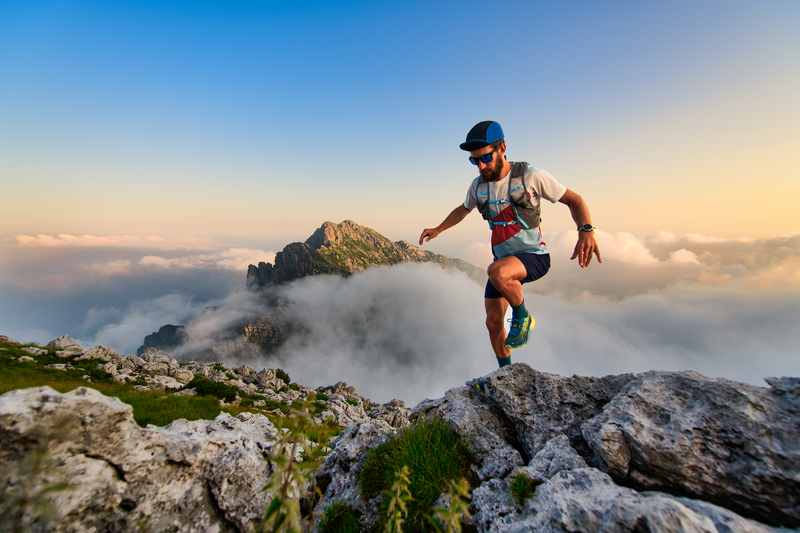
95% of researchers rate our articles as excellent or good
Learn more about the work of our research integrity team to safeguard the quality of each article we publish.
Find out more
REVIEW article
Front. Chem. , 04 March 2022
Sec. Green and Sustainable Chemistry
Volume 10 - 2022 | https://doi.org/10.3389/fchem.2022.823005
This article is part of the Research Topic Reviews in Chemistry View all 14 articles
The second (lignocellulosic biomass and industrial wastes) and third (algal biomass) generation feedstocks gained substantial interest as a source of various value-added chemicals, produced by fermentation. Lactic acid is a valuable platform chemical with both traditional and newer applications in many industries. The successful fractionation, separation, and hydrolysis of lignocellulosic biomass result in sugars’ rich raw material for lactic acid fermentation. This review paper aims to summarize the investigations and progress in the last 5 years in lactic acid production from inexpensive and renewable resources. Different aspects are discussed—the type of raw materials, pretreatment and detoxification methods, lactic acid-producers (bacteria, fungi, and yeasts), use of genetically manipulated microorganisms, separation techniques, different approaches of process organization, as well as main challenges, and possible solutions for process optimization.
The intensive economic growth during the past century is accompanied by high energy consumption. Nowadays fossil fuels (coil, petroleum, and natural gas) are still the main energy source and raw material for the production of various chemicals. The fossil fuels were formed and stored underground for millions of years and their extensive use has led to the situation where the present vegetation on Earth cannot treat the emitted carbon dioxide by photosynthesis (Damyanova and Beschkov 2020). As a consequence, the strong emissions of carbon dioxide and greenhouse gases affect and change the climate. One of the ways to cope with this global problem is to close the natural carbon cycle using renewable sources as a platform for biofuels and chemicals production, and thus enabling recycling of the biological sources and consumption of the resulting carbon dioxide by photosynthesis (Beschkov et al., 2020).
A major step in the development of a sustainable, industrial society will be the shift from dependence on oil to the use of renewable resources. In the prospect of environmental sustainability, the utilization of agro-industrial waste residues as feedstock for the production of both biofuels and basic synthetic chemicals in biorefineries has gained widespread attention (Yaashikaa et al., 2022). In the long run, oil use can be eliminated and gas emissions reduced. The market share of biotechnological processes for the production of various chemical products is expected to increase in the coming years from 5 to 20%. Organic acids are a key group of chemicals that can be produced microbiologically. Functional groups that need to be introduced through an expensive multistage oil oxidation process are present in plant materials such as carbohydrates.
Cellulosic biomass is an abundant and sustainable source of valuable chemicals that are truly unique for making various organic products. It includes agricultural wastes (corn stover, spent grains, and sugarcane bagasse), some municipal solid wastes (waste paper), wastes from forestry residues (mill wastes and sawdust), herbaceous (switchgrass), and woody (poplar trees) crops. Such materials are plentiful and accessible in many regions of the world and can be competitive in price with petroleum and thus opening up a new route to manufacturing organic fuels and chemicals. Despite the high availability, the degradation of biomass is a substantial challenge. Hence, it is necessary to integrate several unit processes such as biochemical, thermochemical, physical, and catalytic conversion to produce a wide range of bio-based products (Velvizhi et al., 2022).
Lgnocellulosic biomass is composed of cellulose linear chains formed from glucose units. Cellulose fibers are held together with hemicellulose (branched heteropolymer formed by various hexoses and pentoses) and lignin (complex heteropolymer built from phenylpropanoid units). The specific structure of lignocellulose restricts the access of enzymes to cellulose and hemicellulose fibers and hinders effective hydrolysis. In view to converting lignocellulose to valuable chemicals by fermentation, this structure must be destructed by some pretreatment. The purpose of the pretreatment is to decrease the crystallinity of cellulose and degree of polymerization, destruct hydrogen bonds between fibers, and thus increase the area accessible for the enzymes’ action. The lignin should be at least partially removed and cellulose and hemicellulose efficiently hydrolyzed to fermentable sugars. Various pretreatment techniques have been used with the goal of breakdown the lignocellulosic complex and liberating sugars for further use.
Lignocellulosic biomass of various sources can be used for the production of different chemicals such as fuels (ethanol, methane, hydrogen), enzymes (cellulases, amylase, protease), high-value chemicals (hydroxycinnamic acid, lactic acid, xylitol), etc.
The present review aims to summarize in-depth the latest achievements in the field of fermentative lactic acid production from renewable sources –various substrates, microorganisms including gene manipulated, as well as process organization and downstream techniques.
Lactic (2-hydroxypropanoic) acid (LA) is a valuable platform chemical with both traditional and newer applications (Figure 1). It was widely used as a neutralizer, preservative, or acidulant in food and beverage, cosmetic, pharmaceutical, and other industries. Recently, lactic acid is applied as the building block for various biodegradable polymers or precursor for environmentally friendly solvents. The annual global market of lactic acid in 2020 is valued at USD 1.1 billion with an increasing tendency to double till 2025 (Lactic Acid Market by Application (Biodegradable Polymers, Food & Beverages, Pharmaceutical Products) 2020). The global market for lactic acid was 750.00 kilotons in 2017 and is projected to reach 1,845.00 kilotons by 2022 (Global Lactic Acid Market 2017-2025 - Growth Trends, Key Players, Competitive Strategies and Forecasts - Research and Market 2017).
FIGURE 1. Applications of lactic acid in different industries (Newer application are given in italic).
Nowadays, lactic acid is produced by chemical or fermentation way. In the chemical route (Figure 2A), lactic acid is produced from petrochemical sources by a multistep reaction scheme. It includes catalytic oxidation of ethylene, conversion of obtained acetaldehyde to lactonytrile end its hydrolysis to a racemic mixture of D (-) and L (+) lactic acid. Of course, there are other methods for the chemical synthesis of lactic acid like oxidation of propylene or propylene glycol, hydrolysis of chloropropionic acid, but they are not economically and technically feasible (Krishna et al., 2018). Fermentative production of lactic acid is based on the conversion of different sugars (glucose, lactose), starchy or lignocellulosic hydrolysates by different microorganisms, mainly lactobacilli (Figure 2B). Factors affecting lactic acid fermentation (pH, temperature, nutrients, substrate, product concentrations, etc.) are discussed in the review of Rawoof et al. (Rawoof et al., 2021).
- pH—for most lactobacilli the optimum value for effective lactic acid production is between 5 and 7. Unfortunately, accumulation of the lactic acid leads to decreasing pH, even outside the optimum value, leading to a decrease in microorganisms’ growth and lactic acid production. Additionally, high lactic acid concentration inhibits cellular metabolism. A decrease in pH could be overcome by neutralization with strong bases like sodium, ammonium, or calcium hydroxide, or low molecular mass water-soluble amines. Another way is in-situ product removal during fermentation. Discovering or engineering the acid-tolerant strains is a challenge. This will permit to decrease the product inhibition, and overall cost of the process, eliminating the use of neutralizers.
- Temperature—the temperature is an important factor for bacterial growth and lactic acid production. Most of the lactobacilli are mesophilic (25–40°C). An increase in the operational temperature will result in decreasing contamination and increasing substrate hydrolysis. Very often, the optimal temperature for microbial growth differs from that for maximal lactic acid production. The selection of strains capable to grow at thermophilic (40–65°C) or extreme thermophilic (65–80°C) conditions will be very favorable.
- Media composition—the complexity of the fermentation media (carbon and nitrogen sources, minerals, vitamins) is a factor increasing the cost of lactic acid production. Carbon is an indispensable element and the replacement of costly individual sugars with cheap agricultural and industrial wastes is a step towards decreasing the total cost. Nitrogen is a major component in the anabolic and catabolic processes. Usually, complex nitrogen sources like peptone, yeast extract, and meat extract are used. Alternatives for cheap nitrogen sources are corn steep liquor, hydrolysate of fish waste, and wheat bran extract. DDGS (about 30% protein) is an attractive alternative because its hydrolysis results in carbon sources and nitrogen at a time. The ratio between carbon and nitrogen (C/N) also is very important. An optimal C/N ratio results in a positive effect on lactic acid fermentation. Usually, the optimal C/N ratio is between 3 and 7 (Gómez-Gómez et al., 2015), (Gilver Rosero Chasoy et al., 2020), (Mejia-Gomez and Balcazar 2020).
- Sugars concentration—at a high initial substrate concentration some problems such as longer lag phase, decreased activity, cellular lysis, and osmotic stress could occur, resulting in reduced substrate consumption and low lactic acid production. The effect of substrate inhibition can be diminished by adding osmo-protective substances, fed-batch fermentation mode, developing high sugars tolerant strains. Substrates originating from lignocellulosic sources usually are a mix of pentoses and hexoses. Lactic acid bacteria possess a hierarchical pattern of sugar utilization—glucose is utilized first and then other sugars. This leads to carbon catabolite repression, a decrease in fermentation efficiency, and low product concentration. The problem can be solved by using pentoses and hexoses utilizing strains in co-fermentation mode. Another way is isolating or engineering strains with increased carbon catabolic repression resistance.
- Effect of end- and by-product accumulation—the effect of product accumulation and subsequences were discussed above. Glucose and xylose are the main conctituents of agrocellulosic hydrolisates. While glucose undergoes conversion to lactic acid mainly by homo-fermentation, xylose is converted by the hetero-fermentative pathway, leading to the formation of by-products like ethanol and acetic acid. The accumulation of by-products leads to a decrease in lactic acid yield and an increase in the cost of product recovery. Again, the selection of novel strains and genetically modifying the existing strains to follow the desired pathway is the possible solution to the problem.
- Sensitivity to toxic compounds—most of the pretreatment methods lead to the formation of inhibitory compounds—furfural, 5-hydroxymethyl furfural, levulinic acid, formic acid, p-coumaric acid, ferulic acid, etc. These compounds exhibit an inhibitory effect on the enzyme’s action, cellular growth, and lactic acid production. Different approaches can be used for elimination of inhibitory effect—use of pretreatment methods leading to less liberation of inhibitors, chemical or biological neutralization of the inhibitors, use of adaptive evaluated, inhibitor-tolerant, or engineered strains.
Some bottlenecks hinder effective lactic acid production. The major one is product inhibition. Accumulation of the lactic acid leads to a fast drop in the medium pH, outside of the optimum value for the producer strain. This results in low substrate conversion and low final product concentration. The problem may be overcome either by in situ product removal or by using acid-tolerant bacteria. The cost of raw material and nutritional additives are with the major contribution to the overall cost of lactic acid. Usually, the pure sugars used as a substrate for lactic acid fermentation are very costly and take part in the human food chain. According to S. Tejayadi and M. Cheryan in the case of lactic acid production from whey permeate, the cost of whey (including transportation) was 35% and for yeast extract—38% of the total cost (Tejayadi and Cheryan 2005). Akerberg and Zacchi reported that the major operational costs (raw material, saccharification, fermentation, and electrodialysis) contributed about 80% to the total cost in case of lactic acid production from wheat flour (Akerberg and Zacchi 2000). For using starch or other natural polymers, like inulin, it is necessary either to hydrolyze it to individual sugars or use lactic acid-producing bacteria with relevant enzyme activity. In recent years, a new process for lactic acid production attract the interest of researchers in the field—catalytic conversion of lignocellulosic biomass. The process is led in the presence of homogeneous and heterogeneous catalysts at lower temperatures or under subcritical conditions. For example, lactic acid production from empty fruit palm oil bunch under hydrothermal conditions in the presence of metal salts was described (Sitompul et al., 2014) and (Chin et al., 2016). In an extensive and comprehensive review, Mäki-Arvela et al. (2014) describe the research since 2000 on the production of lactic acid from biomass and its transformation to commodity chemicals. The mechanisms in the production of lactic acid and its derivatives in the presence of homogeneous and heterogeneous catalysts were described, as well as reaction conditions, catalysts’ properties, stability, and reuse. The authors also discussed some reactor technologies and kinetic modeling of the processes in hydrogenation and esterification of lactic acid.
In any case, it is clear that finding a cheap, abundant, and easily accessible row material is a key factor for an effective and economically profitable method for fermentative lactic acid production. Lignocellulosic biomass especially forest biomass, waste, and inedible plant materials, as well as various agricultural crop residues, seems to be a promising substrate for an effective and economically profitable method for fermentative lactic acid production which non-competes with the human food chain. Recently, several review papers concerning lignocellulosic biomass utilization as a source for bio-based chemicals production have been published. (Tišma et al., 2021; Banu et al., 2021; Haldar and Purkait 2020; Usmani et al., 2021; Nwamba et al., 2021).
Regarding lactic acid production from lignocellulosic biomass, there are some reviews published in the last 2 years considering different aspects of the issue—microorganisms (Abedi and Hashemi 2020), sources (Lopez-Gomez et al., 2020; Ajala et al., 2020), pretreatment methods (Huang et al., 2021; Mankar et al., 2021), separation (De Oliveira et al., 2020; Li et al., 2021), techno-economic analysis (Li et al., 2021; Manandhar and Shah 2020; Daful and Görgen 2017), etc. Thygesen et al. (2021) summarized recent data on the valorization of municipal organic waste into purified lactic acid - pretreatment, enzymatic hydrolysis, LA fermentation, and downstream processing.
In every step of lactic acid production by fermentation from lignocellulosic feedstocks, from pretreatment to separation, there are various bottlenecks, which must be overcome for the realization of an effective and low-cost process.
Regardless of the undoubted advantages of using lignocellulosic biomass as raw material for lactic acid production, like low cost and global availability, main problems can be summarized as follows:
- in pretreatment step—-i) resistant nature of biomass and difficulties in full separation of lignin from cellulose and hemicellulose; ii) energy consumption, toxicity, and environmental problems of some used chemicals; iii) release of inhibitory compounds; iv) high cost of hydrolytic enzymes and their product inhibition.
- in fermentation step—i) nutritional requirements of lactic acid bacteria; ii) carbon catabolite repression; iii) product and substrate inhibition; iv) by-product formation in hetero-fermentation.
- in separation and purification step—i) low concentration of feeding stream; ii) more complex composition of fermentation broth in comparison with first-generation LA production; iii) high cost of downstream processes; iv) not all separation methods are tested on second-generation LA; v) ecological problems connected with classical precipitation separation.
More details for different problems and possible solutions are given in (Cubas-Cano et al., 2018; Tan et al., 2018; Grewal et al., 2020).
The term biomass generally includes the mass of organic material in all living organisms on Earth (microorganisms, algae, plants, and animals). The main building elements of biomass are carbon, hydrogen, oxygen, and nitrogen, and similarly to fossil feedstocks, the biomass can be used for various chemicals production. Biomass is abundant and renewable and can be divided into three groups (Figure 3). Primary resources are produced directly by photosynthesis and taken from the land; secondary resources result from the processing of primary sources and tertiary—post-consumers residue streams. The greater part of plant biomass consists of lignocellulose—a complex of three polymers (cellulose, hemicellulose, and lignin) and a small number of extractives, pectin, and ash. A sketch of lignocellulose structure is given in Figure 4.
Cellulose is a linear polysaccharide comprising d-glucose units, linked by β (1–4)-glycoside bonds (in contrast to α (1–4) bonds in starch amylose). Cellulosic molecules are located in parallel, forming primary fibrils, stabilized by hydrogen bonds. The sections with a strictly parallel arrangement and additional hydrogen bonding, are referred to as crystalline, in contrast, to those in which such arrangement is missing. The latter are called amorphous. The cellulose fibrils can further interact to form cellulose fibers. The cellulose is very stable and insoluble in water, diluted acids, and the majority of common solvents, but is soluble in concentrated acids, and alkali bases (for DP smaller than 100).
Hemicellulose is a branched polymer built from different saccharides—pentoses (xylose, arabinose), hexoses (glucose, mannose, galactose) as well as some uronic acids (glucuronic, galacturonic acid). For example, the main components in softwood hemicelluloses are built from hexoses and those of hardwood from pentoses. The polymerization degree of hemicelluloses is smaller than in cellulose. Hemicellulosic polysaccharides are soluble in bases (10% KON or 18% NaOH), dimethylsulphoxide, and in rare cases in hot water.
Lignin is a highly branched amorphous phenolic polymer composed mainly of p-coumarin alcohol (H unit), coniferyl alcohol (G unit), and sinapyl alcohol (S unit). Lignin is a highly cross-linked polymer with long chains. The structure of lignin differs considerably from its source (type of plant, and even different parts of the same plant), for example, the lignin, derived from softwood is built predominantly of G units, hardwood lignin is mostly comprised of G and S units, while lignin from grass contains all three units.
Cellulose fibers are stacked together by hydrogen bonds with hemicellulose and pectin. Lignin interacts with cellulose and hemicellulose not only by hydrogen bonding but with covalent bonds and thus forming a rigid complex structure difficult for chemical or biochemical degradation.
Lignocellulosic biomass differs considerably depending on its origin. In general, the composition is cellulose 30–60%, hemicellulose 20–40%, and lignin 15–25% (Norrrahim et al., 2021). Reshmy et al. (2022) discussed the selection of lignocellulosic sources, advanced pretreatment methods for various types of cellulose for use in cost-effective bio-refineries for future industrial application. A short description of major platform chemicals and biomaterials as well as different biofuels such as biomethane, bioethanol, and biohydrogen, is given. In Table 1 data for different lignocellulosic feedstocks composition are summarized.
In what follows a brief data for the most used lignocellulosic feedstocks are given.
The world corn production for 2020/2021 is estimated at around 1.125 billion metric tons (Shahbandeh, 2021a). It is estimated that for each bushel of shelled corn 50 pounds of corn residue (cobs, leaves, stalks, and husks) are also produced (McCutcheon and Samples 2002). It means that an enormous amount of corn residues are available for converting into value-added products.
Brewer’s spent grains (BSG) are the major by-product in the brewing industry. The quantity of residue material in beer production is about 24.4 kg per 100 L of beer produced (about 85% of total generated by-products are brewer’s spent grains or approximately 20 kg per 100 L of beer (Reinold 1997)). The worldwide beer production in the last decade is around 1.93 billion hectoliters (Conway 2021).
Sugarcane is a perennial grass mainly used for sugar production. Annually around 1,89 billion tonnes of sugarcane are produced worldwide and 250–270 kg of sugarcane bagasse are generated from each ton of sugarcane (Wischral et al., 2019).
Coffee is surely one of the most popular beverages consumed in the world. The volume of world coffee production in 2019/2020 is estimated to be 163.7 million 60-kg bags (Shahbandeh, 2021b). Approximately half (in dry weight basses) of the coffee is separated in the form of spent coffee grounds during the preparation of coffee beverages or the manufacturing of instant coffee or instant coffee-making process (Kim et al., 2019). According to (Mussatto et al., 2011) 650 kg of Spent Coffee Grounds (SCG) are generated from each ton of green coffee.
Distillery’s Dried Grains With Solubles (DDGS) represent the most important by-product in bioethanol production. After ethanol distillation, the thin stillage from the fermentation broth was decanted, and the solid is dried to produce DDGS. The world bioethanol production (from sugarcane, wheat, corn, and other feedstocks) in 2020 was estimated at around 100 billion liters (Bio-ethanol market-growth, trends, covid-19 impact, and forecasts (2021 - 2026) 2021). The production of the first generation of ethanol is almost strictly differentiated by countries (mainly from corn in the United States and sugarcane in Brazil). Taking into account that from bushel (25.4 kg) grains about 8.2 kg of ethanol is produced, releasing about 7.7 kg of DDGS (Jacques et al., 2003) it is easy to calculate that about 73 million of tonnes DDGS are produced annually.
From the data presented above, it is seen that lignocellulosic sources are released in enormous quantities and can be used as a substrate for fermentative production of various value-added chemicals after individual sugars liberating.
The specific structure of the lignocellulose restricts the access of enzymes to cellulose and hemicellulose fibers and hinders effective hydrolysis. In view to converting lignocellulose to fermentable sugars, the lignocellulosic biomass must be subjected to some pretreatment. The purpose of the pretreatment is to break down the structure of lignocellulose, destruct hydrogen bonds between fibers, remove at least partially the lignin, decrease the crystallinity of cellulose, and degree of polymerization, and thus increase the area accessible for the enzymes’ action, as well as efficiently hydrolyze cellulose and hemicellulose. Different methods have been applied for the pretreatment of lignocellulosic biomass (Norrrahim et al., 2021). In general, they can be classified into four main groups—physical, chemical, physicochemical, and biological methods (Figure 5). When the main goal of lignocellulosic biomass processing is obtaining maximum fermentable sugars as a substrate for fermentative production of value-added products, pretreatment is an inevitable step. Usually, each method results in different effects (delignification, hemicellulose dissolution, etc.). The most important is that there is no universal method for lignocellulosic biomass pretreatment. This is due to the different compositions of lignocellulosic biomass (ratio of main components and degree of crystallinity of cellulose) from different sources (soft- and hard-wood, agricultural, or industrial wastes). The compositional differences influence the efficacy of pretreatment. The severity of treatment (pressure, temperature, and acid or alkali concentration) also lead to differences in the composition of obtained hydrolysates as well as in the concentration of inhibitors of enzyme hydrolysis and fermentation. Recently Zhao et al. (2022) summarized the current state and the latest advances of lignocellulose pretreatment technologies and described several new strategies for overcoming pretreatment bottlenecks for the realization of highly efficient lignocellulose bioconversion.
In view to choosing an “optimal” pretreatment method, one needs broad knowledge about the chemical composition of the feedstock, but also the final aim of pretreatment. For example, in case the fermentation step is done by an industrial strain non-converting pentoses—lignin, and hemicellulose should be separated from biomass before cellulose hydrolysis. In the case of using a strain capable to convert pentoses or co-fermentation removal of lignin should be enough. Usually, a combination of two or more methods is used before the enzymatic hydrolysis of lignocellulosic biomass.
The main purpose of these methods is to reduce the particle size of biomass, the crystallinity of cellulose, and the degree of polymerization of cellulose and hemicellulose and thus increasing surface area accessible for enzymes and biomass biodegradability. Physical methods do not use any chemicals and include different types of chipping, milling, and grinding, as well as gamma- or microwave irradiation, and ultrasound treatment. The main disadvantage of physical methods is high energy requirements, especially on a large scale.
This group includes several methods, based on reactions between lignocellulose biomass and various chemicals in the water phase. This is the most applied group of methods and its main goal is to reduce the amount of lignin and hemicellulose content in the biomass.
These methods are relatively cheap and easy to perform. Acid hydrolysis of biomass can be done using diluted (0.5–5%) or concentrated (10–60%) acids. Sulphuric and hydrochloric acids are most employed. Smits et al. (2019) developed a fast method for screening of optimal acid-pretreatment conditions in the conversion of wood lignocellulose to sugar. Luo et al. (2021) developed an artificial neural network model to simultaneously predict the derived feature of phenolic compounds content and glucose yield in lignocellulosic biomass hydrolysate from dilute inorganic acid pretreatment and enzymatic hydrolysis. Also, there are attempts to use some organic acids to avoid problems with the corrosive action of the acids. While hydrolysis with concentrated acids can be realized at low temperature and normal pressure, dilute acid hydrolysis require elevated temperatures and pressure.
Hydroxides like NaOH, KOH, NH4OH, Ca(OH)2. Hydroxides are less corrosive in comparison to acids and can be applied at moderate temperatures and ambient pressure when the alkali concentration is about 10–20%. At low concentrations higher temperatures and elevated pressure are necessary. These methods are considered better in deligninfication compared to acid hydrolysis methods, whereas cellulose remains mostly intact, and hemicellulose is affected depending on the severity of treatment.
Another successful method for lignin separation is so-called solvolysis. This technique uses some low boiling alcohols (methanol, ethanol) or high boiling alcohols, like glycerol, ethylene glycol, and butanol. Some ketones—acetone, methyl isobutyl ketone, and organic acids (formic, acetic, oxalic) are also utilized. Methods applying low boiling chemicals require high temperatures (150–250°C) and pressure. After solvolysis pretreatment, the lignocellulosic biomass separates into three fractions—precipitated lignin, solid cellulosic pulp, and liquid phase, containing hemicellulosic sugars (Goh et al., 2011). Sidiras et al. (2022) reviewed organosolv pretreatment methods and the optimization of feedstock delignification, sugars production, enzymatic digestibility of the cellulose fraction, and quality of lignin.
Oxidative pretreatment methods involve biomass processing with air, pure oxygen, hydrogen peroxide, or ozone in the water phase. In the case of ozone, the process can be conducted at ambient conditions, while other oxidizers demand high temperatures (150–250°C) and pressure—10–20 bar for short periods—10–15 min. During pretreatment, the lignin is converted to carboxylic acids, carbon dioxide, and water. The presents of acids in the solution facilitate the solubilization of the hemicellulose and increase the cellulose content.
In the last 20 years, the application of ionic liquids (IL) and deep eutectic solvents (DES) in biomass pretreatment gains more and more the attention of researchers. Ionic liquids are composed of a large organic cation and a small anion. They are liquid at room temperature with low vapor pressure, which facilitates their almost full recovery and thus somehow overcomes the main drawback of using IL—their high cost. Deep eutectic solvents are easily prepared by heating (60–80°C, for 4–8 h) a hydrogen bond donor (urea, acetamide, glycerol, some acids—lactic, oxalic, and sugars—glucose, maltose) and a hydrogen bond acceptor (different quaternary ammonium salts—choline chloride, tetramethylammonium chloride, etc.). The compounds forming DES are linked through hydrogen bonds and the formed eutectic mixture has a lower melting temperature than the initial components. The main advantages of DES are their ease of synthesis, biodegradability, and low cost. It is accepted that IL and DES are more effective in the separation of lignin and hemicellulose than acid and alkali treatment. Xu et al. (2021) analyzed the influence of different hydrogen bond donors on the lignocellulosic biomass pretreatment with choline chloride-based DES. The authors defined the most important among 42 key process factors—HBD hydrophilic ability, HBD polarity, HBD acidity, HBD ability to form hydrogen bonds, the molar ratio of HBD to choline chloride, and pretreatment severity (Xu et al., 2021). Yadav et al. (2020) have screened some lactic acid bacteria for their stability in ionic liquids. Recent reviews published by Usmani et al. (2020) and Halder et al. (2019) describe recent developments in using techniques applying ionic liquids in biomass pretreatment—breakdown mechanism, process parameters, the impact of cation and anion groups, and the advantages of IL on the further processing of the fractionated biomass.
These methods combine physical forces with the action of chemicals to modify the lignocellulosic biomass structure. Steam explosion, ammonia fiber expansion (AFEX), and supercritical fluids treatment take part in this group. The treatment can be done with pure water or water steam at elevated pressure and temperatures (hydrothermal treatment) or in the presence of some chemicals acting as a catalyst. Depending on temperature and pressure (bellow or above the critical point of the solvent) methods can be divided into two groups—subcritical and supercritical. As a result of physicochemical pretreatment dissolution of hemicellulose, changes in lignin structure and decreasing degree of polymerization and cellulose crystallinity and are observed. Duque et al. (2016) described the fundamentals of steam explosion methods, their advantages and disadvantage, and technological advancements made in the last years.
In this method the biomass is subject to high temperature (150–250°C) for a short time (from certain seconds to a few minutes) in the presence of saturated steam, followed by a quick release of the pressure. Sometimes a two-step method is applied—in the first step, the biomass is heated to 180°C in view to solubilize and remove hemicellulose, and then the temperature is elevated to 240°C to modify cellulose and lignin. The method is environmentally friendly with advantages like low energy consumption and low cost. The drawback is the formation of some fermentation inhibitors.
Similarly to steam explosion the biomass is heated to 150–250°C at pressure 3–5 MPa, but without quick decompression, which permits to realize the process in a flow-through reactor. The method is cost-effective, does not use toxic chemicals, and is possible to control the release of inhibitors by controlling pH values between 4 and 7.
The method uses liquid or gaseous ammonia (usually at 1:1 ratio) under high pressure (15–30 bar) and moderate temperatures (60–100°C) for 5–10 min. The moderate temperatures, as well as the possibility of recovering and reuse of the ammonia, reduce the cost of the process. The method is not very effective in treating biomass with high lignin content (higher the 20%) and also does not fully dissolute the hemicellulose. Recently, a new method called extractive ammonia is investigated. It is characterized by a higher ratio of ammonia to biomass (up to 6:1). The method is very effective in lignin removal (about 45% in corn stover) leaving almost all carbohydrates (more than 90%) in the solid phase (Avci et al., 2019).
Supercritical fluids are characterized by their unique properties—they have a density like a liquid and viscosity and diffusivity like gases. Carbon dioxide is the most widely used chemical in supercritical conditions. While the biomass pretreatment with sec carbon dioxide is not enough effective, the presence of moisture ameliorates changes in the biomass structure significantly. In the presence of moisture, CO2 forms carbonic acid which augments hemicellulose hydrolysis. Supercritical CO2 can be used also with co-solvents (methanol or ethanol) and thus enhance digestibility. The main advantages of this method are low cost, easy recovery of CO2, no release of toxic compounds, non-inflammability of CO2, and reduced environmental impact. Supercritical water is also used for biomass pretreatment. In contrast to supercritical CO2, in the case of water, much higher temperatures and pressures are applied. Short reaction times (1–2 s) result in high sugars yield, whereas long reaction times decrease the yield.
These methods involve the use of enzymes or enzymatic systems of whole microorganisms (mainly fungi and bacteria. Depending on their goal, the methods can be divided into two groups—delignification and saccharification methods. Fungi (white-, brown and soft-rot) possess in their enzymatic system enzymes like lignin peroxidases, manganese peroxidases, laccases, glyoxal, and alcohol oxidases and are capable to degrade lignin. Some fungi degrade selectively lignin (mainly white-rot), while others attack also cellulose and hemicellulose (Rouches et al., 2016). Except for fungi, there are many bacterial strains (Bacillus sp., Streptomyces sp., Clostridium sp., Thermomonospora sp., Cellulomonas sp., etc.), producing enzymes capable to degrade various lignocellulosic biomasses (Sharma et al., 2019). Besides using an individual strain in biomass pretreatment, very often consortia of different microorganisms are applied. Working with consortia can provide process robustness, higher stability, and productivity of the treatment. Recently, the application of lignin-degrading enzymes instead of using whole organisms gains considerable interest among researchers. The most utilized enzymes belong to cellulases, ligninases, and xylanases. Different enzymes like laccase, versatile peroxidase, manganese peroxidase, and lignin peroxidase are effective in lignin degradation (Kumar and Chandra 2020). Cellulases are multienzyme complexes comprising mainly three components: endo- β 1–4—glucanase, exo- β 1–4—glucanase, and β-glucosidase. Endo- β 1–4—glucanase hydrolyzes internal links in cellulose chains to short-chain cellodextrins. Exo- β 1–4—glucanase cleaves cellobiose from the non-reducing end of the chains, and β-glycosidase hydrolyzes cellobiose units to glucose units (Shuddhodana et al., 2016). Regarding the heteropolysaccharide structure of hemicellulose, different enzymes are needed to hydrolyze it to monomeric sugars. Endo-xylanases and endo-mannanases randomly attack the inner bonds in xylan and mannan, while β-xylosidase and β-mannosidase hydrolyze oligosaccharides to xylose and mannose. For hydrolysis of the lateral groups (arabinose, acetyl, galactose, and glucose) linked to the main polysaccharide chain supplementary enzymes are necessary—α-glucuronidase, acetyl xylan esterase, acetyl mannan esterase, ferulic acid esterase, etc. (Laca et al., 2019).
Biological methods of lignocellulosic materials degradation have advantages, such as low energy cost, environmental friendliness, low capital investment cost, no reagent dependence, and no production of fermentation inhibitors. Still, long reaction time and low reaction rate, and the relatively high price of the enzymes are the main limitations, preventing the successful implementation of the biological methods in the industry.
Recent trends in lignocellulosic biomass pretreatment methods, their advantages, and disadvantages and the role of various key factors that affect the separation of lignocellulose constituents are discussed in Baruah et al. (2018) and Mankar et al. (2021). It is worth mentioning that the use of only one pretreatment method is not effective and usually a combination of two or more methods are applied. It seems that the use of a mechanical method for reducing the size of the biomass particles and enzyme hydrolysis of hemicellulose and cellulose to fermentable sugars is inevitable.
The most important is that there is no universal method (or combination of methods) for lignocellulosic biomass pretreatment. This is due to the different compositions of lignocellulosic biomass (ratio of main components and degree of crystallinity of cellulose) from different sources (soft and hardwood, agricultural or industrial wastes). The compositional differences influence the efficacy of pretreatment. The severity of treatment (pressure, temperature, and acid or alkali concentration) also lead to differences in the composition of obtained hydrolysates as well as in the concentration of inhibitors of enzyme hydrolysis and fermentation. In view to choosing an “optimal” pretreatment method, one needs broad knowledge about the chemical composition of the feedstock, but also the final aim of pretreatment. For example, in case the fermentation step is done by a strain not converting pentoses—lignin and hemicellulose should be separated from biomass before enzyme hydrolysis. In case of using a strain capable to convert pentoses or co-fermentation removal of lignin should be enough. In general, an “ideal” lignocellulose pretreatment method should provide 1) full degradation and removal of the lignin presented; 2) utterly separation of cellulose and hemicellulose; 3) possibility of complete liberation of fermentative mono sugars; 4) minimal or no release of fermentation inhibitory compounds.
Recently Shen and Sun described three strategies for lignocellulosic biomass pretreatment, depending on the final goal, namely cellulose-first, hemicellulose-first, and lignin-first strategies together with most appropriated methods. These strategies possess unique advantages, permitting a component of lignocellulose to be selectively fractioned for value-added application (Shen and Sun 2021).
Table 2 summarizes the advantages and disadvantages of different pretreatment methods and Table 3—the methods used in lignocellulosic biomass pretreatment for lactic acid production.
As was mentioned above, the majority of pretreatment methods generated some products that can hinder subsequent hydrolysis and fermentation. The inhibitory compounds are a result of the degradation of lignin and sugars from cellulose and hemicellulose and represent different carboxylic acids and also furanic and phenolic compounds. Hexoses from cellulose and hemicellulose can be transformed during pretreatment to 5-hydroxymethyl furfural (HMF), which further dehydration leads to levulinic and formic acid formation. Various inhibitory products like furan aldehydes, sugar acids, and aliphatic acids are produced upon hemicellulose pretreatment. Acetic, formic, acrylic, and levulinic acids are detected in hemicellulose hydrolysates, but furfural is the most strong and abundant inhibitor. Various phenolic compounds are liberated during lignin pretreatment. The concentration and type of these compounds depend on biomass source and H/G/S group ratio in the lignin structure. The most important lignin-degradation products are p-coumaric acid, ferulic acid, 4-hydroxybenzoic acid, vanillic acid, syringic acid, 4-hydroxybenzaldehyde, syringaldehyde, vanillin, etc. In a recent review paper, Kumar et al. summarized various inhibitory compounds produced during lignocellulosic biomass pretreatment and different methods for hydrolysates detoxification and inhibitors removal (Kumar et al., 2020). Vanmarcke et al. (2021) also have described the inhibitor composition of different lignocellulose hydrolysates resulting from different pretreatment methods and investigated individual inhibitor effects in the case of ethanol fermentation.
Van der Pol et al. (2016) developed a small-scale rapid screening method to identify inhibitory effects of single and combined by-products from acid and alkaline hydrolysates on the growth of lactic acid-producing microorganisms. Five lactic acid-producing strains were tested—Lactobacillus casei, Lactobacillus delbrueckii, Lactococcus lactis, Bacillus coagulans, and Bacillus smithii. While in the presence of alkaline treatment by-products, L. caseis was least affected (depending on the inhibitor’s concentration), in the case of acid treatment—only growth of L. casei and L. lactis was not fully inhibited. The synergy between formic acid, acetic acid, and coumaric acid was found as a key inhibitory parameter in alkaline pretreated lignocellulose, while in the case of acid pretreated lignocellulose, furfural was the major inhibitor (van der Pol et al. (2016)). Cola et al. (2020) investigated the influence of an inhibitory cocktail (acetic acid, HMF, furfural, and p-coumaric acid) on the growth of four Saccharomyces cerevisiae and four Lactobacillus paracasei strains. Whilst all yeast strains were unable to grow in the presence of inhibitory compounds from sugarcane-based lignocellulosic hydrolysates, bacteria preserved an average of roughly 50% of their growth rates.
In view to reducing the content of inhibitory compounds, different approaches have been used. Various physicochemical methods like adsorption, ion exchange, extraction, membrane separation, overliming, etc. are applied. Other strategies involve biological treatment of the hydrolysates, gene manipulation of the microorganisms to improve their tolerance in regards to inhibitors, or plant improvement to decrease lignin content. Biological detoxification can be mediated by microorganisms or enzymes like laccase (Bhatia et al., 2020). Tramontina et al. (2020) reviewed enzyme-mediated detoxification methods for the removal of inhibitory compounds from lignocellulosic hydrolysates and described some novel strategies using classical enzymes such as laccases and peroxidases, as well as more advanced strategies using prooxidant, antioxidant, and detoxification enzymes, i.e., superoxide dismutases.
Ludwig et al. (2013) investigated detoxification of organosolv pretreated wheat straw by using free and immobilized laccase. Laccase was immobilized on Sepabeads (modified methacrylic polymer) ion-exchange resin. Free laccase was capable to remove up to 82% of phenolic compounds, while immobilized laccase could remove also HMF and acids not only by enzyme action but by the polymerization and in-situ product removal. Phenolic compounds precipitated onto the carrier surface and could be easily removed. In all cases, enzyme treatment improved the fermentability of pretreated wheat straw hydrolysate (Ludwig et al., 2013).
Liu et al. (2019) have isolated three strains (Enterococcus faecalis B101, Acinetobacter calcoaceticus C1, and Pseudomonas aeruginosa CS) capable to utilize some phenolics from ammonia pretreated corn stover (vanillin, 4- hydroxybenzaldehyde, or syringaldehyde) as a sole carbon source. Lactic acid production from 50 g/L ammonia pretreated corn stover was increased nearly twofold [from 16.98 g/L to 31.35 g/L LA (0.63 g/g corn stover)] by inoculating phenolic degrading bacteria mentioned above and lactic acid bacteria Lactobacillus pentosus FL0421 (Liu et al., 2019). In another paper, Liu et al. (2020) cultivated Trichoderma viride R16 on alkaline/peroxide pretreated corncob as the substrate in a fed-batch SSF process, and the produced enzymes were used in LA production by Bacillus coagulans LA204. Because of the high capacity of inhibitors’ degradation by Trichoderma viride R16 enzymes compared to some commercial ones the lactic acid production was increased by 24% (Liu et al., 2020).
Giacon et al. (2021) have studied the influence of two inhibitors furfural and HMF on the growth of five homofermentative (Lactobacillus plantarum CECT 221, Lactobacillus delbrueckii, Lactobacillus plantarum ESALQ 4, Lactobacillus paracasei LAB 4, Lactobacillus paracasei LAB 5) and seven (Lactobacillus fermentum DSM 20391, Lactobacillus reuteri ATCC 23272, Lactobacillus fermentum ESALQ 3, Lactobacillus fermentum ESALQ 5, Lactobacillus fermentum 1L-6-MRS, Lactobacillus fermentum 3L-2-M17, Lactobacillus paracasei LAB 2) heterofermentative lactic acid bacteria and have found that the effect of HMF and furfural on the growth rate of lactic acid bacteria (LAB) depended on the metabolic pathway. The growth kinetics in the presence of these compounds is enhanced for heterofermentative LAB, whereas is inhibitory to homofermentative LAB. The heterofermentative bacterium presented the ability to decrease the concentrations of furfural and HMF in the fermentation medium, with simultaneous lactic acid production. Low concentrations of these compounds present in the sugarcane bagasse hemicellulosic liquor did not have inhibitory effects on lactic acid production (Giacon et al., 2021).
Liu et al. (2013) compared the possibility for lactic acid production from glucose by three Pediococcus strains in the presence of inhibitors generated by acid hydrolysis of lignocellulosic biomass and reported that the strain Pediococcus acidilactici DQ2 could produce high concentrations of lactic acid from glucose in the presence of acetic acid, furfural, HMF, and vanillin in concentration range presented in acid pretreated biomass.
Oliveira et al. (2018) investigated the lactic acid production from sugarcane bagasse hydrolysates by Lactobacillus plantarum in the presence of furfural and HMF. The strain was capable to assimilate the inhibitors simultaneously with lactic acid production. A decrease of 86% for HMF and 98% for furfural was observed, together with 34.5 g/L lactic acid production. This approach could decrease the cost of the process eliminating the need for detoxification before fermentation (Oliveira et al., 2018).
Zhang et al. (2016) identified the inhibitory compounds generated during corn stover and corn cob pretreatment and investigated the toxicity limits of individual chemicals in the fermentation of hydrolysates to lactic acid by Rhizopus oryzae. They found that HMF and furfural were toxic at 0.5–1.0 g/L, while the carboxylic acid (formic, acetic, and levulinic) were non-toxic at concentrations less than 4 and 10 g/L. Among the phenolic trans-cinnamic acid and syringaldehyde had the highest toxicity at 1 g/L, while ferulic, p-coumaric, and syringic acids were not toxic. Although the concentrations in the hydrolysates were much lower than the toxicity levels of individual inhibitors, the lactic acid fermentation was considerably affected, suggesting possible synergistic action. The authors observed that while cell growth, lactate dehydrogenase, and lactic acid production were strongly inhibited, alcohol dehydrogenase and ethanol production were less or not affected. For the first time, this study showed that the inhibitors shifted the metabolic pathway from lactic acid to ethanol biosynthesis (Zhang et al., 2016).
Kunasundari et al. (2017) studied lactic acid production from oil palm sap by B. coagulans 191 strain and reported 53% conversion in case of non-treated sap, 54–74% when the sap was treated with charcoal, and up to 88% by acid and 92% for alkaline precipitation.
Cubas-Cano et al. (2020) investigated lactic acid production by three B. coagulans strains in defined media with inhibitors mixtures at high concentrations and hemicellulosic gardening hydrolysate pretreated by steam explosion. One of the isolates (A162) demonstrated high lactic acid productivity (up to 2.4 g L−1 h−1), even in presence of 5 g L−1 of furans and phenols (Cubas-Cano et al., 2020).
Zhang et al. used dry acid pretreated corn stover for LA production. The hydrolysate was neutralized by 20% Ca(OH)2 and bio-detoxified by solid-state fermentation with Amorphotheca resinae ZN1. The furfural and HMF content were fully degraded, and an increase of lactic acid production in simultaneous saccharification and co-fermentation (SSCF) of corn stover hydrolysate was observed (Han et al., 2018).
Different groups of microorganisms (bacteria, fungi, yeasts, and algae) are capable to produce lactic acid from various substrates. Although many bacteria have been extensively used for lactic acid production as a primary or secondary end-product, the term lactic acid bacteria (LAB) is used for several genera. The recently reclassified genus Lactobacillus (into 25 new genera) (Zheng et al., 2020), Lactococcus, Enterococcus, and Pediococcus, have been proven as the main producers. LAB are divided into two main groups - homo - and heterofermentative strains. Homofermentative LAB operate via Embden-Meyerhof-Parnas (EMP) pathway, expressing the aldolase enzyme with LA being the major product. They convert one molecule of glucose to two molecules of LA generating two molecules of ATP (Grewal et al., 2020). Some homofermentative strains metabolize sugars via the pentose phosphate (PP) pathway, producing 1.67 molecules LA from pentoses and hexoses and generating either 1.67 molecules (from hexose) or 0.67 (from pentose) ATP. Hetero-fermentative LAB are classified as obligate and facultative. Obligate LAB use exclusively the phosphoketolase (PK) pathway to convert one molecule of glucose or xylose to one molecule of LA and one molecule of ethanol or acetic acid and generate one molecule of ATP. Facultative heterofermentative LAB convert hexoses via EMP pathway and pentose via PK pathway (Cubas-Cano et al., 2018). Homofermentative strains producing optically pure L (+) or D (-) LA are preferred for industrial application due to the higher yield and easier downstream processing (Juturu and Wu 2016). Bacteria of genus Bacillus sp. (B. subtilis, B. coagulans B. stearothermophilus, B. licheniformis, B. licheniformis), Corynebacterium glutamicum, and Escherichia coli are also capable to produce lactic acid. Filamentous fungi are another important microbial producer of LA. Various representatives of the genus Rhizopus (R. oryzae and R. arrhizus) are utilized in free and immobilized form. The main advantages of using fungi for LA production are their amylolytic properties, simple medium requirements, their facilitate separation from the fermentation broth, and capacity to assimilate complex substrates like different lignocellulosic biomass. Numerous yeasts also can produce LA. Yeasts can tolerate low pH in comparison with LABs and thus minimizing the use of the neutralizing agent. The main disadvantage of yeasts is the low final LA concentration, but the use of engineered yeasts can overcome this drawback. The most important representatives of LA producing yeasts are Candida sp. (C. sonorensis, C. boidinii, and C. utilise), Kluyveromyces sp. (K. lactis, K. marxianus), Pichia sp. (P. stipidis, P. pastoris) as well as Saccharomyces cerevisiae. Other LA-producing species are algae and cyanobacteria. They are photosynthetic microorganisms, which can grow at minimal feed medium almost anywhere with a short growth cycle.
In a detailed review paper, Abedi and Hashemi listed almost all LA producing microorganisms together with their substrates, final LA concentration, LA yield, and productivity (Abedi and Hashemi 2020).
In Table 4 microorganisms capable of lactic acid production from lignocellulosic materials are summarized.
TABLE 4. Microorganisms, substrates, and lactic acid yield and productivity from different feedstocks.
Normally, lactobacilii can not ferment pentose sugars, which is an obstacle to the effective utilization of hemicellulosic hydrolysates as a substrate. On the one hand, some of the highly productive strains produce a racemic mixture of D- and L-lactic acid. On the other hand, in hetero-fermentative lactic acid production, by-products are formed, which decrease the effectiveness of the process and increase the cost of pure lactic acid production. Metabolic engineering is a powerful tool finding increased application for overcoming some bottlenecks in lactic acid production from lignocellulosic biomass—low yield, substrate specificity, optical purity, acid tolerance, etc. Obtaining genetically modified strains by adding genes for pentoses assimilation, deleting undesirable branches in the metabolic pathway, or deleting one of the lactate dehydrogenase genes will help in using lignocellulosic feedstocks for pure lactic acid isomers production. Wu et al. (2021) summarized the advances in techniques of genome manipulation for engineering lactobacilli and future development of genetic tools for obtaining recombinant lactic acid bacteria.
The main approaches for obtaining high-performing strains LAB are adaptive evolution, mutagenesis screening, and metabolic engineering. Each of these methods has its own advantages and disadvantages. High-throughput screening techniques can be introduced into the post-mutagenesis screening process, but still relies heavily on random mutation and is time-consuming. In contrast, both metabolic engineering and adaptive evolution approaches targeted compensating shortcomings of mutagenesis screening. Currently, commonly used methods for the gene editing and metabolic engineering of LAB are plasmid-based homologous recombination, Red/RecET-mediated double-stranded DNA recombination, and single-stranded DNA recombination. Recently emerged CRISPR/Cas9 gene-editing technique is characterized by simple operation, efficiency, and precision (Tian et al., 2021). The gene manipulation tools of lactic acid bacteria are also discussed in Borner et al. (2019), Plavec and Berlec (2020), and Cho et al. (2020).
Upadhyaya et al. (2014) highlighted four main challenges (purity of lactic acid, acid tolerance of lactic acid bacteria, carbon sources, and parameters for industrial production) and summarized metabolic engineering solutions for lactic acid production.
Mazzoli (2020) outlined two main strategies in metabolic engineering of microorganisms for consolidated lactic acid production from lignocellulosic biomass—recombinant cellulolytic strategy, consisting in introducing cellulase systems in native producers of LA, and native cellulolytic strategy, aiming at improving LA production in natural cellulolytic microorganisms.
Cubas-Cano et al. (2019) applied adaptive laboratory evolution to improve the xylose fermentation capacity of a Lactobacillus pentosus strain. In sequential batch cultivation the ratio xylose: glucose was increased gradually from 15: 5 to 20: 0. When an improvement in bacterial growth and xylose consumption was detected the xylose content in media was increased. Clones were isolated from the final population of cells at different working conditions and that showed best performance was selected for further experiments. The selected strain (MAX2) showed up to 2-fold more xylose consumption and lactic acid production in comparison with the parental strain. The strain possessed high acidic tolerance and was capable to convert mixed sugars presented in a wheat straw hydrolysate (Cubas-Cano et al., 2019).
Other microorganisms were also been engineered for high lactic acid production. Yang et al. (2013) engineered Thermoanaerobacterium aotearoense by blocking the acetic acid formation pathway. The observed maximum l-lactic acid yield with the engineered strain was 0.93 g/g glucose, 0.79 g/g xylose, and 0.32 g/g xylan as a sole carbon source without any pretreatment. The obtained optical purity of l-lactic acid was 99.3% and the engineered strain was capable of high production in non-sterile fermentation (Yang et al., 2013).
Kong et al. (2019) described the genetic manipulation of a yeast strain Kluyveromyces marxianus for effective LA production from corncob. Introduction of heterologous lactate dehydrogenase gene (from Plasmodium falciparum or Bacillus subtilis) and the proton-coupled monocarboxylate transporter gene from Saccharomyces cerevisiae together with disruption of putative d-lactate dehydrogenase in Kluyveromyces marxianus led to production of 103.00 g/l l-lactic acid with optical purity of 99.5% from 180.00 g/L corncob residue via simultaneous saccharification and co-fermentation (Kong et al., 2019).
Kuo et al. (2015) isolated twenty-six strains from soil and putrid fruits and tested the ability of 11 of them to ferment wood hydrolysate. Lactobacillus paracasei 7B showed high lactic acid productivity and high tolerance to inhibitors was chosen for further gene manipulation by interruption of ldhD gene. The resulting strain was able to ferment glucose and lignocellulosic hydrolysates of wood and rice straw without detoxification and achieved high yields—215 g/L in fed-batch glucose fermentation, 99 g/L from the undetoxified and 102 g/L from the detoxified hydrolysate (Kuo et al., 2015).
Turner et al. (2016) constructed a recombinant Saccharomyces cerevisiae strain capable of assimilating cellobiose and xylose and producing lactic acid. Different genes (cdt-1, gh1-1, XYL1, XYL2, XYL3, and ldhA) coding cellobiose transporter, β-glucosidase, xylose reductase, xylitol dehydrogenase, xylulokinase, and lactate dehydrogenase were integrated into the S. serevisiae. In the engineered strain native pyruvate decarboxylase (pdc) and alcohol dehydrogenase (adh) genes were not deleted, but still, almost no ethanol was produced when fermenting a cellobiose and xylose mixture. The engineered strain produced 83 g/L of lactic acid with a yield of 0.66 g/g sugar from a cellulosic sugar mixture (10 g/L glucose, 40 g/L xylose, and 80 g/L cellobiose) (Turner et al., 2016).
Kim et al. (2019) engineered Saccharomyces cerevisiae SR8 to produce lactic acid and ethanol from pretreated spent coffee grounds. The pRS41N-Cas9 plasmid was introduced and expression of the lactate dehydrogenase gene was confirmed (Kim et al., 2019).
Using helium-based atmospheric and room temperature plasma mutation and evolution Jiang et al. (2016) adapted Bacillus coagulans NL01 strain to overcome the inhibitors’ action in corn stover hydrolysates. The mutant strain Bacillus coagulans GKN316 was capable to convert different hydrolysates to lactic acid with high inhibitor tolerance. The individual inhibitory effect of furfural, 5-hydroxymethylfurfural, vanillin, syringaldehyde, and p-hydroxybenzaldehyde was also studied and was found that the syringyl compound was most toxic. The strain B. coagulans GKN316 could effectively convert these inhibitors to the less toxic corresponding alcohols (Jiang et al., 2016).
Most of the microorganisms producing lactic acid ferment sugars to l-lactic acid and there are only a few capable to produce d-lactic acid. In recent years, the demand for d-lactic acid has increased, especially for the production of polylactic blends (stereo-complex polylactic acid, composed of both L and d-lactic acid) which is characterized by a high melting point. Therefore, the interest in d-lactic acid-producing strains, including genetically manipulated also increases.
Zhang et al. investigated d-lactic production from glucose, xylose, and alkaline corn stover hydrolysate by an l-lactate deficient strain of Lactobacillus plantarum in which xylose-assimilating genes encoding xylose isomerase and xylulokinase were successfully cloned. The engineered strain produced 19,7 g/l d-lactic from 40 g/L xylose in a batch experiment. In a fed-batch mode production of 30.1 g/L of d-lactic was achieved. When a mixture of glucose and xylose (2:1 ratio) was used as a substrate, 47.2 g/l d-lactic was produced, with a lactic acid yield of 0.84 g/g. The experiments with corn stover were carried out in two modes—separate saccharification and fermentation (SHF) and simultaneous saccharification and fermentation (SSF). In the first case, 19.4 g/l d-lactic acid was produced, while in the second—26.8 g/L when the hydrolysate was supplemented with yeast extract and 29.4 g/L when soybean meal extract was added. Finally, in a fed-batch experiment with corn stover hydrolysate in SSF mode production of 61.3 g/l d-lactic acid was achieved. The production of acetic acid was observed in all experiments (Zhang et al., 2016). In other work, the authors constructed and compared an l-lactate-deficient mutant strain Lactobacillus plantarum NCIMB 8826 ldhL1 and its derivative harboring a xylose assimilation plasmid (DldhL1-pCU-PxylAB). Recombinant DldhL1-pCU-PxylAB used xylose to produce high yields of d-lactic acid and was able to ferment xylose and glucose simultaneously which is an important advantage when using lignocellulosic biomass as a substrate for producing lactic acid. As substrates for d-lactic acid production, corn stover and sorghum stalk hydrolysates were successfully used. DldhL1-pCUPxylAB produces 20% more d-lactic acid than DldhL1 from lignocellulosic biomass. In the SSCF process the yield increased about 38%, and productivity—almost three-fold when compared with SHF process (Zhang et al., 2016).
In a series of three papers, Kondo and coworkers studied the possibility of d-lactic production with engineered Lactobacillus plantarum strains. Firstly, they engineered an l-lactate dehydrogenase-deficient Lactobacillus plantarum strain for d-lactic acid production from cellulosic materials. When an endoglucanase-secreting plasmid was introduced into L. plantarum 1.27 g/l d-lactic acid was produced with 2 g/L cellohexaose as substrate and 1.47 g/L with barley β-glucan as substrate. Although anaerobic conditions partially suppressed this conversion of d-lactic to acetic acid, the final product was predominantly acetic acid (Okano et al., 2010). In another paper, the xylose assimilating operon from Lactobacillus pentosus was introduced into an l-lactate dehydrogenase deficient Lactobacillus plantarum to achieve efficient d-lactic acid fermentation from a mixture of xylose and glucose. Successful homo-d-lactic acid production was achieved 41.0 g/L lactic acid (88% g/g xylose yield and 98.7% optical purity) from 50 g/L xylose. In the case of a mixture of xylose and glucose (1:3 ratio) 74.2 g/L of lactic acid was produced with 0.78 g/g sugar yield with d-lactic acid optical purity of 99.5%. Finally, a mixture of three sugars—xylose, glucose, and arabinose (5:10:1 ratio) and 61.2 g/L of lactic acid was produced with a yield of 0.80 g/g sugar consumed and 99.5% optical purity was produced from 80 g/L sugars. Simultaneous sugars utilization was achieved without carbon catabolite repression (Yoshida et al., 2011). The last paper was focused on the d-lactic acid production from delignified hardwood pulp. The previously described Lactobacillus plantarum mutant (Yoshida et al., 2011) was used for d-lactic acid production from both glucose and xylose in a simultaneous saccharification and fermentation (SSF) process. The SSF resulted in 55.2–84.6 g/L lactic acid, depending on the load (5–15%) after 72 h. To improve the enzymatic saccharification at high-load, short-term pulverization of pulp was conducted. The pretreatment significantly ameliorated saccharification and suppressed the formic acid by-product formation. In this case, SSF resulted in a lactic acid production of 102.3 g/(0.88 g/g-sugars yield) and optical purity of 99.2%. (Hama et al., 2015).
Žunar et al. (2020) manipulated a wild strain Lactobacillus gasseri JCM 1131T firstly by transforming with plasmids carrying additional copies for each of the three lactate dehydrogenases that the wild type encodes for. Secondly, each of the three endogenous genes for lactate dehydrogenases was inactivated using the plasmid pHBintE, which was used for the first time to inactivate genes in lactobacilli. Transformation of L. gasseri with plasmids carrying additional genes for L- or d-lactate dehydrogenases didn’t affect the ratio of produced stereoisomers, but inactivation of the endogenous genes created strains which yielded 0.96 g/g glucose of either L- or d-lactate. Constructed strains efficiently fermented wheat straw hydrolysate and produced 0.37–0.42 g of lactate/g wheat straw (Žunar et al., 2020).
Prasad et al. (2020) described the application of an evolutionary engineered thermo-tolerant strain of Lactobacillus bulgaricus strain in d-lactic acid production from rice straw. The engineered strain was capable to work at 45°C and thus be used in an SSF process enzyme significantly reducing the enzyme loading. The powdered rice straw biomass was pretreated by dilute acid followed by steam explosion. The rice straw was soaked with 0.2% w/v H2SO4 for 24 h and then heated to 175°C for 30 min followed by rapid decompression to atmospheric pressure. The pretreated rice straw contained 45.84% cellulose and 4.63% xylan and was used in further experiments after enzymatic hydrolysis with commercial cellulase complex (SacchariSEB C6L Plus). SHF and batch and fed-bach SSF mode of fermentation were carried out. In batch SSF experiments 11.8–32.4 g/l d-lactic acid was produced, depending on the enzyme and total solid loading, while in fed-batch SSF the final obtained lactic acid concentration was 108.6 g/L (Prasad et al., 2020).
Tian et al. (2021) developed through the CRISPR-Cas9 gene-editing platform a high optical purity l-lactic acid producing strains. Further, by adaptive evolution a high-performance strain (NCBIO01-M2-ldhL1-HT) was obtained, that could efficiently produce l-lactic acid at a high temperature (45°C). The strain was capable of producing 221.0 g/L of l-lactic acid in open fermentation. The productivity and yield were above 7.5 g/L/h and 0.96 g/g respectively, and the optical purity of l-lactic acid exceeded 99.1% (Tian et al., 2021).
A lactogenic Escherichia coli strain JU15 (MG1655, ΔpflB, ΔadhE, ΔfrdA, ΔxylFGH, gatC-S184L, ΔmidarpA, and Δreg 27.3 kb) was used and modified to produce d-lactic acid from sugarcane bagasse and corn stover hydrolysates. The strain JU15 was additionally genetically modified by deleting the pathways for the production of l-lactic acid and acetic acid, thus leading to a new strain - AV03 (JU15, ΔpoxB, ΔackA-pta, ΔmgsA). While JU15 showed sequential sugars assimilation and acetic acid production, AV03 showed simultaneous sugars consumption no acetic acid production, with a minimal nutrient addition in pH-controlled fermentation. The d-lactic acid yield in all cases was close to 0.95 g/g sugars (Utrilla et al., 2016).
Werlang et al. (2020) have also used the recombinant Escherichia coli strain JU15 for d-lactic acid production from algal biomass pretreated by acid and enzyme hydrolysis. The strain was capable of producing 25.5 g/L d-lactate (86% of the theoretical conversion of glucose to lactate) after 9 h of fermentation with 0.255 g/g of dried A. platensis biomass (Werlang et al., 2020).
Cells immobilization offers undoubted advantages in a fermentation process such as the possibility of realization of fed-batch or continuous process; high cells concentration; increased stability of the biocatalyst and reduced influence of substrate and product inhibition; easier separation of biocatalyst from fermentation broth; and higher fermentation rate. Different immobilization methods were used in lactic acid production from biomass—adhesion, encapsulation, gel entrapment, etc.
Radosavljevic et al. (2021) used L. rhamnosus cells immobilized by encapsulation in polyvinyl alcohol cryogel for LA production from brewer’s spent grain (BSG) and malt rootlets (MR) hydrolysates. For hydrolysate production, dry BSG and MR in ratio 4:1 were sequentially mixed with Termamyl SC (amylase), SAN Super 240 L (glucoamylase), and Cellic CTec2 (cellulase) enzymes. After enzymatic hydrolysis, the obtained hydrolysate was separated from solids and vacuum concentrated to 5.5% reducing sugars content before was used in LA fermentations. Application of 10% PVA in BSG and MR hydrolysate throughout 12 consecutive batch fermentations resulted in high LA yield and volumetric productivity of 97.1% and 2.1 g/L h−1, respectively (Radosavljevic et al., 2021).
Lactobacillus bifermentans cells were immobilized in Ca-alginate gel for lactic acid production from the wheat bran hemicellulosic hydrolysate. Cells entrapped in calcium alginate beads can consume all glucose and arabinose and 75% of xylose in one step. The maximum values of lactic acid yield, productivity, and percent sugar utilization were 0.83 g/g, 1.17 g/l.h, and 76%, respectively, at temperature 42°C and pH 7.5 (Givry et al., 2008).
Mladenovic et al. (2018) have used Lactobacillus paracasei immobilized by adhesion onto agro-industrial residues—sunflower seed hull, brewer’s spent grain, and sugar beet pulp for lactic acid production from distillery waste potato stillage remaining after bioethanol production. The immobilized preparations were stable at least in five consecutive cycles and a maximal LA concentration of 80.1 g/L with an average yield coefficient of 0.97 g/g was achieved using sugar beet pulp. The LA productivity was 1.48 g/l.h. In a later paper (Mladenovic et al., 2019) extended their research on the possibility of simultaneous production of LA and probiotic enriched livestock feed on a combined substrate based on molasses and potato stillage. The highest total LA concentration of 399 g/L and overall productivity of 1.27 g/l.h was achieved in five repeated fermentation batches.
Shi et al. (2012) investigated the production of l-lactic acid by immobilizing Lactococcus lactis cells in a fibrous bed bioreactor system. The substrate was Jerusalem artichoke hydrolysate and the immobilization was done by adhesion in a column packed with spiral wound cotton towel. Using the fed-batch strategy, 142 g/L lactic acid was produced. Subsequent repeated-batch fermentations further exhibited the persistence and stability of the system for the high production of l-lactic acid in a long term.
Lactobacillus plantarum 23 immobilized in polyvinyl alcohol beads was tested for LA production from renewable feedstocks (Nagarajan et al., 2020). Sugarcane bagasse, cheese whey, and microalgal biomass of Chlorella vulgaris and Ulva sp. were used as substrates after pretreatment. Sugarcane bagasse was hydrolyzed by two steps—phosphoric acid hydrolysis to remove the lignin fraction and obtain bagasse cellulose, followed by sulfuric acid hydrolysis to hydrolyze the cellulose. Microalgal biomass was subjected to a combined acid/thermal pretreatment (4% H2SO4, 120°C, 20 min). Lactic acid fermentation was carried out in batch and continuous mode. The best results were obtained with sugarcane bagasse—41–42 g/L LA and 0.98–1.0 g/g sugars and microalgal biomass—37.9 g/L and 0.91 g/g sugars. In another publication (Chen et al., 2020) extended the research on LA production from Chlorella vulgaris biomass with PVA immobilized Lactobacillus plantarum, including in-situ LA removal by ion exchange. Optimal conditions of fermentation were determined and a substantial increase in LA yield (72%) was observed in the case fed-batch coupled with ion exchange. Continuous fermentation using immobilized L. plantarum with high productivity was demonstrated (14.22 g/l.h from glucose and 9.93 g/l.h from algal biomass) and immobilized beads could be used for 4 months without loss of activity.
Thakur et al. (2019) investigated lactic acid production from sugarcane molasses by calcium alginate immobilized Lactobacillus casei MTCC 1423 cells. Different process parameters (bead diameter, bead coating, biomass concentration, shaking speed, substrate concentration, nitrogen content, temperature, incubation time, and pH) were optimized. The immobilized cells were stable between 4 and 9 cycles depending on the bead coating method. At optimal conditions, 128.45 g/L lactic acid was achieved (Thakur et al., 2019).
All well-known biotechnological fermentation methods are also applicable in lactic acid fermentation from lignocellulosic and industrial wastes. Batch fermentation is the widely used and simplest method. The fermentation medium is seeded with the appropriate microorganism and no product is extracted or substrate is added to the end of the process. High final LA concentration and low risk of contamination are the main advantages of this fermentation mode, while low cell densities, low productivity, and substrate and/or product inhibition are the main disadvantages. The substrate inhibition can be overcome by fed-batch—substrate is added in portions and no product is withdrawn, but still, product inhibition is the major obstacle. In repeated batch (semicontinuous), fermentation a part or all of the cells from a previous run are inoculated into the next run. The semicontinuous mode can assure a decrease in time, energy costs, and increased LA productivity. Continuous fermentation is realized by steadily fresh medium feeding and fermentation broth withdrawal. The dilution rate (the velocity of substrate feeding and product withdrawal) must be carefully chosen, so no cell washing or product accumulation is accomplished. The main disadvantages of this process organization are incomplete substrates utilization and low LA concentration. High cell density fermentation using cell recycling is an efficient strategy for enhancing sugar consumption. The cells retention by ultrafiltration permits separation and recirculation. Cells immobilization can resolve the problem with retention of the cells into the system and facilitate continuous process realization. Different reactor types– continuous stirred tank, packed bad, and fibrous bad were utilized.
The necessity of individual sugars production by hydrolysis of cellulose and hemicellulose in pretreated lignocellulosic biomass leads to the use of enzymes like amylases and cellulases. Separate hydrolysis and fermentation (SHF) and simultaneous saccharification and fermentation (SSF) are the main strategies for lignocellulosic and waste biomass utilization. An asset of SHF is that hydrolysis and fermentation are separately performed under their optimal conditions. Usually, hydrolysis and fermentation have different optimal conditions (temperature and pH). The drawback is that during enzymatic hydrolysis, the sugars released might lead to an increase of the inhibition and suppress enzyme activity. On the other hand, SSF offers more advantages than SHF—the possibility of a one-pot process, reducing time and cost of fermentation, and reducing inhibition. The mentioned above difference in optimal temperatures and pH for both hydrolysis and fermentation is the main drawback of SSF. A possible way of overcoming this discrepancy lies in using microorganisms releasing cellulitic enzymes during so-called simultaneous saccharification and co-fermentation (SSCF). Tarraran and Mazzoli (2018) reviewed recent research in co-fermentation of LAB with native cellulolytic microorganisms, as well as the construction of recombinant cellulolytic LAB by metabolic engineering, for direct fermentation of plant biomass for reducing the high cost of exogenous cellulase supplementation. Zaini et al. (2019) compared SHF and SSF approaches for d-lactic acid production from DDGS by Lactobacillus coryniformis. They reported that SSF demonstrated better fermentation characteristics compared to SHF [Zaini, Chatzifragkou and Charalampopoulos, Microbial production of d-lactic acid from Dried Distillers Grains with Solubles (DDGS) 2019]. An additional plus offers the possibility of such microorganisms to grow in the presence of inhibitors released during pretreatment. Some of them use the inhibitors as a carbon source and thus decreasing their concentration and negative effect on LA fermentation. Examples of using different modes of fermentation are listed in Table 4.
Integration of fermentation and separation steps in situ extractive fermentation gives the possibility of increasing productivity and decreasing energy consumption. Different methods are proposed for lactic acid removal—ion exchange (Othman et al., 2018), membrane bioreactors (Gössi et al., 2020), liquid-liquid extraction (Lan et al., 2019). Although process integration is well documented in the case of lactic acid production from sugars (M. Othman et al., 2017), there are no published results for second-generation biomass.
Some advantages and drawbacks of different process organizations are presented in Table 5.
Downstream processing (separation, purification, and recovery) is a very important step in lactic acid production and its cost can reach up to 50% of the total price of the final product. In general, separation and purification methods of second-generation LA (from lignocellulosic feedstocks) don’t differ from these applied for first-generation LA. Having in mind that the use of pretreated biomass for LA production can result in a more complex fermentation broth, containing more impurities (unfermented pentoses and hexoses, inhibitory substances, lignin, protein, pigments, and salts), a more complex and expensive downstream process will be needed. Therefore, searching for a highly effective, improving LA yield, low-cost, and environmentally friendly method is of great importance. It is well known that LA fermentation is characterized by relatively low final concentration and low production rate, due on one hand, to the change of pH caused by acid accumulation and, on the other hand, to the product inhibition. Hence, neutralization of the broth or in situ removal of the product and thus increasing productivity is necessary. Sodium hydroxide, ammonia Ca(OH)2, or CaCO3 are used as neutralizing agents.
Conventionally, industrially produced by fermentation LA is removed by precipitation with Ca(OH)2. The method suffers from many drawbacks—precipitated calcium lactate has considerable water solubility [5–20%, depending on temperature (10–50°C)], additional steps have to be applied for converting lactate to free lactic acid—filtration, acidification with H2SO4 (generates a large amount of CaSO4), activated carbon purification and decolorization, concentration by evaporation and crystallization. In the case of lignocellulosic biomass-derived substrates, the separation process is additionally complicated by other simultaneously precipitated.
Because of numerous disadvantages of the precipitation method, different techniques have been investigated as an alternative to the traditional recovery process. These include liquid-liquid extraction (including liquid membranes), electrodialysis, ion exchange, membrane separation, aqueous two-phase systems, etc.
Liquid-liquid extraction is a separation method based on different solubility of the target compound in water and immiscible organic solvent (hydrocarbons or alcohols with high molecular mass). For increasing the partition a chemical called extractant is added to the organic phase. The target compound (lactic acid) forms a complex with the extractant with an increased affinity towards the organic phase.
Different membrane-based separation techniques (micro-, ultra-, nano-filtration, reverse osmose, and pervaporation) can be successfully used as a preliminary step for lactic acid purification. These methods are environmentally friendly and easily scaled up. Usually, they are employed for the separation of cells, proteins, and sugars.
Adsorption is a powerful method for bioseparation with easy regeneration, low energy consumption, and high selectivity. Neutral (activated carbon) or ion exchange adsorbents can be used. Din et al. (2021) analyzed in-depth second-generation lactic acid separation and recovery from fermentation broth by ion exchange. The authors highlighted different factors influencing the process and briefly discussed other methods for LA separation.
Electrodialysis is a method facilitating salt ions transport through an ion-exchange membrane from one to another solution by applying an electric field. Mono- and bipolar electrodialysis are widely used for lactic acid separation.
Aqueous two-phase systems (ATPS) are formed when two water-soluble polymers (or a polymer and a salt) are mixed in a common solution. Because of the incompatibility of the constituents, the system is separated into two phases. ATPS are extensively used in the separation of various bioproducts. Zhou et al. (2021) investigated the separation of lactic acid from simulated and actual lignocellulosic (from SSCF of dilute acid-pretreated corn stover) fermentation broth. In a three-stage ionic liquid-based sugaring-out extraction from the filtered and unfiltered fermentation broth, the total recovery of LA was 89.5 and 89.4%, respectively (Zhou et al., 2021).
Other methods like molecular distillation, short path evaporation, salting-out extraction, emulsion liquid membranes are also tested for lactic acid separation. There are numerous papers concerning lactic acid separation from the fermentation broth, but the majority of them are devoted to lactic acid produced of sugars or starchy materials (first generation LA), and data for the separation of lactic acid obtained from lignocellulosic biomass are scarce. Several review papers summarize recent progress in lactic acid separation by different methods (Kumar et al., 2019; Li et al., 2021; Yankov 2020). De Oliveira et al. (2020) discussed current state-of-the-art on the separation and purification methods of lactic acid derived from the fermentation of second-generation feedstocks.
Baral et al. (2021) investigated lactic acid separation from the broth after sugarcane bagasse hydrolysate fermentation. Various extractants (tripropylamine, trihexylamine, trioctylamine, triisootcylamine, and tributylphosphate) and diluents (chloroform, dodecane, ethyl acetate, MIBK, hexane, and 1-octanol) were screened for choosing the best extractant-diluent pair. Tributylphosphate and ethyl acetate were selected because of their higher extraction efficiency—59.63 ± 1.28%. Different salts—calcium chloride, ammonium sulfate, sodium dihydrogen phosphate, and,di-potassium hydrogen phosphate were used in the view to enhance extraction. Salting-out extraction with 60% ammonium sulfate increased the lactic acid extraction efficiency to 85.95 ± 0.44%. About 80% salt recovery was achieved using chilled acetone (Baral et al., 2021).
Demmelmayer and Kienberger (2022) studied reactive extraction of lactic acid from sweet sorghum silage press juice. Different extractants (dioctylamine, trioctylamine, and Aliquat 336) and diluents/modifiers (octanol, decanol, hexane, nonane, and undecane) were used in various ratios. Stripping of the loaded organic phase was done by sodium hydroxide. The extraction efficiency of 41.1% was reached using system DOA/ALIQ:1–octanol:n–nonane (35:35; 15:15) with a stripping efficiency of 98.2% (Demmelmayer and Kienberger 2022).
Hu et al. (2017) explored lactic acid recovery from fermentation broths of food waste and bakery waste hydrolysates by continuous ultrasonic solvent extraction. They compared the extraction efficiency of six organic solvents and chose ethyl acetate (EA) as the best option. The extraction into ethyl acetate (LA:EA ratio 1:2) by sonication at pH 2 under room temperature resulted in 82–84% yield and 98% purity (Hu et al., 2017).
Lan et al. (2019) compared two methods for lactic acid extraction from corn stover hemicellulose-derived liquor (HDL). In the first one, 10% trioctylamine in octanol was used with 50.8% extraction efficiency. An additional salting-out extraction step was included before TOA extraction. The salting-out extraction was done in THF/NaCl system. The overall efficiency of 83% was achieved in the two-step extraction of an activated carbon-treated HDL. The process provided stable LA removal during five consecutive extraction/striping cycles (Lan et al., 2019).
Xu et al. (2018) studied salting-out extraction of lactic acid in systems composed of Na2SO4 and organic solvent (1,4-dioxane, THF, γ-butyrolactone, and γ-valerolactone). Best results were obtained with the system Na2SO4/1,4-dioxane, and the conditions for LA extraction were further optimized. In the case of 13.5% Na2SO4/28% 1,4-dioxane, at low pH (1.68) 91% extraction efficiency was achieved in the case of model LA solution and 90% from real mixture directly derived from the chemical catalytic conversion of corn stover (Xu et al., 2018).
Chen et al. (2020) realized a combined process for LA production and separation. After fermentation of corn cob hydrolysate, the fermentation broth was fed into an ultrafiltration cell and retentate, containing mainly cellulase was recycled. The permeate was acidified to liberate free acid from calcium lactate and the liquid fraction was transferred in a nanofiltration unit. The permeate was further purified by distillation for pure lactic acid production, while the retentate, containing mainly residual sugars was transferred back in the fermentor. A total of six cycles of fed-batch SSF were performed (Chen et al., 2020).
Short-path evaporation of lactic acid from hemicellulose hydrolysate after acid hydrolysis of sugarcane bagasse was investigated by De Oliveira et al. (2020) During the fermentation pH of the broth was maintained by Ca(OH)2. At the end of fermentation, the broth was treated with sulphuric acid for calcium lactate destroying and then filtered and centrifuged. The clear liquid was evaporated and a concentration of 3 times (from 27.85 g/L to 86.69 g/L) was obtained at 120°C evaporator temperature of, 13°C condenser temperature of, and 8.27 ml/min feed flow rate. The authors mentioned a more difficult process than the separation of LA from sugars (Oliveira et al., 2019).
Garett et al. (2015) realized an in situ extractive fermentation of lactic acid from corn stover sugars. The corn stover was pretreated by a wet explosion followed by enzyme hydrolysis. The resulting sugar solution was fermented by a mixed culture, dominated by a Bacillus coagulans strain. A weak base resin Amberlite IRA-67 was used for LA removal during fermentation. The base eluted resin was able to maintain stable fermentation over 108-days, during which the average loading of lactic and acetic acids was 112.2 and 19.6 mg acid/g resin, respectively (Garrett et al., 2015).
Oonkhanond et al. (2017) used pretreated sugarcane bagasse in an integrated system with ex-situ nanofiltration. The first step fractionation was carried out using acid ethanolysis (5N H2SO4 in 50% v/v ethanol) at 90°C for 4 h. In the second step, the neutralized solid part was treated with an alkaline/peroxide reaction at 4.5% wt H2O2 at pH 11.5 for 24 h at 60°C. and finally was hydrolyzed by Accellerase 1,500 at pH 5.0, 50°C for 96 h. Using nanofiltration with a low flux membrane, lactic acid was efficiently separated from the fermentation broth, and after vacuum distillation, a lactic acid solution with 80% purity was achieved (Oonkhanond et al., 2017).
Alexandri et al. (2018) carried out a pilot-scale LA production using a defined medium with glucose, acid whey, sugar bread, and crust bread, coupled with membrane technologies. Microfiltration and nanofiltration were successfully used to separate lactic acid from the majority of the other fermentation components, leading to a more than 10% improvement of lactic acid’s purity (Alexandri et al., 2018).
Neu et al. (2016) explored different methods for downstream processing of a lactic acid stream obtained after fermentation of coffee mucilage. Micro- and nanofiltration, electro-dialysis, ion-exchange, and distillation result in a 930 g/L lactic acid solution with 99.8% purity (Neu et al., 2016).
Olszewska-Widdrat et al. (2019) investigated complex method combining various technics in LA separation from sweet sorghum juice—filtration and ultra-filtration, chelating resin, mono- and bipolar electrodialysis, followed by chromatography and vacuum evaporation were subsequently carried out producing a 905.8 g/L lactic acid solution, with an optical purity of 98.9% (Olszewska-Widdrat et al., 2019).
Prado-Rubio et al. (2020) presented a techno-economic assessment of lactic acid (produced from sugarcane feedstock) separation. The evaluation of three separation methods (reactive distillation, reactive extraction, and electrodialysis) was made based on a LA/water stream in mass ratio 0.3038/0.6962. The calculations were made using a specific methodology design for each separation technique applying Aspen Plus, Matlab, and their combination. Taking reactive distillation as a base, other methods showed a significant reduction in total annual cost—reactive extraction—44% and electrodialysis—55% (Prado-Rubio et al., 2020).
Mandegari et al. (2017) investigated four scenarios for ethanol and/or lactic acid production in lignocellulose biorefineries annexed to a typical sugar mill using Aspen Plus simulator. Economic evaluation, energy assessment, and environmental life cycle assessment (LCA) were carried out. The LCA suggested that all LA producing scenarios introduced environmental burdens (Mandegari et al., 2017).
In the way of decreasing dependence on petroleum-based production of valuable chemicals, considerable efforts are concentrated on the investigation of different lignocellulosic feedstocks as raw materials for fermentative production of various chemical products. Fermentative lactic acid production from first-generation biomass is an example of a growing production process. High cost of the substrates and enzymes, some environmental issues, food competition, and waste generation are the factors hindering the industrial process implementation. Second and third-generation sources present an attractive alternative for lactic acid production. From the summarized here research during the last 5 years, the most employed feedstock is corn stover and the most used pretreatment method—acid and enzyme hydrolysis. Nevertheless, there are numerous challenges in the route towards a cost-effective process—separation of lignin from cellulose and hemicellulose; release of inhibitory compounds during pretreatment; product and substrate inhibition; and by-product formation in fermentation; the more complex composition of fermentation broth in and ecological problems connected with classical precipitation separation, etc. However, there are some encouraging signs of developing an economically effective and environmentally friendly method for lactic acid production from renewable resources. First of all, is the progress in using green solvents like ionic liquids, capable of being more effective and producing fewer inhibitors during lignocellulose dissolution. What is more, ionic liquids have very tunable characteristics and can be easily tailored for different feedstocks. Secondly, developing genetically modified microorganisms with high productivity and adapted to inhibitors. Finally, process integration—elaboration of different SSF processes with different microorganisms, as well as in situ extraction, both leading to minimization of the substrate and product inhibition and thus increasing the yield and decreasing the total cost.
In general, the development of such innovative, sustainable, and cost-effective method with high yield and purity of lactic acid is still on the horizon. The most promising method for lactic acid production from lignocellulosic feedstocks seems to be pretreatment with DES, followed by SSF (or SSCF) in fed-batch or continuous mode.
DY contributed to the conceptualization, the literature research, the writing of the manuscript, and the design of the figures.
The author declares that the research was conducted in the absence of any commercial or financial relationships that could be construed as a potential conflict of interest.
All claims expressed in this article are solely those of the authors and do not necessarily represent those of their affiliated organizations, or those of the publisher, the editors and the reviewers. Any product that may be evaluated in this article, or claim that may be made by its manufacturer, is not guaranteed or endorsed by the publisher.
The author is thankful to the National Science Fund of Bulgaria for the financial support by the contract KP-06-Russia-10/2020, which made this study possible.
Abdel-Rahman, M. A., Hassan, S. E.-D., Alrefaey, H. M. A., El-Belely, E. F., Elsakhawy, T., Fouda, A., et al. (2021). Subsequent Improvement of Lactic Acid Production from Beet Molasses by Enterococcus Hirae Ds10 Using Different Fermentation Strategies. Bioresour. Technol. Rep. 13, 100617. doi:10.1016/j.biteb.2020.100617
Abedi, E., and Hashemi, S. M. B. (2020). Lactic Acid Production - Producing Microorganisms and Substrates Sources-State of Art. Heliyon 6, e04974. doi:10.1016/j.heliyon.2020.e04974
Ahmad, A., Banat, F., and Taher, H. (2021). Comparative Study of Lactic Acid Production from Date Pulp Waste by Batch and Cyclic-Mode Dark Fermentation. Waste Manage. 120, 585–593. doi:10.1016/j.wasman.2020.10.029
Ahring, B. K., Traverso, J. J., Murali, N., and Srinivas, K. (2016). Continuous Fermentation of Clarified Corn stover Hydrolysate for the Production of Lactic Acid at High Yield and Productivity. Biochem. Eng. J. 109, 162–169. doi:10.1016/j.bej.2016.01.012
Ajala, E. O., Olonade, Y. O., Ajala, M. A., and Akinpelu, G. S. (2020). Lactic Acid Production from Lignocellulose - A Review of Major Challenges and Selected Solutions. ChemBioEng Rev. 7 (2), 38–49. doi:10.1002/cben.201900018
Akao, S., Nagare, H., Maeda, M., Kondo, K., and Fujiwara, T. (2016). Non-sterile Simultaneous Saccharification and Fermentation of Corn Leaves and Stalks to L-Lactic Acid without External Nutrient Addition. J. Mater. Cycles Waste Manag. 18, 208–214. doi:10.1007/s10163-015-0390-y
Åkerberg, C., and Zacchi, G. (2000). An Economic Evaluation of the Fermentative Production of Lactic Acid from Wheat Flour. Bioresour. Technol. 75, 119–126. doi:10.1016/s0960-8524(00)00057-2
Alexandri, M., Schneider, R., and Venus, J. (2018). Membrane Technologies for Lactic Acid Separation from Fermentation Broths Derived from Renewable Resources. Membranes 8, 94. doi:10.3390/membranes8040094
Alhafiz, H. A., Isa, M. T., Sallau, A. B., and O Ameh, A. (2020). Delignification of Corn Cob for the Synthesis of Lactic Acid. J. Niger. Soc. Chem. Eng. 35 (1), 64–70.
Alrumman, S. A. (2016). Enzymatic Saccharification and Fermentation of Cellulosic Date palm Wastes to Glucose and Lactic Acid. Braz. J. Microbiol. 47, 110–119. doi:10.1016/j.bjm.2015.11.015
Alves de Oliveira, R., Komesu, A., Vaz Rossell, C. E., Wolf Maciel, M. R., and Maciel Filho, R. (2019). Concentrating Second-Generation Lactic Acid from Sugarcane Bagasse via Hybrid Short Path Evaporation: Operational Challenges. Separat. Purif. Technol. 209, 26–31. doi:10.1016/j.seppur.2018.07.012
Alves de Oliveira, R., Schneider, R., Vaz Rossell, C. E., Maciel Filho, R., and Venus, J. (2019). Polymer Grade L-Lactic Acid Production from Sugarcane Bagasse Hemicellulosic Hydrolysate Using Bacillus Coagulans. Bioresour. Technol. Rep. 6, 26–31. doi:10.1016/j.biteb.2019.02.003
Alves de Oliveira, R., Vaz Rossell, C. E., Venus, J., Cândida Rabelo, S., and Maciel Filho, R. (2018). Detoxification of Sugarcane-Derived Hemicellulosic Hydrolysate Using a Lactic Acid Producing Strain. J. Biotechnol. 278, 56–63. doi:10.1016/j.jbiotec.2018.05.006
Asim, A. M., Uroos, M., Naz, S., and Muhammad, N. (2021). Pyridinium Protic Ionic Liquids: Effective Solvents for Delignification of Wheat Straw. J. Mol. Liquids 325, 115013. doi:10.1016/j.molliq.2020.115013
Avci, U., Zhou, X., Pattathil, S., da Costa Sousa, L., Hahn, M. G., Dale, B., et al. (2019). Effects of Extractive Ammonia Pretreatment on the Ultrastructure and Glycan Composition of Corn Stover. Front. Energ. Res. 7, 85. doi:10.3389/fenrg.2019.00085
Azaizeh, H., Abu Tayeh, H. N., Schneider, R., Klongklaew, A., and Venus, J. (2020). Production of Lactic Acid from Carob, Banana and Sugarcane Lignocellulose Biomass. Molecules 25, 2956. doi:10.3390/molecules25132956
Ballesteros, L. F., Teixeira, J. A., and Mussatto, S. I. (2014). Chemical, Functional, and Structural Properties of Spent Coffee Grounds and Coffee Silverskin. Food Bioproc. Technol 7, 3493–3503. doi:10.1007/s11947-014-1349-z
Banu, J. R., Preethi, S. K., Kavitha, S., Tyagi, V. K., Gunasekaran, M., Karthikeyan, O. P., et al. (2021). Lignocellulosic Biomass Based Biorefinery: A Successful Platform towards Circular Bioeconomy. Fuel 302, 121086. doi:10.1016/j.fuel.2021.121086
Baral, P., Munagala, M., Shastri, Y., Kumar, V., and Agrawal, D. (2021). Cost Reduction Approaches for Fermentable Sugar Production from Sugarcane Bagasse and its Impact on Techno-Economics and the Environment. Cellulose 28, 6305–6322. doi:10.1007/s10570-021-03940-5
Baral, P., Pundir, A., Kumar, V., Kurmi, A. K., and Agrawal, D. (2020). Expeditious Production of Concentrated Glucose-Rich Hydrolysate from Sugarcane Bagasse and its Fermentation to Lactic Acid with High Productivity. Food Bioproducts Process. 124, 72–81. doi:10.1016/j.fbp.2020.08.005
Baral, P., Pundir, A., Kurmi, A., Singh, R., Kumar, V., and Agrawal, D. (2021). Salting-out Assisted Solvent Extraction of L (+) Lactic Acid Obtained after Fermentation of Sugarcane Bagasse Hydrolysate. Separat. Purif. Technol. 269, 118788. doi:10.1016/j.seppur.2021.118788
Baruah, J., Nath, B. K., Sharma, R., Kumar, S., Deka, R. C., Baruah, D. C., et al. (2018). Recent Trends in the Pretreatment of Lignocellulosic Biomass for Value-Added Products. Front. Energ. Res. 6, 141. doi:10.3389/fenrg.2018.00141
Berlowska, J., Cieciura, W., Borowski, S., Dudkiewicz, M., Binczarski, M., Witonska, I., et al. (2016). Simultaneous Saccharification and Fermentation of Sugar Beet Pulp with Mixed Bacterial Cultures for Lactic Acid and Propylene Glycol Production. Molecules 21, 1380. doi:10.3390/molecules21101380
Beschkov, V., Razkazova-Velkova, E., and Liutzkanov, L. (2020). Carbon Dioxide Recycling for Fuels and Chemical Products. Prog. Petrochemical Sci. 3 (5), 376–379. doi:10.31031/pps.2020.03.000572
Bhatia, S. K., Jagtap, S. S., Bedekar, A. A., Bhatia, R. K., Patel, A. K., Pant, D., et al. (2020). Recent Developments in Pretreatment Technologies on Lignocellulosic Biomass: Effect of Key Parameters, Technological Improvements, and Challenges. Bioresour. Technol. 300, 122724. doi:10.1016/j.biortech.2019.122724
Bio-ethanol market-growth, trends, covid-19 impact, and forecasts (2021 - 2026) (2021). Bio-ethanol Market-Growth, Trends, Covid-19 Impact, and Forecasts (2021 - 2026). Available at: https://www.mordorintelligence.com/industry-reports/bio-ethanol-market (Accessed October 27, 2021).
Börner, R. A., Kandasamy, V., Axelsen, A. M., Nielsen, A. T., and Bosma, E. F. (2019). Genome Editing of Lactic Acid Bacteria: Opportunities for Food, Feed, Pharma and Biotech. FEMS Microbiol. Lett. 366, fny291. doi:10.1093/femsle/fny291
Brock, S., Kuenz, A., and Prüße, U. (2019). Impact of Hydrolysis Methods on the Utilization of Agricultural Residues as Nutrient Source for D-Lactic Acid Production by Sporolactobacillus Inulinus. Fermentation 5 (12), 5010012. doi:10.3390/fermentation5010012
Bustamante, D., Tortajada, M., Ramón, D., and Rojas, A. (2020). Production of D-Lactic Acid by the Fermentation of Orange Peel Waste Hydrolysate by Lactic Acid Bacteria. Fermentation 6, 1.
Carvalheiro, F., Esteves, M. P., Parajo, J. C., Pereira, H., and Girio, F. M. (2004). Production of Oligosaccharides by Autohydrolysis of Brewery's Spent Grain. Bioresour. Technol. 91 (1), 93–100. doi:10.1016/s0960-8524(03)00148-2
Chai, C. Y., Tan, I. S., Foo, H. C. Y., Lam, M. K., Tong, K. T. X., and Lee, K. T. (2021). Sustainable and green Pretreatment Strategy of Eucheuma Denticulatum Residues for Third-Generation L-Lactic Acid Production. Bioresour. Technol. 330, 124930. doi:10.1016/j.biortech.2021.124930
Chen, H., Chen, B., Su, Z., Wang, K., Wang, B., Wang, Y., et al. (2020). Efficient Lactic Acid Production from Cassava Bagasse by Mixed Culture of Bacillus Coagulans and Lactobacillus Rhamnosus Using Stepwise pH Controlled Simultaneous Saccharification and Co-fermentation. Ind. Crops Prod. 146, 112175. doi:10.1016/j.indcrop.2020.112175
Chen, H., Huo, W., Wang, B., Wang, Y., Wen, H., Cai, D., et al. (2019). L-lactic Acid Production by Simultaneous Saccharification and Fermentation of Dilute Ethylediamine Pre-treated rice Straw. Ind. Crops Prod. 141, 111749. doi:10.1016/j.indcrop.2019.111749
Chen, H., Su, Z., Wang, Y., Wang, B., Si, Z., Lu, J., et al. (2020). Lactic Acid Production from Pretreated Corn stover with Recycled Streams. Process Biochem. 91, 132–140. doi:10.1016/j.procbio.2019.12.004
Chen, P.-T., Hong, Z.-S., Cheng, C.-L., Ng, I.-S., Lo, Y.-C., Nagarajan, D., et al. (2020). Exploring Fermentation Strategies for Enhanced Lactic Acid Production with Polyvinyl Alcohol-Immobilized Lactobacillus Plantarum 23 Using Microalgae as Feedstock. Bioresour. Technol. 308, 123266. doi:10.1016/j.biortech.2020.123266
Cheng, Q., Shi, X., Liu, Y., Liu, X., Dou, S., Ning, C., et al. (2018). Production of Nisin and Lactic Acid from Corn stover through Simultaneous Saccharification and Fermentation. Biotechnol. Biotechnological Equipment 32 (2), 420–426. doi:10.1080/13102818.2017.1420425
Chin, S. X., Tasirin, S. M., Chan, C. H., Chia, C. H., Chook, S. W., Zakaria, S., et al. (2016). Catalytic Conversion of Empty Fruit Bunch (EFB) Fibres into Lactic Acid by Lead (II) Ions. BioResources 11 (1), 2186–2201. doi:10.15376/biores.11.1.2186-2201
Cho, S. W., Yim, J., and Seo, S. W. (2020). Engineering Tools for the Development of Recombinant Lactic Acid Bacteria. Biotechnol. J. 15, 1900344. doi:10.1002/biot.201900344
Cola, P., Procópio, D. P., Alves, A. T. d. C., Carnevalli, L. R., Sampaio, I. V., da Costa, B. L. V., et al. (2020). Differential Effects of Major Inhibitory Compounds from Sugarcane-Based Lignocellulosic Hydrolysates on the Physiology of Yeast Strains and Lactic Acid Bacteria. Biotechnol. Lett. 42, 571–582. doi:10.1007/s10529-020-02803-6
Conway, J. (2021). Global Beer Production 1998-2020. October 15. Available at: https://www.statista.com/statistics/270275/worldwide-beer-production/.
Cruz-Lopes, L., Domingos, I., Ferreira, J., and Esteves, B. (2017). J. Int. Scientific Publications 5, 85–93.
Cubas-Cano, E., González-Fernández, C., Ballesteros, M., and Tomás-Pejó, E. (2018). Biotechnological Advances in Lactic Acid Production by Lactic Acid Bacteria: Lignocellulose as Novel Substrate. Biofuels, Bioprod. Bioref. 12 (2), 290–303. doi:10.1002/bbb.1852
Cubas-Cano, E., González-Fernández, C., Ballesteros, M., and Tomás-Pejó, E. (2019). Lactobacillus Pentosus CECT 4023 T Co-utilizes Glucose and Xylose to Produce Lactic Acid from Wheat Straw Hydrolysate: Anaerobiosis as a Key Factor. Biotechnol. Prog. 35 (1), e2739. doi:10.1002/btpr.2739
Cubas-Cano, E., González-Fernández, C., and Tomás-Pejó, E. (2019). Evolutionary Engineering of Lactobacillus Pentosus Improves Lactic Acid Productivity from Xylose-Rich media at Low pH. Bioresour. Technol. 288, 121540. doi:10.1016/j.biortech.2019.121540
Cubas-Cano, E., Venus, J., González-Fernández, C., and Tomás-Pejó, E. (2020). Assessment of Different Bacillus Coagulans Strains for L-Lactic Acid Production from Defined media and Gardening Hydrolysates: Effect of Lignocellulosic Inhibitors. J. Biotechnol. 323, 9–16. doi:10.1016/j.jbiotec.2020.07.017
Damyanova, S., and Beschkov, V. (2020). “Biogas as a Source of Energy and Products,” in Biorefinery Concepts, Energy and Products (London: InthechOpen Ltd), 17–30.
de la Torre, I., Acedos, M. G., Ladero, M., and Santos, V. E. (2019). On the Use of Resting L. Delbrueckii Spp. Delbrueckii Cells for D-Lactic Acid Production from orange Peel Wastes Hydrolysates. Biochem. Eng. J. 145, 162–169. doi:10.1016/j.bej.2019.02.012
de la Torre, I., Ladero, M., and Santos, V. E. (2020). D-lactic Acid Production from orange Waste Enzymatic Hydrolysates with L. Delbrueckii Cells in Growing and Resting State. Ind. Crops Prod. 146, 112176. doi:10.1016/j.indcrop.2020.112176
de la Torre, I., Ravelo, M., Segarra, S., Tortajada, M., Santos, V. E., and Ladero, M. (2017). Study on the Effects of Several Operational Variables on the Enzymatic Batch Saccharification of orange Solid Waste. Bioresour. Technol. 245, 906–915. doi:10.1016/j.biortech.2017.08.094
de Oliveira Moraes, A., Ramirez, N. I. B., and Pereira, N. (2016). Evaluation of the Fermentation Potential of Pulp Mill Residue to Produce D(−)-Lactic Acid by Separate Hydrolysis and Fermentation Using Lactobacillus Coryniformis Subsp. Torquens. Appl. Biochem. Biotechnol. 180, 1574–1585. doi:10.1007/s12010-016-2188-3
De Oliveira, R. A., Alexandri, M., Komesu, A., Venus, J., Vaz Rossell, C. E., and Maciel Filho, R. (2020). Current Advances in Separation and Purification of Second-Generation Lactic Acid. Separat. Purif. Rev. 49 (2), 159–175. doi:10.1080/15422119.2019.1590412
Demmelmayer, P., and Kienberger, M. (2022). Reactive Extraction of Lactic Acid from Sweet Sorghum Silage Press Juice. Separat. Purif. Technol. 282, 120090. doi:10.1016/j.seppur.2021.120090
Dhandapani, B., Vishnu, D., Murshid, S., A, R. P., R, M., D, P., et al. (2021). Production of Lactic Acid from Industrial Waste Paper Sludge Using Rhizopus Oryzae MTCC5384 by Simultaneous Saccharification and Fermentation. Chem. Eng. Commun. 208 (6), 822–830. doi:10.1080/00986445.2019.1657422
Din, N. A. S., Lim, S. J., Maskat, M. Y., Mutalib, S. A., and Zaini, N. A. M. (2021). Lactic Acid Separation and Recovery from Fermentation Broth by Ion-Exchange Resin: A Review. Bioresour. Bioproc. 8, 31. doi:10.1186/s40643-021-00384-4
Djukic-Vukovic, A., Mladenovic, D., Radosavljevic, M., Kocic-Tanackov, S., Pejin, J., and Mojovic, L. (2016). Wastes from Bioethanol and Beer Productions as Substrates for L(+) Lactic Acid Production – A Comparative Study. Waste Manage. 48, 478–482.
Duque, A., Manzanares, P., Ballesteros, I., and Ballesteros, M. (2016). “Steam Explosion as Lignocellulosic Biomass Pretreatment,” in Biomass Fractionation Technologies for a Lignocellulosic Feedstock-Based Biorefinery. Editor S. I. Mussatto (Amsterdam: Elsevier), 349–368. doi:10.1016/b978-0-12-802323-5.00015-3
Erliana, W. H., Widjaja, T., Altway, A., and Pudjiastuti, L. (2020). Synthesis of Lactic Acid from Sugar palm Trunk Waste (Arenga Pinnata): Preliminary Hydrolysis and Fermentation Studies. Biodiversitas 21 (5), 2281–2288. doi:10.13057/biodiv/d210559
Esquivel-Hernández, D. E., Pennacchio, A., Torres-Acosta, M. A., Parra-Saldívar, R., de Souza Vandenberghe, L. P., and Faraco, V. (2021). Multi-product Biorefinery from Arthrospira Platensis Biomass as Feedstock for Bioethanol and Lactic Acid Production. Scientific Rep. 11, 19309.
Garrett, B. G., Srinivas, K., and Ahring, B. K. (2015). Performance and Stability of Amberlite IRA-67 Ion Exchange Resin for Product Extraction and pH Control during Homolactic Fermentation of Corn stover Sugars. Biochem. Eng. J. 94, 1–8. doi:10.1016/j.bej.2014.11.004
Gezae Daful, A., and Görgens, J. F. (2017). Techno-economic Analysis and Environmental Impact Assessment of Lignocellulosic Lactic Acid Production. Chem. Eng. Sci. 162, 53–65. doi:10.1016/j.ces.2016.12.054
Giacon, T. G., Cunha, G. G. G., Eliodório, K. P., Oliveira, R. P. S., and Basso, T. O. (2021). Homo-and Heterofermentative Lactobacilli Are Differently Affected by Lignocellulosic Inhibitory Compounds. bioRxiv 2021.01.18.427060. doi:10.1101/2021.01.18.427060
Gilver Rosero Chasoy, G. R., Chairez, I., and Durán-Páramo, E. (2020). Carbon/nitrogen Ratio and Initial pH Effects on the Optimization of Lactic Acid Production by. Wulfenia J. 27 (10), 37–59.
Girotto, F., Pivato, A., Cossu, R., Nkeng, G. E., and Lavagnolo, M. C. (2018). The Broad Spectrum of Possibilities for Spent Coffee Grounds Valorisation. J. Mater. Cycles Waste Manag. 20, 695–701. doi:10.1007/s10163-017-0621-5
Givry, S., Prevot, V., and Duchiron, F. (2008). Lactic Acid Production from Hemicellulosic Hydrolyzate by Cells of Lactobacillus Bifermentans Immobilized in Ca-Alginate Using Response Surface Methodology. World J. Microbiol. Biotechnol. 24, 745–752. doi:10.1007/s11274-007-9534-0
Global Lactic Acid Market 2017-2025 - Growth Trends, Key Players, Competitive Strategies and Forecasts - Research and Market (2017). Global Lactic Acid Market 2017-2025 - Growth Trends, Key Players, Competitive Strategies and Forecasts - Research and Market. Available at: https://www.businesswire.com/news/home/20170621005594/en/Global-Lactic-Acid-Market-2017-2025---Growth-Trends-Key-Players-Competitive-Strategies-and-Forecasts---Research-and-Markets.
Goh, C. S., Tan, H. T., Lee, K. T., and Brosse, N. (2011). Evaluation and Optimization of Organosolv Pretreatment Using Combined Severity Factors and Response Surface Methodology. Biomass and Bioenergy 35 (9), 4025–4033. doi:10.1016/j.biombioe.2011.06.034
Gómez-Gómez, J. A., Giraldo-Estrada, C., Habeych, D., and Baena, S. (2015). Evaluation of Biological Production of Lactic Acid in a Synthetic Medium and in Aloe Vera (L.) Burm. F. Processing By-Products. Universitas Scientiarum 20 (3), 369–385.
González-Leos, A., Bustos-Vázquez, M. G., Rodríguez-Castillejos, G. C., Rodríguez-Durán, L. V., and Del Ángel-Del Ángel, A. (2020). Kinetics of Lactic Acid Fermentation from Sugarcane Bagasse by Lactobacillus Pentosus. Revista Mexicana de Ingeniería Química 19 (1), 377–386.
Gössi, A., Burgener, F., Kohler, D., Urso, A., Kolvenbach, B. A., Riedl, W., et al. (2020). In-situ Recovery of Carboxylic Acids from Fermentation Broths through Membrane Supported Reactive Extraction Using Membrane Modules with Improved Stability. Separat. Purif. Technol. 241, 116694.
Grewal, J., and Khare, S. K. (2018). One-pot Bioprocess for Lactic Acid Production from Lignocellulosic Agro-Wastes by Using Ionic Liquid Stable Lactobacillus Brevis. Bioresour. Technol. 251, 268–273. doi:10.1016/j.biortech.2017.12.056
Grewal, J., Sadaf, A., Yadav, N., and Khare, S. K. (2020). “Agroindustrial Waste Based Biorefineries for Sustainable Production of Lactic Acid,” in Waste Biorefinery: Integrating Biorefineries for Waste Valorisation. Editors T. Bhaskar, A. Pandey, E. Rene, and D. Tsang (Amsterdam, Netherlands: Elsevier), 125–153. doi:10.1016/b978-0-12-818228-4.00005-8
Haldar, D., and Purkait, M. K. (2020). Lignocellulosic Conversion into Value-Added Products: A Review. Process Biochem. 89, 110–133. doi:10.1016/j.procbio.2019.10.001
Halder, P., Kundu, S., Patel, S., Setiawan, A., Atkin, R., Parthasarthy, R., et al. (2019). Progress on the Pre-treatment of Lignocellulosic Biomass Employing Ionic Liquids. Renew. Sustain. Energ. Rev. 105, 268–292. doi:10.1016/j.rser.2019.01.052
Hama, S., Mizuno, S., Kihara, M., Tanaka, T., Ogino, C., Noda, H., et al. (2015). Production of D -lactic Acid from Hardwood Pulp by Mechanical Milling Followed by Simultaneous Saccharification and Fermentation Using Metabolically Engineered Lactobacillus Plantarum. Bioresour. Technol. 187, 167–172. doi:10.1016/j.biortech.2015.03.106
Han, X., Dong, W., and Bao, J. (2021). Upgrading Steam Pretreatment by Converting Water-Soluble Carbohydrates into Lactic Acid Prior to Pretreatment. Biomass Conv. Bioref. doi:10.1007/s13399-020-01183-1
Han, X., Hong, F., Liu, G., and Bao, J. (2018). An Approach of Utilizing Water-Soluble Carbohydrates in Lignocellulose Feedstock for Promotion of Cellulosic L-Lactic Acid Production. J. Agric. Food Chem. 66, 10225–10232. doi:10.1021/acs.jafc.8b03592
Han, X., Li, L., Wei, C., Zhang, J., and Bao, J. (2019). Facilitation of L-Lactic Acid Fermentation by Lignocellulose Biomass Rich in Vitamin B Compounds. J. Agric. Food Chem. 67, 7082–7086. doi:10.1021/acs.jafc.9b02297
He, T., Jiang, Z., Wu, P., Yi, J., Li, J., and Hu, C. (2016). Fractionation for Further Conversion: from Raw Corn stover to Lactic Acid. Sci. Rep. 6, 38623. doi:10.1038/srep38623
He, Y.-C., Liu, F., Di, J.-H., Ding, Y., Zhu, Z.-Z., Wu, Y.-Q., et al. (2016). Effective Enzymatic Saccharification of Dilute NaOH Extraction of Chestnut Shell Pretreated by Acidified Aqueous Ethylene Glycol media. Ind. Crops Prod. 81, 129–138. doi:10.1016/j.indcrop.2015.11.079
He, Y.-C., Liu, F., Gong, L., Di, J.-H., Ding, Y., Ma, C.-L., et al. (2016). Enzymatic In Situ Saccharification of Chestnut Shell with High Ionic Liquid-Tolerant Cellulases from Galactomyces Sp. CCZU11-1 in a Biocompatible Ionic Liquid-Cellulase media. Bioresour. Technol. 201, 133–139. doi:10.1016/j.biortech.2015.11.034
He, Y., Ding, Y., Ma, C., Di, J., Jiang, C., and Li, A. (2017). One-pot Conversion of Biomass-Derived Xylose to Furfuralcohol by a Chemo-Enzymatic Sequential Acid-Catalyzed Dehydration and Bioreduction. Green. Chem. 19, 3844–3850. doi:10.1039/c7gc01256j
Hoheneder, R., Fitz, E., Bischof, R. H., Russmayer, H., Ferrero, P., Peacock, S., et al. (2021). Efficient Conversion of Hemicellulose Sugars from Spent Sulfite Liquor into Optically Pure L-Lactic Acid by Enterococcus Mundtii. Bioresour. Technol. 333, 125215. doi:10.1016/j.biortech.2021.125215
Hu, J., Lin, Y., Zhang, Z., Xiang, T., Mei, Y., Zhao, S., et al. (2016). High-titer Lactic Acid Production by Lactobacillus Pentosus FL0421 from Corn stover Using Fed-Batch Simultaneous Saccharification and Fermentation. Bioresour. Technol. 214, 74–80. doi:10.1016/j.biortech.2016.04.034
Hu, Y., Kwan, T. H., Daoud, W. A., and Lin, C. S. K. (2017). Continuous Ultrasonic-Mediated Solvent Extraction of Lactic Acid from Fermentation Broths. J. Clean. Prod. 145, 142–150. doi:10.1016/j.jclepro.2017.01.055
Huang, C., Liu, J., Geng, W., Tang, W., and Yong, Q. (2021). A Review of Lignocellulosic Biomass Pretreatment Technologies. Paper Biomater. 6 (3), 61–76.
Hudeckova, H., Neureiter, M., Obruca, S., Frühauf, S., and Marova, I. (2018). Biotechnological Conversion of Spent Coffee Grounds into Lactic Acid. Lett. Appl. Microbiol. 66, 306–312. doi:10.1111/lam.12849
Hwang, H. J., Kim, S. M., Chang, J. H., and Lee, S. B. (2012). Lactic Acid Production from Seaweed Hydrolysate of Enteromorpha Prolifera (Chlorophyta). J. Appl. Phycol 24, 935–940. doi:10.1007/s10811-011-9714-z
Jacques, K. A., Lyons, T. P., and Kelsall, D. R. (2003). The Alcohol Textbook. 4th edition. Nottingham: Nottingham University Press.
Jaichakan, P., Nakphaichit, M., Rungchang, S., Weerawatanakorn, M., Phongthai, S., and Klangpetch, W. (2021). Two-stage Processing for Xylooligosaccharide Recovery from rice By-Products and Evaluation of Products: Promotion of Lactic Acid-Producing Bacterial Growth and Food Application in a High-Pressure Process. Food Res. Int. 147, 110529. doi:10.1016/j.foodres.2021.110529
Jiang, S., Xu, P., and Tao, F. (2019). L-lactic Acid Production by Bacillus Coagulans through Simultaneous Saccharification and Fermentation of Lignocellulosic Corncob Residue. Bioresour. Technol. Rep. 6, 131–137. doi:10.1016/j.biteb.2019.02.005
Jiang, T., Qiao, H., Zheng, Z., Chu, Q., Li, X., Yong, Q., et al. (2016). Lactic Acid Production from Pretreated Hydrolysates of Corn Stover by a Newly Developed Bacillus Coagulans Strain. PLoS ONE 11 (2), e0149101. doi:10.1371/journal.pone.0149101
Juturu, V., and Wu, J. C. (2016). Microbial Production of Lactic Acid: the Latest Development. Crit. Rev. Biotechnol. 36 (6), 967–977. doi:10.3109/07388551.2015.1066305
Kim, J.-w., Jang, J. H., Yeo, H. J., Seol, J., Kim, S. R., and Jung, Y. H. (2019). Lactic Acid Production from a Whole Slurry of Acid-Pretreated Spent Coffee Grounds by Engineered Saccharomyces cerevisiae. Appl. Biochem. Biotechnol. 189, 206–216. doi:10.1007/s12010-019-03000-6
Kong, X., Zhang, B., Hua, Y., Zhu, Y., Li, W., Wang, D., et al. (2019). Efficient L-Lactic Acid Production from Corncob Residue Using Metabolically Engineered Thermo-Tolerant Yeast. Bioresour. Technol. 273, 220–230. doi:10.1016/j.biortech.2018.11.018
Koo, J.-R., Min Park, H., Kyung Kim, S., and Shik Yun, H. (2019). Lactic Acid Fermentation from Coffee Ground Waste Hydrolysate by Lactobacillus Rhamnosus. J. Renew. Mater. 7 (4), 365–372. doi:10.32604/jrm.2019.04170
Krishna, B. S., Nikhilesh, G. S. S., Tarun, B., Narayana, K. V. S., and Gopinadh, R. (2018). Industrial Production of Lactic Acid and its Applications. Int. J. Biotech Res. 1 (1), 42–54.
Krull, S., Brock, S., Prüße, U., and Kuenz, A. (2020). Hydrolyzed Agricultural Residues-Low-Cost Nutrient Sources for L-Lactic Acid Production. Fermentation 6, 97. doi:10.3390/fermentation6040097
Kumar, A., and Chandra, R. (2020). Ligninolytic Enzymes and its Mechanisms for Degradation of Lignocellulosic Waste in Environment. Heliyon 6, e03170. doi:10.1016/j.heliyon.2020.e03170
Kumar, A., Thakur, A., and Panesar, P. S. (2019). Lactic Acid and its Separation and Purification Techniques: A Review. Rev. Environ. Sci. Biotechnol. 18, 823–853. doi:10.1007/s11157-019-09517-w
Kumar, V., Yadav, S. K., Kumar, J., and Ahluwalia, V. (2020). A Critical Review on Current Strategies and Trends Employed for Removal of Inhibitors and Toxic Materials Generated during Biomass Pretreatment. Bioresour. Technol. 299, 122633. doi:10.1016/j.biortech.2019.122633
Kunasundari, B., Arai, T., Sudesh, K., Hashim, R., Sulaiman, O., Stalin, N. J., et al. (2017). Detoxification of Sap from Felled Oil Palm Trunks for the Efficient Production of Lactic Acid. Appl. Biochem. Biotechnol. 183, 412–425. doi:10.1007/s12010-017-2454-z
Kuo, Y.-C., Yuan, S.-F., Wang, C.-A., Huang, Y.-J., Guo, G.-L., and Hwang, W.-S. (2015). Production of Optically Pure L -lactic Acid from Lignocellulosic Hydrolysate by Using a Newly Isolated and D -lactate Dehydrogenase Gene-Deficient Lactobacillus Paracasei Strain. Bioresour. Technol. 198, 651–657. doi:10.1016/j.biortech.2015.09.071
Laca, A., Laca, A., and Díaz, M. (2019). “Hydrolysis: From Cellulose and Hemicellulose to Simple Sugars,” in Second and Third Generation of Feedstocks (Amsterdam: Elsevier), 213–240. doi:10.1016/b978-0-12-815162-4.00008-2
Lactic Acid Market by Application (Biodegradable Polymers, Food & Beverages, Pharmaceutical Products) (2020). Lactic Acid Market by Application (Biodegradable Polymers, Food & Beverages, Pharmaceutical Products). Available at: https://www.marketsandmarkets.com/Market-Reports/polylacticacid-387.html.
Lan, K., Xu, S., Li, J., and Hu, C. (2019). Recovery of Lactic Acid from Corn Stover Hemicellulose-Derived Liquor. ACS Omega 4, 10571–10579. doi:10.1021/acsomega.9b00794
Lee, K. H., Jang, Y. W., Lee, J., Kim, S., Park, C., and Yoo, H. Y. (2021). Statistical Optimization of Alkali Pretreatment to Improve Sugars Recovery from Spent Coffee Grounds and Utilization in Lactic Acid Fermentation. Processes 9, 494. doi:10.3390/pr9030494
Li, C., Gao, M., Zhu, W., Wang, N., Ma, X., Wu, C., et al. (2021). Recent Advances in the Separation and Purification of Lactic Acid from Fermentation Broth. Process Biochem. 104, 142–151. doi:10.1016/j.procbio.2021.03.011
Li, J., Shi, S., Wang, Y., and Jiang, Z. (2021). Integrated Production of Optically Pure L-Lactic Acid from Paper Mill Sludge by Simultaneous Saccharification and Co-fermentation (SSCF). Waste Manage. 129, 35–46. doi:10.1016/j.wasman.2021.05.008
Li, Q., Ma, C.-L., Zhang, P.-Q., Li, Y.-Y., Zhu, X., and He, Y.-C. (2021). Effective Conversion of Sugarcane Bagasse to Furfural by Coconut Shell Activated Carbon-Based Solid Acid for Enhancing Whole-Cell Biosynthesis of Furfurylamine. Ind. Crops Prod. 160, 113169. doi:10.1016/j.indcrop.2020.113169
Li, Y., Bhagwat, S. S., Cortés-Peña, Y. R., Ki, D., Rao, C. V., Jin, Y.-S., et al. (2021). Sustainable Lactic Acid Production from Lignocellulosic Biomass. ACS Sustain. Chem. Eng. 9, 1341–1351. doi:10.1021/acssuschemeng.0c08055
Lin, H.-T. V., Huang, M.-Y., Kao, T.-Y., Lu, W.-J., Lin, H.-J., and Pan, C.-L. (2020). Production of Lactic Acid from Seaweed Hydrolysates via Lactic Acid Bacteria Fermentation. Fermentation 6 (1), 37. doi:10.3390/fermentation6010037
Lin, Q., Yan, Y., Liu, X., He, B., Wang, X., Wang, X., et al. (2020). Production of Xylooligosaccharide, Nanolignin, and Nanocellulose through a Fractionation Strategy of Corncob for Biomass Valorization. Ind. Eng. Chem. Res. 59, 17429–17439. doi:10.1021/acs.iecr.0c02161
Liu, J., Cai, Y., Liu, L., Zhan, R., Zhao, S., Liang, Y., et al. (2020). Enhanced Lactic Acid Production byBacillus Coagulansthrough Simultaneous Saccharification, Biodetoxification, and Fermentation. Biofuels, Bioprod. Bioref. 14, 533–543. doi:10.1002/bbb.2086
Liu, L., Cai, Y., Li, H., Zhao, S., He, M., Hu, G.-q., et al. (2019). Bio-detoxification Bacteria Isolated from Dye-Polluted Soils Promote Lactic Acid Production from Ammonia Pretreated Corn Stover. Appl. Biochem. Biotechnol. 189, 129–143. doi:10.1007/s12010-019-02993-4
Liu, Y., Ashok, S., Seol, E., Bao, J., and Park, S. (2013). Comparison of Three Pediococcus Strains for Lactic Acid Production from Glucose in the Presence of Inhibitors Generated by Acid Hydrolysis of Lignocellulosic Biomass. Biotechnol. Bioproc. E 18, 1192–1200. doi:10.1007/s12257-013-0360-y
Liu, Y., Zheng, J., Xiao, J., He, X., Zhang, K., Yuan, S., et al. (2019). Enhanced Enzymatic Hydrolysis and Lignin Extraction of Wheat Straw by Triethylbenzyl Ammonium Chloride/Lactic Acid-Based Deep Eutectic Solvent Pretreatment. ACS Omega 4 (22), 19829–19839. doi:10.1021/acsomega.9b02709
Loow, Y.-L., Wu, T. Y., Yang, G. H., Ang, L. Y., New, E. K., Siow, L. F., et al. (2018). Deep Eutectic Solvent and Inorganic Salt Pretreatment of Lignocellulosic Biomass for Improving Xylose Recovery. Bioresour. Technol. 249, 818–825. doi:10.1016/j.biortech.2017.07.165
López-Gómez, J. P., Pérez-Rivero, C., and Venus, J. (2020). Valorisation of Solid Biowastes: The Lactic Acid Alternative. Process Biochem. 99, 222–235. doi:10.1016/j.procbio.2020.08.029
Lu, S., Wang, Q., Liang, Z., Wang, W., Liang, C., Wang, Z., et al. (2021). Saccharification of Sugarcane Bagasse by Magnetic Carbon-Based Solid Acid Pretreatment and Enzymatic Hydrolysis. Ind. Crops Prod. 160, 113159. doi:10.1016/j.indcrop.2020.113159
Ludwig, D., Amann, M., Hirth, T., Rupp, S., and Zibek, S. (2013). Development and Optimization of Single and Combined Detoxification Processes to Improve the Fermentability of Lignocellulose Hydrolyzates. Bioresour. Technol. 133, 455–461. doi:10.1016/j.biortech.2013.01.053
Luo, H., Gao, L., Liu, Z., Shi, Y., Xie, F., Bilal, M., et al. (2021). Prediction of Phenolic Compounds and Glucose Content from Dilute Inorganic Acid Pretreatment of Lignocellulosic Biomass Using Artificial Neural Network Modeling. Bioresour. Bioproc. 8, 134. doi:10.1186/s40643-021-00488-x
Ma, K., Hu, G., Pan, L., Wang, Z., Zhou, Y., Wang, Y., et al. (2016). Highly Efficient Production of Optically Pure L-Lactic Acid from Corn stover Hydrolysate by Thermophilic Bacillus Coagulans. Bioresour. Technol. 219, 114–122. doi:10.1016/j.biortech.2016.07.100
Ma, X., Gao, M., Yin, Z., Zhu, W., Liu, S., and Wang, Q. (2020). Lactic Acid and Animal Feeds Production from Sophora Flavescens Residues by Rhizopus Oryzae Fermentation. Process Biochem. 92, 401–408. doi:10.1016/j.procbio.2020.01.030
Manandhar, A., and Shah, A. (2020). Techno-Economic Analysis of Bio-Based Lactic Acid Production Utilizing Corn Grain as Feedstock. Processes 8, 199. doi:10.3390/pr8020199
Mandegari, M. A., Farzad, S., van Rensburg, E., and Görgens, J. F. (2017). Multi‐criteria Analysis of a Biorefinery for Co‐production of Lactic Acid and Ethanol from Sugarcane Lignocellulose. Biofuels, Bioprod. Bioref. 11 (6), 971–990. doi:10.1002/bbb.1801
Mankar, A. R., Pandey, A., Modak, A., and Pant, K. K. (2021). Pretreatment of Lignocellulosic Biomass: A Review on Recent Advances. Bioresour. Technol. 334, 125235. doi:10.1016/j.biortech.2021.125235
Marin-Batista, J. D., Mohedano, A. F., and de la Rubia, A. (2021). Pretreatment of Lignocellulosic Biomass with 1-Ethyl-3-Methylimidazolium Acetate for its Eventual Valorization by Anaerobic Digestion. Resources 10, 118. doi:10.3390/resources10120118
Marques, S., Gírio, F. M., Santos, J. A. L., and Roseiro, J. C. (2017). Pulsed Fed-Batch Strategy towards Intensified Process for Lactic Acid Production Using Recycled Paper Sludge. Biomass Conv. Bioref. 7, 127–137. doi:10.1007/s13399-016-0211-0
Martínez-Trujillo, M. A., Bautista-Rangel, K., García-Rivero, M., Martínez-Estrada, A., and Cruz-Díaz, M. R. (2020). Enzymatic Saccharification of Banana Peel and Sequential Fermentation of the Reducing Sugars to Produce Lactic Acid. Bioproc. Biosyst. Eng. 43, 413–427.
Marzo, C., Díaz, A. B., Caro, I., and Blandino, A. (2021). Effect of Several Pretreatments on the Lactic Acid Production from Exhausted Sugar Beet Pulp. Foods 10, 2414. doi:10.3390/foods10102414
Mazzoli, R. (2020). Metabolic Engineering Strategies for Consolidated Production of Lactic Acid from Lignocellulosic Biomass. Biotechnol. Appl. Biochem. 67 (1), 61–72. doi:10.1002/bab.1869
Mäki-Arvela, P., Simakova, I. L., Salmi, T., and Murzin, D. Yu. (2014). Production of Lactic Acid/Lactates from Biomass and Their Catalytic Transformations to Commodities. Chem. Rev. 114, 1909–1971.
McCutcheon, J., and Samples, D. (2002). Grazing Corn Residue. Columbus, OH ANR-10-02: The Ohio State University Extension. Available at: https://www.agrireseau.net/bovinsboucherie/documents/0010[1].pdf.
Mejia-Gomez, C. E., and Balcázar, N. (2020). Isolation, Characterisation and Continuous Culture of Lactobacillus Spp. And its Potential Use for Lactic Acid Production from Whey. Food Sci. Technol. 40 (4), 1021–1028. doi:10.1590/fst.29619
Meneses, N. G. T., Martins, S., Teixeira, J. A., and Mussatto, S. I. (2013). Influence of Extraction Solvents on the Recovery of Antioxidant Phenolic Compounds from brewer's Spent Grains. Separat. Purif. Technol. 108, 152–158. doi:10.1016/j.seppur.2013.02.015
Mikulski, D., and Kłosowski, G. (2018). Efficiency of Dilute Sulfuric Acid Pretreatment of Distillery Stillage in the Production of Cellulosic Ethanol. Bioresour. Technol. 268, 424–433. doi:10.1016/j.biortech.2018.08.005
Mladenovic, D., Djukic-Vukovic, A., Stankovic, M., Milašinovic-Šeremešic, M., Radosavljevic, M., Pejin, J., et al. (2019). Bioprocessing of Agro-Industrial Residues into Lactic Acid and Probiotic Enriched Livestock Feed. J. Sci. Food Agric. 99, 5293–5302.
Mladenović, D., Pejin, J., Kocić-Tanackov, S., Radovanović, Ž., Djukić-Vuković, A., and Mojović, L. (2018). Lactic Acid Production on Molasses Enriched Potato Stillage by Lactobacillus Paracasei Immobilized onto Agro-Industrial Waste Supports. Ind. Crops Prod. 124, 142–148.
Montipó, S., Pedroso, G. B., Bevilaqua, D. B., Prestes, O. D., Corona-González, R. I., and Martins, A. F. (2016). Building Block Lactic Acid from Rice Husks and Agave Bagasse. Waste Biomass Valor. 7, 1495–1507. doi:10.1007/s12649-016-9554-9
Mussatto, S. I., Machado, E. M. S., Martins, S., and Teixeira, J. A. (2011). Production, Composition, and Application of Coffee and its Industrial Residues. Food Bioproc. Technol 4, 661–672. doi:10.1007/s11947-011-0565-z
Mussatto, S. I., and Roberto, I. C. (2006). Chemical Characterization and Liberation of Pentose Sugars from brewer's Spent Grain. J. Chem. Technol. Biotechnol. 81, 268–274. doi:10.1002/jctb.1374
Nagarajan, D., Nandini, A., Dong, C.-D., Lee, D.-J., and Chang, J.-S. (2020). Lactic Acid Production from Renewable Feedstocks Using Poly(vinyl Alcohol)-Immobilized Lactobacillus Plantarum 23. Ind. Eng. Chem. Res. 59, 17156–17164. doi:10.1021/acs.iecr.0c01422
Nagarajan, D., Oktarina, N., Chen, P.-T., Chen, C.-Y., Lee, D.-J., and Chang, J.-S. (2022). Fermentative Lactic Acid Production from Seaweed Hydrolysate Using Lactobacillus Sp. And Weissella Sp. Bioresour. Technol. 344, 126166. doi:10.1016/j.biortech.2021.126166
Nalawade, K., Baral, P., Patil, S., Pundir, A., Kurmi, A. K., Konde, K., et al. (2020). Evaluation of Alternative Strategies for Generating Fermentable Sugars from High-Solids Alkali Pretreated Sugarcane Bagasse and Successive Valorization to L (+) Lactic Acid. Renew. Energ. 157, 708–717. doi:10.1016/j.renene.2020.05.089
Nandini, A., Nagarajan, D., and Chang, J.-S. (2020). Production of Lactic Acid from Microalgal Biomass Chlorella Vulgar ESP-31 as a Feedstock Using PVA Immobilized Bacteria L. Plantarum 23. NST Proc. 2020, 165–169. doi:10.11594/nstp.2020.0525
Neu, A.-K., Pleissner, D., Mehlmann, K., Schneider, R., Puerta-Quintero, G. I., and Venus, J. (2016). Fermentative Utilization of Coffee Mucilage Using Bacillus Coagulans and Investigation of Down-Stream Processing of Fermentation Broth for Optically Pure L(+)-lactic Acid Production. Bioresour. Technol. 211, 398–405. doi:10.1016/j.biortech.2016.03.122
Norrrahim, M. N. F., Ilyas, R. A., Nurazzi, N. M., Rani, M. S. A., Atikah, M. S. N., and a Shazleen, S. S. (2021). Chemical Pretreatment of Lignocellulosic Biomass for the Production of Bioproducts: An Overview. Appl. Sci. Eng. Prog. 14 (4), 588–605. doi:10.14416/j.asep.2021.07.004
Nwamba, M. C., Sun, F., Mukasekuru, M. R., Song, G., Harindintwali, J. D., Boyi, S. A., et al. (2021). Trends and Hassles in the Microbial Production of Lactic Acid from Lignocellulosic Biomass. Environ. Technol. Innovation 21, 101337. doi:10.1016/j.eti.2020.101337
Okano, K., Zhang, Q., Yoshida, S., Tanaka, T., Ogino, C., Fukuda, H., et al. (2010). D-Lactic Acid Production from Cellooligosaccharides and β-glucan Using L-LDH Gene-Deficient and Endoglucanase-Secreting Lactobacillus Plantarum. Appl. Microbiol. Biotechnol. 85, 643–650. doi:10.1007/s00253-009-2111-8
Olszewska-Widdrat, A., Alexandri, M., López-Gómez, J. P., Schneider, R., Mandl, M., and Venus, J. (2019). Production and Purification of L-Lactic Acid in Lab and Pilot Scales Using Sweet Sorghum Juice. Fermentation 5 (2), 36. doi:10.3390/fermentation5020036
Oonkhanond, B., Jonglertjunya, W., Srimarut, N., Bunpachart, P., Tantinukul, S., Nasongkla, N., et al. (2017). Lactic Acid Production from Sugarcane Bagasse by an Integrated System of Lignocellulose Fractionation, Saccharification, Fermentation, and Ex-Situ Nanofiltration. J. Environ. Chem. Eng. 5, 2533–2541. doi:10.1016/j.jece.2017.05.004
Othman, M., Ariff, A. B., Kapri, M. R., Rios-Solis, L., and Halim, M. (2018). Growth Enhancement of Probiotic Pediococcus Acidilactici by Extractive Fermentation of Lactic Acid Exploiting Anion-Exchange Resin. Front. Microbiol. 9, 2554. doi:10.3389/fmicb.2018.02554
Othman, M., Ariff, A. B., Rios-Solis, L., and Halim, M. (2017). Extractive Fermentation of Lactic Acid in Lactic Acid Bacteria Cultivation: A Review. Front. Microbiol. 8, 2285. doi:10.3389/fmicb.2017.02285
Ouyang, S., Zou, L., Qiao, H., Shi, J., Zheng, Z., and Ouyang, J. (2020). One-pot Process for Lactic Acid Production from Wheat Straw by an Adapted Bacillus Coagulans and Identification of Genes Related to Hydrolysate-Tolerance. Bioresour. Technol. 315, 123855. doi:10.1016/j.biortech.2020.123855
Pedro Silva, J., Sousa, S., Rodrigues, J., Antunes, H., Porter, J. J., Gonçalves, I., et al. (2004). Adsorption of Acid orange 7 Dye in Aqueous Solutions by Spent Brewery Grains. Separat. Purif. Technol. 40, 309–315. doi:10.1016/j.seppur.2004.03.010
Pejin, J., Radosavljević, M., Kocić-Tanackov, S., Djukić-Vuković, A., and Mojović, L. (2017). Lactic Acid Fermentation of brewer's Spent Grain Hydrolysate byLactobacillus Rhamnosuswith Yeast Extract Addition and pH Control. J. Inst. Brew. 123, 98–104. doi:10.1002/jib.403
Pin, T. C., Nakasu, P. Y. S., Mattedi, S., Rabelo, S. C., and Costa, A. C. (2019). Screening of Protic Ionic Liquids for Sugarcane Bagasse Pretreatment. Fuel 235, 1506–1514. doi:10.1016/j.fuel.2018.08.122
Plavec, T. V., and Berlec, A. (2020). Safety Aspects of Genetically Modified Lactic Acid Bacteria. Microorganisms 8, 297. doi:10.3390/microorganisms8020297
Pontes, R., Romaní, A., Michelin, M., Domingues, L., Teixeira, J., and Nunes, J. (2021). L-lactic Acid Production from Multi-Supply Autohydrolyzed Economically Unexploited Lignocellulosic Biomass. Ind. Crops Prod. 170, 113775. doi:10.1016/j.indcrop.2021.113775
Prado-Rubio, O. A., Gasca-González, R., Fontalvo, J., Gómez-Castro, F. I., Pérez-Cisneros, E. S., and Morales-Rodriguez, R. (2020). Design and Evaluation of Intensified Downstream Technologies towards Feasible Lactic Acid Bioproduction. Chem. Eng. Process. - Process Intensification 158, 108174. doi:10.1016/j.cep.2020.108174
Procentese, A., and Rehmann, L. (2018). Fermentable Sugar Production from a Coffee Processing By-Product after Deep Eutectic Solvent Pretreatment. Bioresour. Technol. Rep. 4, 174–180. doi:10.1016/j.biteb.2018.10.012
Puspita Aini, A., Lee, H. W., Parningotan Sitompul, J., and Rasrendra, C. B. (2018). Production of Lactic Acid from Empty Fruit Bunch of Palm Oil Using Catalyst of Barium Hydroxide. MATEC Web Conf. 156, 06004. doi:10.1051/matecconf/201815606004
Qin, L., Qian, H., and He, Y. (2017). Microbial Lipid Production from Enzymatic Hydrolysate of Pecan Nutshell Pretreated by Combined Pretreatment. Appl. Biochem. Biotechnol. 183 (4), 1336–1350. doi:10.1007/s12010-017-2501-9
Radosavljevic, M., Levic, S., Belovic, M., Pejin, J., Djukic-Vukovic, A., Mojovic, L., et al. (2021). Encapsulation of Lactobacillus Rhamnosus in Polyvinyl Alcohol for the Production of L-(+)-Lactic Acid. Process Biochem. 100, 149–160.
Radosavljevic, M., Pejin, J., Pribic, M., Kocic-Tanackov, S., Mladenovic, D., Djukic-Vukovic, A., et al. (2020). Brewing and Malting Technology By-Products as Raw Materials in L-(+)-lactic Acid Fermentation. J. Chem. Technol. Biotechnol. 95, 339–347.
Rahman, M. S., H. Mondal, M. I., Yeasmin, M. S. S., Sayeed, M. A., Hossain, M. A., and Ahmed, M. B. (2020). Conversion of Lignocellulosic Corn Agro-Waste into Cellulose Derivative and its Potential Application as Pharmaceutical Excipient. Processes 8, 711. doi:10.3390/pr8060711
Rapado, P., Faba, L., and Ordóñez, S. (2021). Influence of Delignification and Reaction Conditions in the Aqueous Phase Transformation of Lignocellulosic Biomass to Platform Molecules. Bioresour. Technol. 321, 124500. doi:10.1016/j.biortech.2020.124500
Rawoof, S. A. A., Kumar, P. S., Vo, D.-V. N., Devaraj, K., Mani, Y., Devaraj, T., et al. (2021). Production of Optically Pure Lactic Acid by Microbial Fermentation: a Review. Environ. Chem. Lett. 19, 539–556. doi:10.1007/s10311-020-01083-w
Redondo-Gómez, C., Quesada, M. R., Astúa, S. V., Zamora, J. P. M., Lopretti, M., and Vega-Baudrit, J. R. (2020). Biorefinery of Biomass of Agro-Industrial Banana Waste to Obtain High-Value Biopolymers. Molecules 25, 3829.
Reinold, M. R. (1997). Manual pratico de cervejaria. first edition. São Paulo: ADEN Editora e Comunicações Ltda.
Reshmy, R., Philip, E., Madhavan, A., Sirohi, R., Pugazhendhi, A., Binod, P., et al. (2022). Lignocellulose in Future Biorefineries: Strategies for Cost-Effective Production of Biomaterials and Bioenergy. Bioresour. Technol. 344, 126241. doi:10.1016/j.biortech.2021.126241
Rojas, M. J., Siqueira, P. F., Miranda, L. C., Tardioli, P. W., and Giordano, R. L. C. (2014). Sequential Proteolysis and Cellulolytic Hydrolysis of Soybean Hulls for Oligopeptides and Ethanol Production. Ind. Crops Prod. 61, 202–210. doi:10.1016/j.indcrop.2014.07.002
Rouches, E., Zhou, S., Steyer, J. P., and Carrere, H. (2016). White-rot Fungi Pretreatment of Lignocellulosic Biomass for Anaerobic Digestion: Impact of Glucose Supplementation. Process Biochem. 51, 1784–1792. doi:10.1016/j.procbio.2016.02.003
Sánchez, C., Egüés, I., García, A., Llano-Ponte, R., and Labidi, J. (2012). Lactic Acid Production by Alkaline Hydrothermal Treatment of Corn Cobs. Chem. Eng. J. 181–182, 655–660.
Schroedter, L., Streffer, F., Streffer, K., Unger, P., and Venus, J. (2021). Biorefinery Concept Employing Bacillus Coagulans: LX-Lignin and L-(+)-Lactic Acid from Lignocellulose. Microorganisms 9, 1810. doi:10.3390/microorganisms9091810
Shahab, R. L., Luterbacher, J. S., Brethauer, S., and Studer, M. H. (2018). Consolidated Bioprocessing of Lignocellulosic Biomass to Lactic Acid by a Synthetic Fungal-Bacterial Consortium. Biotechnol. Bioeng. 115, 1207–1215. doi:10.1002/bit.26541
Shahbandeh, M. (2021a). Coffee Production Worldwide from 2003/04 to 2019/20 (In Million 60 Kilogram Bags). March 4. Available at: https://www.statista.com/statistics/263311/worldwide-production-of-coffee/(Accessed October 26, 2021).
Shahbandeh, M. (2021b). Corn Industry Worldwide - Statistics & Facts. July 23. Available at: https://www.statista.com/topics/7169/corn-industry-worldwide/#dossierKeyfigures.
Sharma, H. K., Xu, C., and Qin, W. (2019). Biological Pretreatment of Lignocellulosic Biomass for Biofuels and Bioproducts: An Overview. Waste Biomass Valor. 10, 235–251. doi:10.1007/s12649-017-0059-y
Shen, X., and Sun, R. (2021). Recent Advances in Lignocellulose Prior-Fractionation for Biomaterials, Biochemicals, and Bioenergy. Carbohydr. Polym. 261, 117884. doi:10.1016/j.carbpol.2021.117884
Shi, S., Guan, W., Blersch, D., and Li, J. (2021). Improving the Enzymatic Digestibility of Alkaline-Pretreated Lignocellulosic Biomass Using polyDADMAC. Ind. Crops Prod. 162, 113244. doi:10.1016/j.indcrop.2021.113244
Shi, Z., Wei, P., Zhu, X., Cai, J., Huang, L., and Xu, Z. (2012). Efficient Production of L-Lactic Acid from Hydrolysate of Jerusalem Artichoke with Immobilized Cells of Lactococcus Lactis in Fibrous Bed Bioreactors. Enzyme Microb. Technol. 51, 263–268. doi:10.1016/j.enzmictec.2012.07.007
Shuddhodana, D. M., Mohnot, D., Biswas, R., and Bisaria, V. S. (2016). “Enzymatic Hydrolysis of Lignocellulosic Residues,” in Biomass Fractionation Technologies for a Lignocellulosic Feedstock Based Biorefinery (Amsterdam: Elsevier), 543–560. doi:10.1016/b978-0-12-802323-5.00023-2
Si, H., Liang, X., Liu, S., Xu, M., Wang, J., and Hua, D. (2020). Efficient Production of L-Lactic Acid from Corn Straw Hydrolysate. IOP Conf. Ser. Earth Environ. Sci. 514, 052049. doi:10.1088/1755-1315/514/5/052049
Sidiras, D., Politi, D., Giakoumakis, G., and Salapa, I. (2022). Simulation and Optimization of Organosolv Based Lignocellulosic Biomass Refinery: A Review. Bioresour. Technol. 343, 126158. doi:10.1016/j.biortech.2021.126158
Sitompul, J. P., Simangunsong, R. F., Asrizal, A. A., Alisyahbana, H., Lee, H. W., and Rasrendra, C. B. (2014). Catalytic Conversion of Empty Fruit Bunch of Palm Oil for Producing Lactic Acid. Proced. Chem. 9, 88–93. doi:10.1016/j.proche.2014.05.011
Smits, J., Bevers, L., van Haastert, M., Wiertz, R., and Kroon, H. (2019). Fast Screening of Optimal Acid-Pretreatment Conditions in the Conversion of wood to Lignocellulosic Sugars. Bioresour. Technol. Rep. 5, 220–229. doi:10.1016/j.biteb.2019.01.016
Sun, Y., Li, X., Wei, C., Qi, W., and Xiu, Z. (2021). An Aptly Industrialized Bioprocess for Lactic Acid Production from Corn stover Using Thermotolerant Microbial Consortia. Bioproc. Biosyst Eng 44, 2445–2454. doi:10.1007/s00449-021-02616-5
Tan, J., Abdel-Rahman, M. A., and Sonomoto, K. (2018). Biorefinery-Based Lactic Acid Fermentation: Microbial Production of Pure Monomer Product. Adv. Polym. Sci. 279, 27–66.
Tarraran, L., and Mazzoli, R. (2018). Alternative Strategies for Lignocellulose Fermentation through Lactic Acid Bacteria: the State of the Art and Perspectives. FEMS Microbiol. Lett. 365 (15), fny126. doi:10.1093/femsle/fny126
Tejayadi, S., and Cheryan, M. (2005). Lactic Acid from Cheese Whey Permeate. Productivity and Economics. Appl. Microbiol. Biotechnol. 43, 242–248.
Thakur, A., Panesar, P. S., and Saini, M. S. (2019). l(+)-Lactic Acid Production by Immobilized Lactobacillus Casei Using Low Cost Agro-Industrial Waste as Carbon and Nitrogen Sources. Waste Biomass Valor. 10, 1119–1129. doi:10.1007/s12649-017-0129-1
Thygesen, A., Tsapekos, P., Alvarado-Morales, M., and Angelidaki, I. (2021). Valorization of Municipal Organic Waste into Purified Lactic Acid. Bioresour. Technol. 342, 125933. doi:10.1016/j.biortech.2021.125933
Tian, X., Liu, X., Zhang, Y., Chen, Y., Hang, H., Chu, J., et al. (2021). Metabolic Engineering Coupled with Adaptive Evolution Strategies for the Efficient Production of High-Quality L-Lactic Acid by Lactobacillus Paracasei. Bioresour. Technol. 323, 124549. doi:10.1016/j.biortech.2020.124549
Tišma, M., Bucić-Kojić, A., and Planinić, M. (2021). Bio-based Products from Lignocellulosic Waste Biomass: A State of the Art. Chem. Biochem. Eng. Q. 35 (2), 139–156.
Tramontina, R., Brenelli, L. B., Sodré, V., Franco Cairo, J. P., Travália, B. M., Egawa, V. Y., et al. (2020). Enzymatic Removal of Inhibitory Compounds from Lignocellulosic Hydrolysates for Biomass to Bioproducts Applications. World J. Microbiol. Biotechnol. 36, 166. doi:10.1007/s11274-020-02942-y
Tsegaye, B., Gupta, P., Balomajumder, C., and Roy, P. (2021). Optimization of Organosolv Pretreatment Conditions and Hydrolysis by Bacillus Sp. BMP01 for Effective Depolymerization of Wheat Straw Biomass. Biomass Conv. Bioref. 11 (6), 2747–2761. doi:10.1007/s13399-020-00691-4
Tu, W.-L., Hsu, T.-C., Wang, C.-A., Guo, G.-L., and Chao, Y. (2019). Using Novel Lactobacillus Plantarum to Produce Lactic Acid from Lignocellulosic Biomass in an Integrated Simultaneous Saccharification and Fermentation Process. BioResources 14 (2), 3873–3885.
Turner, T. L., Zhang, G.-C., Oh, E. J., Subramaniam, V., Adiputra, A., Subramaniam, V., et al. (2016). Lactic Acid Production from Cellobiose and Xylose by engineeredSaccharomyces Cerevisiae. Biotechnol. Bioeng. 113, 1075–1083. doi:10.1002/bit.25875
Unrean, P. (2018). Optimized Feeding Schemes of Simultaneous Saccharification and Fermentation Process for High Lactic Acid Titer from Sugarcane Bagasse. Ind. Crops Prod. 111, 660–666. doi:10.1016/j.indcrop.2017.11.043
Upadhyaya, B. P., DeVeaux, L. C., and Christopher, L. P. (2014). Metabolic Engineering as a Tool for Enhanced Lactic Acid Production. Trends Biotechnol. 32 (12), 637–644. doi:10.1016/j.tibtech.2014.10.005
Usmani, Z., Sharma, M., Awasthi, A. K., Lukk, T., Tuohy, M. G., Gong, L., et al. (2021). Lignocellulosic Biorefineries: The Current State of Challenges and Strategies for Efficient Commercialization. Renew. Sustain. Energ. Rev. 148, 111258. doi:10.1016/j.rser.2021.111258
Usmani, Z., Sharma, M., Gupta, P., Karpichev, Y., Gathergood, N., Bhat, R., et al. (2020). Ionic Liquid Based Pretreatment of Lignocellulosic Biomass for Enhanced Bioconversion. Bioresour. Technol. 304, 123003. doi:10.1016/j.biortech.2020.123003
Utrilla, J., Vargas-Tah, A., Trujillo-Martínez, B., Gosset, G., and Martinez, A. (2016). Production of D-Lactate from Sugarcane Bagasse and Corn stover Hydrolysates Using Metabolic Engineered Escherichia coli Strains. Bioresour. Technol. 220, 208–214. doi:10.1016/j.biortech.2016.08.067
van der Pol, E. C., Vaessen, E., Weusthuis, R. A., and Eggink, G. (2016). Identifying Inhibitory Effects of Lignocellulosic By-Products on Growth of Lactic Acid Producing Micro-organisms Using a Rapid Small-Scale Screening Method. Bioresour. Technol. 209, 297–304. doi:10.1016/j.biortech.2016.03.037
Vanmarcke, G., Demeke, M. M., Foulquié-Moreno, M. R., and Thevelein, J. M. (2021). Identification of the Major Fermentation Inhibitors of Recombinant 2G Yeasts in Diverse Lignocellulose Hydrolysates. Biotechnol. Biofuels 14, 92. doi:10.1186/s13068-021-01935-9
Velvizhi, G., Balakumar, K., Shetti, N. P., Ahmad, E., Kishore Pant, K., and Aminabhavi, T. M. (2022). Integrated Biorefinery Processes for Conversion of Lignocellulosic Biomass to Value Added Materials: Paving a Path towards Circular Economy. Bioresour. Technol. 343, 126151. doi:10.1016/j.biortech.2021.126151
Verma, D., and Subudhi, S. (2021). 'Lactobacillus Sp. Strain TERI-D3', as Microbial Cell Factory for Fermentative Production of Lactic Acid'. Curr. Res. Green Sustain. Chem. 4, 100059. doi:10.1016/j.crgsc.2021.100059
Vishnu Prasad, J., Sahoo, T. K., Naveen, S., and Jayaraman, G. (2020). Evolutionary Engineering of Lactobacillus Bulgaricus Reduces Enzyme Usage and Enhances Conversion of Lignocellulosics to D-Lactic Acid by Simultaneous Saccharification and Fermentation. Biotechnol. Biofuels 13, 171. doi:10.1186/s13068-020-01812-x
Wang, Y., Wang, M., Cai, D., Wang, B., Wang, Z., Qin, P., et al. (2016). Efficient L-Lactic Acid Production from Sweet Sorghum Bagasse by Open Simultaneous Saccharification and Fermentation. RSC Adv. 6, 35771–35777. doi:10.1039/c6ra04538c
Wang, Z.-K., Li, H., Lin, X.-C., Tang, L., Chen, J.-J., Mo, J.-W., et al. (2020). Novel Recyclable Deep Eutectic Solvent Boost Biomass Pretreatment for Enzymatic Hydrolysis. Bioresour. Technol. 307, 123237. doi:10.1016/j.biortech.2020.123237
Wei, C., Liu, G., Zhang, J., and Bao, J. (2018). Elevating Fermentation Yield of Cellulosic Lactic Acid in Calcium Lactate Form from Corn stover Feedstock. Ind. Crops Prod. 126, 415–420. doi:10.1016/j.indcrop.2018.10.041
Werlang, E. B., Ibarra, E. S., Neves, F. d. F., Julich, J., Martinez, A., and Schneider, R. d. C. d. S. (2020). D-lactate Production from Spirulina (Arthrospira Platensis) Biomass Using Lactogenic Escherichia coli. Bioresour. Technol. Rep. 12, 100598. doi:10.1016/j.biteb.2020.100598
Wischral, D., Arias, J. M., Modesto, L. F., de França Passos, D., and Pereira, N. (2019). Lactic Acid Production from Sugarcane Bagasse Hydrolysates by Lactobacillus Pentosus : Integrating Xylose and Glucose Fermentation. Biotechnol. Prog. 35 (1), e2718. doi:10.1002/btpr.2718
Wu, J., Chandra, R., Takada, M., Del Rio, P., Kim, K. H., Kim, C. S., et al. (2020). Alkaline Sulfonation and Thermomechanical Pulping Pretreatment of Softwood Chips and Pellets to Enhance Enzymatic Hydrolysis. Bioresour. Technol. 315, 123789. doi:10.1016/j.biortech.2020.123789
Wu, J., Xin, Y., Kong, J., and Guo, T. (2021). Genetic Tools for the Development of Recombinant Lactic Acid Bacteria. Microb. Cel Fact 20, 118. doi:10.1186/s12934-021-01607-1
Wu, M., Gong, L., Ma, C., and He, Y.-C. (2021). Enhanced Enzymatic Saccharification of Sorghum Straw by Effective Delignification via Combined Pretreatment with Alkali Extraction and Deep Eutectic Solvent Soaking. Bioresour. Technol. 340, 125695. doi:10.1016/j.biortech.2021.125695
Wu, J., Chandra, R., and Saddler, J. (2019). Alkali-oxygen Treatment Prior to the Mechanical Pulping of Hardwood Enhances Enzymatic Hydrolysis and Carbohydrate Recovery through Selective Lignin Modification. Sustain. Energ. Fuels 3 (1), 227–236. doi:10.1039/c8se00452h
Xu, H., Kong, Y., Peng, J., Song, X., Liu, Y., Su, Z., et al. (2021). Comprehensive Analysis of Important Parameters of Choline Chloride-Based Deep Eutectic Solvent Pretreatment of Lignocellulosic Biomass. Bioresour. Technol. 319, 124209. doi:10.1016/j.biortech.2020.124209
Xu, S., Lan, K., Li, J., He, T., and Hu, C. (2018). Separation of Lactic Acid from Synthetic Solutions and the Mixture Directly Derived from Corn stover by Aqueous Two Phase Extraction. Separat. Purif. Technol. 204, 281–289. doi:10.1016/j.seppur.2018.04.086
Yaashikaa, P. R., Senthil Kumar, P., and Varjani, S. (2022). Valorization of Agro-Industrial Wastes for Biorefinery Process and Circular Bioeconomy: a Critical Review. Bioresour. Technol. 343, 126126. doi:10.1016/j.biortech.2021.126126
Yadav, N., Nain, L., and Khare, S. K. (2021). One-pot Production of Lactic Acid from rice Straw Pretreated with Ionic Liquid. Bioresour. Technol. 323, 124563. doi:10.1016/j.biortech.2020.124563
Yadav, N., Pranaw, K., and Khare, S. K. (2020). Screening of Lactic Acid Bacteria Stable in Ionic Liquids and Lignocellulosic By-Products for Bio-Based Lactic Acid Production. Bioresour. Technol. Rep. 11, 100423. doi:10.1016/j.biteb.2020.100423
Yang, X., Lai, Z., Lai, C., Zhu, M., Li, S., Wang, J., et al. (2013). Efficient Production of L-Lactic Acid by an Engineered Thermoanaerobacterium Aotearoensewith Broad Substrate Specificity. Biotechnol. Biofuels 6, 124. doi:10.1186/1754-6834-6-124
Yankov, D. (2020). Aqueous Two-phase Systems as a Tool for Bioseparation - Emphasis on Organic Acids. Phys. Sci. Rev. 5 (9), 20180067. doi:10.1515/psr-2018-0067
Yao, Ri., Qi, B., Deng, Sh., Liu, N., Peng, Sh., and Cui, Q. (2007). Use of Surfactants in Enzymatic Hydrolysis of rice. BioResources 2 (3), 389–398.
Yoshida, S., Okano, K., Tanaka, T., Ogino, C., and Kondo, A. (2011). Homo-D-lactic Acid Production from Mixed Sugars Using Xylose-Assimilating Operon-Integrated Lactobacillus Plantarum. Appl. Microbiol. Biotechnol. 92, 67–76. doi:10.1007/s00253-011-3356-6
Younas, R., Zhang, S., Zhang, L., Luo, G., Chen, K., Cao, L., et al. (2016). Lactic Acid Production from rice Straw in Alkaline Hydrothermal Conditions in Presence of NiO Nanoplates. Catal. Today 274, 40–48. doi:10.1016/j.cattod.2016.03.052
Zaini, N. A. B. M., Chatzifragkou, A., and Charalampopoulos, D. (2019). Alkaline Fractionation and Enzymatic Saccharification of Wheat Dried Distillers Grains with Solubles (DDGS). Food Bioproducts Process. 118, 103–113. doi:10.1016/j.fbp.2019.09.006
Zaini, N. A. B. M., Chatzifragkou, A., and Charalampopoulos, D. (2019). Microbial Production Ofd-Lactic Acid from Dried Distiller's Grains with Solubles. Eng. Life Sci. 19, 21–30. doi:10.1002/elsc.201800077
Zhang, F., Lan, W., Li, Z., Zhang, A., Tang, B., Wang, H., et al. (2021). Co-production of Functional Xylo-Oligosaccharides and Fermentable Sugars from Corn stover through Fast and Facile ball Mill-Assisted Alkaline Peroxide Pretreatment. Bioresour. Technol. 337, 125327. doi:10.1016/j.biortech.2021.125327
Zhang, L., Li, X., Yong, Q., Yang, S.-T., Ouyang, J., and Yu, S. (2016). Impacts of Lignocellulose-Derived Inhibitors on L-Lactic Acid Fermentation by Rhizopus Oryzae. Bioresour. Technol. 203, 173–180. doi:10.1016/j.biortech.2015.12.014
Zhang, Y., Kumar, A., Hardwidge, P. R., Tanaka, T., Kondo, A., and Vadlani, P. V. (2016). D-Lactic Acid Production from Renewable Lignocellulosic Biomass via Genetically modifiedLactobacillus Plantarum. Biotechnol. Prog. 32 (2), 271–278. doi:10.1002/btpr.2212
Zhang, Y., Vadlani, P. V., Kumar, A., Hardwidge, P. R., Govind, R., Tanaka, T., et al. (2016). Enhanced D-Lactic Acid Production from Renewable Resources Using Engineered Lactobacillus Plantarum. Appl. Microbiol. Biotechnol. 100, 279–288. doi:10.1007/s00253-015-7016-0
Zhang, Z., Li, Y., Zhang, J., Peng, N., Liang, Y., and Zhao, S. (2020). High-Titer Lactic Acid Production by Pediococcus Acidilactici PA204 from Corn Stover through Fed-Batch Simultaneous Saccharification and Fermentation. Microorganisms 8, 1491. doi:10.3390/microorganisms8101491
Zhao, L., Sun, Z.-F., Zhang, C.-C., Nan, J., Ren, N.-Q., Lee, D.-J., et al. (2022). Advances in Pretreatment of Lignocellulosic Biomass for Bioenergy Production: Challenges and Perspectives. Bioresour. Technol. 343, 126123. doi:10.1016/j.biortech.2021.126123
Zheng, J., Wittouck, S., Salvetti, E., Franz, C. M. A. P., Harris, H. M. B., Mattarelli, P., et al. (2020). A Taxonomic Note on the Genus Lactobacillus: Description of 23 Novel Genera, Emended Description of the Genus Lactobacillus Beijerinck 1901, and union of Lactobacillaceae and Leuconostocaceae. Int. J. Syst. Evol. Microbiol. 70, 2782–2858. doi:10.1099/ijsem.0.004107
Zheng, X., Xian, X., Hu, L., Tao, S., Zhang, X., Liu, Y., et al. (2021). Efficient Short-Time Hydrothermal Depolymerization of Sugarcane Bagasse in One-Pot for Cellulosic Ethanol Production without Solid-Liquid Separation, Water Washing, and Detoxification. Bioresour. Technol. 339, 125575. doi:10.1016/j.biortech.2021.125575
Zhou, J., Ouyang, J., Xu, Q., and Zheng, Z. (2016). Cost-effective Simultaneous Saccharification and Fermentation of L -lactic Acid from Bagasse Sulfite Pulp by Bacillus Coagulans CC17. Bioresour. Technol. 222, 431–438. doi:10.1016/j.biortech.2016.09.119
Zhou, X., Sun, Y., Zhan, H., Liu, H., Wang, X., Xu, Y., et al. (2021). Ionic Liquid-Based Multi-Stage Sugaring-Out Extraction of Lactic Acid from Simulated Broth and Actual Lignocellulosic Fermentation Broth. Bioresour. Bioproc. 8, 123. doi:10.1186/s40643-021-00481-4
Zulkefli, S., Abdulmalek, E., and Abdul Rahman, M. B. (2017). Pretreatment of Oil palm Trunk in Deep Eutectic Solvent and Optimization of Enzymatic Hydrolysis of Pretreated Oil palm Trunk. Renew. Energ. 107, 36–41. doi:10.1016/j.renene.2017.01.037
Keywords: lignocellulosic biomass, lactic acid, pretreatment, fermentation, separation
Citation: Yankov D (2022) Fermentative Lactic Acid Production From Lignocellulosic Feedstocks: From Source to Purified Product. Front. Chem. 10:823005. doi: 10.3389/fchem.2022.823005
Received: 26 November 2021; Accepted: 21 January 2022;
Published: 04 March 2022.
Edited by:
Svilen Simeonov, Institute of Organic Chemistry with Centre of Phytochemistry (BAS), BulgariaReviewed by:
Paripok Phitsuwan, King Mongkut’s University of Technology Thonburi, ThailandCopyright © 2022 Yankov. This is an open-access article distributed under the terms of the Creative Commons Attribution License (CC BY). The use, distribution or reproduction in other forums is permitted, provided the original author(s) and the copyright owner(s) are credited and that the original publication in this journal is cited, in accordance with accepted academic practice. No use, distribution or reproduction is permitted which does not comply with these terms.
*Correspondence: Dragomir Yankov, eWFucGVAYmFzLmJn
Disclaimer: All claims expressed in this article are solely those of the authors and do not necessarily represent those of their affiliated organizations, or those of the publisher, the editors and the reviewers. Any product that may be evaluated in this article or claim that may be made by its manufacturer is not guaranteed or endorsed by the publisher.
Research integrity at Frontiers
Learn more about the work of our research integrity team to safeguard the quality of each article we publish.