania, R., Najda, A., Chawla, P., Kaushik, R., and Khan, M. A. (2022). Low-energy Assisted Sodium Alginate Stabilized Phyllanthus Niruri Extract Nanoemulsion: Characterization, In Vitro Antioxidant and Antimicrobial Application. Biotechnol. Rep. 33, e00711. doi:10.1016/j.btre.2022.e00711
Pierce, R. A., Campbell-Kelly, R. P., Visser, A. E., and Laurinat, J. E. (2007). Removal of Chloride from Acidic Solutions Using NO2. Ind. Eng. Chem. Res. 46, 2372–2376. doi:10.1021/ie061167p
Sabbaghi, S., Maleki, R., Shariaty-Niassar, M., Zerafat, M. M., and Nematollahi, M. M. (2012). Modeling of Chloride Ion Separation by Nanofiltration Using Machine Learning Techniques. Int. J. Nanosci. Nanotechnol. 8, 185–190.
Scott, D. A. (2000). A Review of Copper Chlorides and Related Salts in Bronze Corrosion and as Painting Pigments. Stud. Conservation 45, 39–53. doi:10.1179/sic.2000.45.1.39
Sun, W.-Q., Xu, X.-D., Zhang, Y., and Wu, J.-Z. (2019). Chlorine Corrosion of Blast Furnace Gas Pipelines: Analysis from thermal Perspective. J. Min Metall. B Metall. 55, 197–208. doi:10.2298/JMMB181016028S
Tanimizu, M., Takahashi, Y., and Nomura, M. (2007). Spectroscopic Study on the Anion Exchange Behavior of Cu Chloro-Complexes in HCl Solutions and its Implication to Cu Isotopic Fractionation. Geochem. J. 41, 291–295. doi:10.2343/geochemj.41.291
Wilhelm, S. M., Risk Analysis for Operation of Aluminum Heat Exchangers Contaminated by Mercury, Proc. Saf. prog. 28 (2009) 259–266. doi:10.1002/prs.10322
Wilhelm, S. M., and Bloom, N. (2000). Mercury in Petroleum. Fuel Process. Techn. 63, 1–27. doi:10.1016/S0378-3820(99)00068-5
Wu, X., Liu, Z., and Liu, X. (2013). Chloride Ion Removal from Zinc Sulfate Aqueous Solution by Electrochemical Method. Hydrometallurgy 134-135, 62–65. doi:10.1016/j.hydromet.2013.01.017
Yan, T. Y. (1987). Removal of Mercury from Natural Gas and Liquid Hydrocarbons Utilizing Downstream Guard Chabmer.
Yang, H., Xu, Z., Fan, M., Bland, A. E., and Judkins, R. R. (2007). Adsorbents for Capturing Mercury in Coal-Fired Boiler Flue Gas. J. Hazard. Mater. 146, 1–11. doi:10.1016/j.jhazmat.2007.04.113
Keywords: natural gas condensate, mercury and chloride impurities, nano-emulsion, dicopper chloride trihydroxide, catalytic mediator, agricultural pesticide
Citation: Banan Khorshid Z and Doroodmand MM (2022) Reusable Extractant and Direct Catalytic Mediation of Water/Oil/Chlorodifluoromethane Nano-Emulsion in Natural Gas Condensate for Efficient Conversion of Chloride Impurities Into the Dicopper Chloride Trihydroxide Nanoparticles. Front. Chem. 10:823357. doi: 10.3389/fchem.2022.823357
Received: 27 November 2021; Accepted: 21 February 2022;
Published: 26 April 2022.
Edited by:
Vinoth Kumar Ponnusamy, Kaohsiung Medical University, TaiwanReviewed by:
Md. Mominul Islam, University of Dhaka, BangladeshAminul Islam, Jashore University of Science and Technology, Bangladesh
Copyright © 2022 Banan Khorshid and Doroodmand. This is an open-access article distributed under the terms of the Creative Commons Attribution License (CC BY). The use, distribution or reproduction in other forums is permitted, provided the original author(s) and the copyright owner(s) are credited and that the original publication in this journal is cited, in accordance with accepted academic practice. No use, distribution or reproduction is permitted which does not comply with these terms.
*Correspondence: Mohammad Mahdi Doroodmand, ZG9yb29kbWFuZEBzaGlyYXp1LmFjLmly