- 1School of Basic Medical Sciences, Nanchang University, Nanchang, China
- 2Queen Mary School, Nanchang University, Nanchang, China
Severe acute respiratory syndrome Coronavirus-2 (SARS-CoV-2), the pathogen of the Coronavirus disease-19 (COVID-19), is still devastating the world causing significant chaos to the international community and posing a significant threat to global health. Since the first outbreak in late 2019, several lines of intervention have been developed to prevent the spread of this virus. Nowadays, some vaccines have been approved and extensively administered. However, the fact that SARS-CoV-2 rapidly mutates makes the efficacy and safety of this approach constantly under debate. Therefore, antivirals are still needed to combat the infection of SARS-CoV-2. Papain-like protease (PLpro) of SARS-CoV-2 supports viral reproduction and suppresses the innate immune response of the host, which makes PLpro an attractive pharmaceutical target. Inhibition of PLpro could not only prevent viral replication but also restore the antiviral immunity of the host, resulting in the speedy recovery of the patient. In this review, we describe structural and functional features on PLpro of SARS-CoV-2 and the latest development in searching for PLpro inhibitors. Currently available inhibitors targeting PLpro as well as their structural basis are also summarized.
Introduction
Severe acute respiratory syndrome coronavirus 2 (SARS-CoV-2), causing the coronavirus disease 2019 (COVID-19) (Wu F. et al., 2020; Zhu et al., 2020), is the third highly pathogenic human coronavirus in this century after the SARS-CoV emerged in 2003 (Stadler et al., 2003) and the Middle East Respiratory Syndrome Coronavirus (MERS-CoV) emerged in 2012 (Chafekar and Fielding, 2018). Although it has a relatively lower mortality rate, SARS-CoV-2 exhibits a higher transmission efficiency compared to SARS-CoV and MERS-CoV (Madewell et al., 2020; Patel et al., 2020). The rapid spread of SARS-CoV-2 has continued to cause a worldwide pandemic and posed a serious threat to global public health since the beginning of 2020. The SARS-CoV-2 infection mostly affects the lungs and causes symptoms of varying degrees of morbidity, ranging from asymptomatic infection to mild infection with flu-like illness or severe infection with lung injury (Chen et al., 2020; Huang et al., 2020; Wang et al., 2020). COVID-19 outbreak has resulted in over 320 million confirmed cases as of 16 January 2022, including nearly 5.5 million deaths (https://www.who.int/publications/m/item/weekly-epidemiological-update-on-covid-19---18-january-2022). Though vaccines have been administered on a large scale, this viral disease is far from being controlled, in particular, due to the occurrence of cumulative mutations. Given the ongoing pandemic and disruptive impact, there is still an urgent need to develop new antiviral strategies for the prompt and effective therapy of SARS-CoV-2 infection.
SARS-CoV-2 is an enveloped, single-stranded, and positive-sense RNA virus (+ssRNA) which belongs to the Coronaviridae family (Khailany et al., 2020; Zhou et al., 2020). Coronaviruses can be divided into four groups indicated with the Greek letters α, β, γ, and δ, respectively (Su et al., 2016). Along with the newly emerged SARS-CoV-2, seven coronaviruses are currently able to infect humans, among which HCoV-NL63 and HCoV-229E belong to α coronavirus, while the others (HCoV-HKU1, HCoV-OC43, SARS-CoV, MERS-CoV, and SARS-CoV-2) belong to β coronavirus (Ye et al., 2020). Similar to other human coronaviruses, the genome of SARS-CoV-2 has two open reading frames that encode two replicase polyproteins, namely pp1a and pp1ab. The polyproteins are digested into sixteen mature non-structural proteins (nsp1-16) by two cysteine proteases, chymotrypsin-like protease (3CLpro or Mpro) encoded by nsp5 and papain-like protease (PLpro) encoded by nsp3. Mpro cleavage results in the releasing of the functional nsp4-16, while PLpro cleavage results in the maturation of nsp1-3 (Fehr and Perlman, 2015).
Importantly, SARS-CoV-2 PLpro possesses additional function of inhibiting interferon related antiviral responses of the host (Klemm et al., 2020; Shin et al., 2020). Given that SARS-CoV-2 causes a substantially higher mortality rate in elderly patients with compromised immune systems (Ruan 2020; Wu and McGoogan 2020), viral factors that mitigate or evade from immune responses are desirable drug targets. Thus, therapy by targeting SARS-CoV-2 PLpro can not only suppress viral infection but also promote antiviral immunity, which is similar to killing two birds with one stone. Herein, this review updates the recent research progress in the structure and function of SARS-CoV-2 PLpro and the discovery of PLpro inhibitors against COVID-19.
Structural and Functional Features of SARS-CoV-2 PLpro
The genome of SARS-CoV-2 is approximately 30 kb in size and contains at least 12 open reading frames (ORF) that flanked by 5′-cap and 3′-poly(A) tail (Wu A. et al., 2020; Zhang RH. et al., 2020). The 3′-terminal one-thirds of the SARS-CoV-2 genome encodes four structural proteins (spike protein, envelope protein, membrane protein, and nucleocapsid protein) and several accessory proteins, while two-thirds of the SARS-CoV-2 genome at the 5′-terminal encodes two replicase polyproteins, namely pp1a (about 450 KD) and pp1ab (about 750 KD) (Figure 1). These two polyproteins can be cleaved by virus-encoded PLpro and 3C-like protease into 16 non-structural proteins. Many of them are localized to the double membrane like vesicles and assemble into a replication complex on the cytoplasmic face of the endoplasmic reticulum (Knoops et al., 2008; Klein et al., 2020). PLpro is part of the largest non-structural protein nsp3 and is highly conserved (Lei et al., 2018). Often two copies, referred to as PL1pro and PL2pro, are found in coronaviruses and the two PLpros show distinct substrate specificity in different coronaviruses (Woo et al., 2010; Mielech et al., 2014). For example, PL1pro of mouse hepatitis virus processes between nsp1/2 and between nsp2/3, while PL2pro processes between nsp3/4 (Bonilla et al., 1997; Kanjanahaluethai and Baker, 2000). In the case of HCoV-NL63, PL1pro cleaves between nsp1/2, while PL2pro cleaves between nsp2/3 and between nsp3/4 (Chen et al., 2007). However, like SARS-CoV and MERS-CoV, only one functional PLpro is encoded in SARS-CoV-2. After being released from nsp3 through autocleavage, SARS-CoV-2 PLpro recognizes the common motif LXGG(A/K)X between nsp1/2, nsp2/3, and nsp3/4 (X represents any type of amino acid) and cleaves between glycine and alanine/lysine residues (Anirudhan et al., 2021), which is essential for coronavirus RNA synthesis and viral survival (Shamsi et al., 2021; Yadav et al., 2021). Enzyme activity analysis revealed that the S2 site of PLpro strictly recognizes glycine and the S4 site preferentially recognizes amino acids with hydrophobic side chains, while the S3 site has broader substrate specificity and can recognize any type of amino acid (Rut et al., 2020).
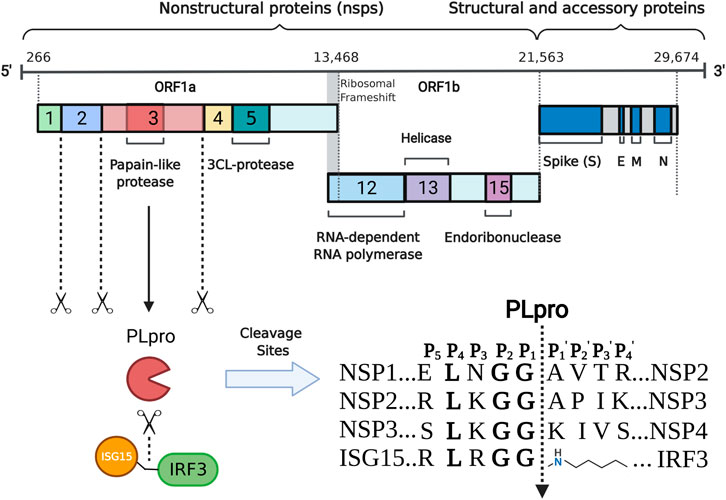
FIGURE 1. Schematic representation of the production and function of SARS- CoV- 2 PLpro. The positive- strand RNA (numbers indicate nucleotide position) including the ORF 1a/b, structural proteins S (spike), E (envelope), M (membrane) and N (nucleocapsid) are shown. PLpro is encoded by part of nsp3. It is able to mature nsp1- 3 and preferentially cleave the ubiquitin- like protein ISG15 from IRF3 by recognizing specific cleavage sites.
Beyond the role in cleaving the viral polypeptide, PLpro also participates in regulating host antiviral innate immunity through antagonising ubiquitin and ubiquitin-like modifications (Shin et al., 2020; Klemm et al., 2020). The host elicits various defense strategies to thwart viral infection. During viral invasion, the host could recognize the specific viral components through pattern recognition receptors and subsequently produce type I interferon and proinflammatory cytokines to establish the first line of host defense against viral infection. Ubiquitins and ubiquitin- like protein ISG15, an interferon- induced protein, are important post- translation modifying processes and have emerged as crucial players at this stage (Zheng and Gao, 2019, Zheng and Gao, 2020; Perng and Lenschow, 2018; McClain and Vabret, 2020). In response, virus often repurposes its protease to evade host antiviral immunity through deubiquitinating and deISGylating activities (James et al., 2011; Swatek et al., 2018). In the case of SARS-CoV-2, PLpro can remove ubiquitin and ISG15 modifications from host proteins by cleaving the consensus site (LXGG) (Figure 1) (Swaim et al., 2020). Similar to SARS-CoV PLpro, SARS-CoV-2 PLpro shows detectable activity for K48-linked ubiquitin chains but not K63, even though at a substantially slower rate (Freitas et al., 2020; Rut et al., 2020; Shin et al., 2020). Unlike SARS-CoV PLpro that predominantly cleaves K48 ubiquitin chains, PLpro of SARS-CoV-2 shows an enhanced deISGylation activity (Freitas et al., 2020; Rut et al., 2020; Shin et al., 2020). However, the deISGylation activity is sensitive to species-species differences (Freitas et al., 2020). SARS-CoV-2 PLpro appears to prefer ISG15s from sheep and the vesper bat, but shows no protease activity for the ISG15 substrate from fish. The deISGylase activities are moderate for ISG15s from human, pig, camel, and mouse, while weak for ISG15s from Egyptian fruit bat, hedgehog, and northern tree shrew. The preference of PLpro in processing ISG15 substrates may indicate the species that SARS-CoV-2 can productively infect.
Recently, the crystal structure of the 315-residue SARS-CoV-2 PLpro has been solved (Osipiuk et al., 2021). Similar to PLpros of SARS-CoV and MERS-CoV PLpro, SARS-CoV-2 PLpro contains a ubiquitin-like domain at N-terminal and a catalytic domain at C-terminal, which can be divided into three subdomains, namely thumb, palm and finger subdomains (Figure 2A). The catalytic site of SARS-CoV-2 PLpro contains a classical catalytic triad, consisting of Cys111, His273, and Asp287, and is situated at the interface between thumb and palm subdomains. A 6-amino-acid (267–272 residues) flexible loop named blocking loop 2 (BL2) near the catalytic site plays an important role in controlling the access to the active site (Ratia et al., 2008). The finger subdomain contains a zinc-ribbon region and zinc ion binding is necessary for enzyme catalysis and structural integrity of PLpro (Tian et al., 2021; Zhao et al., 2021).
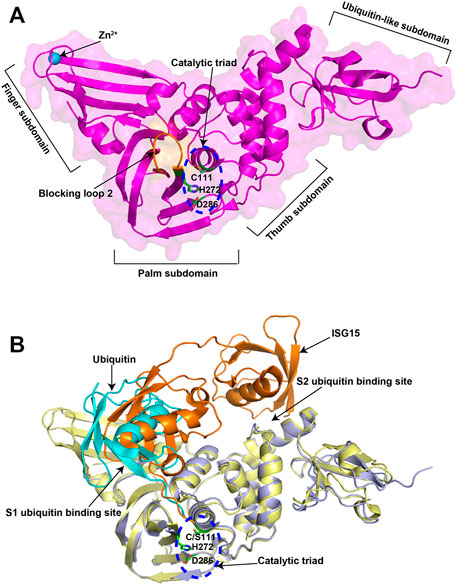
FIGURE 2. Crystal structures of SARS-CoV-2 PLpro in apo and substrate-bound forms. (A) Crystal structure of SARS-CoV-2 PLpro (PDB ID: 6WZU). The four distinct subdomains are indicated. The catalytic triad is shown in the blue dashed circle. (B) Superposition of ubiquitin/PLpro complex (PDB ID: 6XAA) with mouse-ISG15/PLpro complex (PDB ID: 6YVA). The catalytic triad is shown in the blue dashed circle. The two ubiquitin binding sites are indicated. The ubiquitin is colored in cyan, while the mouse ISG15 is colored in orange.
PLpro possesses two ubiquitin binding sites (S1 and S2) for the recognition of its substrates, ubiquitin and ISG15, with the structural basis of which has also been elucidated (Klemm et al., 2020; Shin et al., 2020). S1 ubiquitin binding site recognizes the C-terminal domain of ISG15 through a different binding orientation compared with ubiquitin (Figure 2B). S2 ubiquitin binding site not only recognizes the N-terminal domain of ISG15, but also provides exquisite specificity for the distal ubiquitin of K48-linked diubiquitin chains. Residue Phe69 and Thr75 in the S2 ubiquitin-binding site are key amino acids for PLpro to interact with Ile44 in ISG15, which may contribute to the enhanced affinity for ISG15. However, these observations are based on the available structures of SARS-CoV-2 PLpro in complex with ubiquitin or mouse full-length ISG15. It would be of interest in future to determine the structure of human full-length ISG15 or K48-diubiquitin in complex with SARS-CoV-2 PLpro.
Nevertheless, these data reveal the 3D structures of PLpro at both apo state and substrate-binding state, which facilitates the PLpro based drug design. Molecules that mimic the hydrophobic interaction with SARS-CoV-2 PLpro observed in its substrates or alter the conformation of BL2 in the active site will affect the protease activity.
Strategies in PLpro Inhibitor Development
Drug Repurposing
Drug repurposing refers to the discovery of new therapeutic uses of clinically available drugs. It is a prompt strategy in searching of medications for COVID-19 therapy since the traditional drug discovery process can be costly and takes decades to complete (Aherfi et al., 2021; Hijikata et al., 2021; Mslati et al., 2021; Shende et al., 2021). As repurposed drugs have gone through clinical trial, important parameters of these drugs, such as inhibitory potential, cell permeability, bio-availability, and safety, have been well characterized and can be available in a short time in face of pandemics (Pushpakom et al., 2019). Since the outbreak of COVID-19 in late December, 2019, drug repurposing has been employed to deal with this worldwide health emergency by targeting PLpro, even though such efforts were not as successful as hoped at the beginning of the pandemic.
The disclosure of high resolution crystal structures of SARS-CoV-2 PLpro has made virtual screening a useful strategy in drug repurposing (Gao et al., 2021; Ma et al., 2021; Osipiuk et al., 2021). In several studies, the crystal structure of SARS-CoV-2 PLpro was included in a molecular docking analysis to identify PLpro inhibitors (Choudhury et al., 2021; Hosseini et al., 2021). In addition, pharmacophore based screen is an alternative method to obtain potential inhibitors (Kouznetsova et al., 2020). Although virtual screening is a useful strategy for drug repurposing, further in vitro and in vivo tests are necessary to confirm its activities against SARS-CoV-2 PLpro.
High-throughput screening (HTS) is another useful strategy in drug repurposing. It selects the most promising candidates from a large number of molecules via experimental approaches. Smith et al. developed a cell-based luciferase complementation assay to evaluate the inhibition of known drugs against SARS-CoV-2 viral PLpro (Smith et al., 2020). Based on the fluorescence-based enzymatic inhibition assay, several groups have identified potential inhibitors of SARS-CoV-2 PLpro with the IC50 value under 10 μM (Cho et al., 2021; Xu et al., 2021; Zhao et al., 2021). Although not all the identified compounds exhibited good performance in a cell-based assay, they could serve as a starting point for further chemical modification.
Discovery of New Drug Leads
Discovery of new drug leads is an important step to develop a novel drug. It involves the employment of a wide range of technologies including virtual screening, high-throughput screening and structure-based drug design (Guido et al., 2011). These interdisciplinary expertises are complementary and comprehensive application of a variety of techniques in identification of new lead compounds is feasible. The general process of leads identification includes two essential steps, namely hits generation and subsequent hits validation. Compared with drug repurposing, screenings for hit compounds, either in vitro or in silico, incorporate a much larger scale of molecules including microbial metabolites, natural products and marine-derived bio-active compounds (Cragg and Newman, 2013; Kumar et al., 2021; Quimque et al., 2021). Since the outbreak of COVID-19, the widespread use of combinatorial screening has revealed a large mount of hit compounds that specifically target SARS-CoV-2 PLpro (Amin et al., 2021; Gogoi et al., 2021; Goyzueta-Mamani et al., 2021; Hajbabaie et al., 2021; Jade et al., 2021; Jamalan et al., 2021; Kumar et al., 2021; Li et al., 2021; Quimque et al., 2021; Rahul and Sarkar, 2021; Rudrapal et al., 2021; Stasiulewicz et al., 2021). Hits generated through the initial screens are further validated with an aim to choose the best ones to serve as leads for drug developments. As not all the identified lead compounds exhibited desired activities, co-crystallization of these effective inhibitors with PLpro is often adopted to investigate how the two molecules interact (Wang et al., 2021), which can inform further lead optimization and future drug design.
Optimization of Lead Compounds
Once a potential lead is identified, lead optimization is pressing as the potency, selectivity, or pharmacokinetic parameters of leads identified may not be satisfactory. Thus, optimization of lead compounds is a crucial step in the development of new drugs. As an example of success, Shan et al. optimized GRL0617 based on the GRL0617/PLpro complex structure and found analogue 19 has favorable potency and selectivity against SARS-CoV-2 PLpro (Shan et al., 2021). Also, Ma et al. optimized the Jun9-13-7 and Jun9-13-9 (Ma et al., 2021), while Weglarz-Tomczak et al. modified ebselen (Weglarz-Tomczak et al., 2021). Both optimized inhibitors are more potent than parent compounds. It is worth noting that all these optimized inhibitors suppress the PLpro activity at nanomolar level, representing the most potent inhibitors at the time of this manuscript submission.
Potential SARS-CoV-2 PLpro Inhibitors
Many inhibitors have been identified against SARS-CoV-2 PLpro by using the strategies as stated above, even though no PLpro inhibitor has been approved by the US Food and Drug Administration (FDA) for marketing. Cysteine protease inhibitors can be divided into two categories, namely, covalent and non-covalent inhibitors. Covalent inhibitors form a C-S thioether linkage with the catalytic residue cysteine, while the non-covalent inhibitors interact with the protease through non-covalent binding which is always a reversible process (Aljoundi et al., 2020). SARS-CoV-2 PLpro inhibitors identified to date are mostly non-covalent, including naphthalene-based inhibitors, FDA approved drugs and natural products. As follows, we review the promising inhibitors currently known that target SARS-CoV-2 PLpro with the representative ones summarized in Table 1.
Naphthalene-Based Inhibitors
GRL0617, a naphthalene-based drug, was previously developed as a non-covalent inhibitor of SARS-CoV PLpro (Ratia et al., 2008). In various high-throughput screen studies for SARS-CoV-2 PLpro inhibitors, it has stood out for its potent protease inhibition and antiviral activities, with the IC50 being around 2.0 μM and EC50 being around 20 μM, respectively (Table 1) (Gao et al., 2021; Fu et al., 2021; Osipiuk et al., 2021; Klemm et al., 2020; Freitas et al., 2020). Several groups then resolved the crystal structure of SARS-CoV-2 PLpro in complex with GRL0617 and revealed its inhibitory mechanism (Gao et al., 2021; Fu et al., 2021; Osipiuk et al., 2021; Ma et al., 2021). According to the structural information, GRL0617 fits well with the substrate cleft with its aromatic ring and naphthalene group inserted into S3 and S4 pockets, respectively (Figures 3A,B). Unlike other inhibitors such as VIR251 (described below), which stabilizes the BL2 in an open conformation, GRL0617 keeps this loop in a close conformation (Figure 3A) (Rut et al., 2020; Gao et al., 2021). Thus, GRL0617 prevents the substrate from entering the active site and is therefore a competitive inhibitor. These findings inspire the discovery of new generation of naphthalene based PLpro inhibitors.
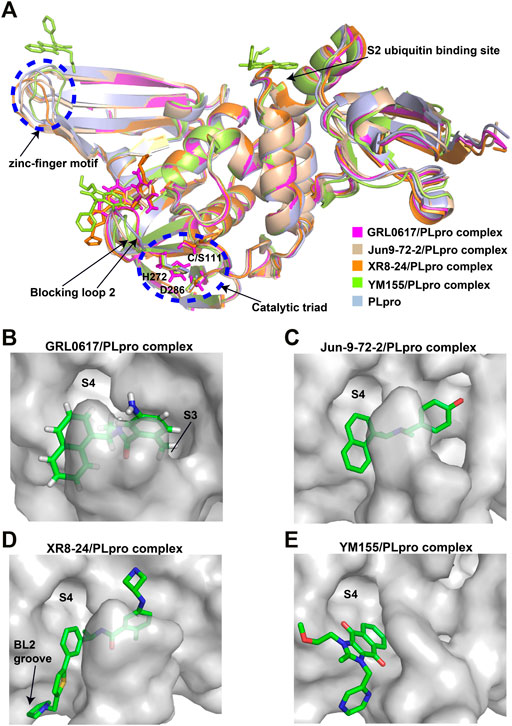
FIGURE 3. Crystal structure of SARS-CoV-2 PLpro in complex with non-covalent inhibitors. (A) Superposition of PLpro (light blue; PDB ID: 6WZU) with GRL0617/PLpro (magentas; PDB ID: 7CMD), Jun9-72-2/PLpro (wheat; PDB ID: 7SDR), XR8-24/PLpro (orange; PDB ID: 7LBS), and YM155/PLpro (limon; PDB ID: 7D7L) complexes. The catalytic triad and the zinc-finger motif are shown in the blue dashed circle. The blocking loop 2 and the S2 ubiquitin binding site are indicated. (B) Surface presentation of GRL0617 binding pocket. PLpro is shown as gray surface, while GR0617 is shown as sticks. S3 and S4 pockets are labeled. (C) Surface presentation of Jun9-72-2 binding pocket. PLpro is shown as gray surface, while Jun9-72-2 is shown as sticks. S4 pocket is labeled. (D) Surface presentation of XR8-24 binding pocket. PLpro is shown as gray surface, while XR8-24 is shown as sticks. S4 pocket and BL2 groove are labeled. (E) Surface presentation of YM155 binding pocket. PLpro is shown as gray surface, while YM155 is shown as sticks. S4 pocket is labeled.
Osipiuk et al. reported several GRL0617 analogues that also show good inhibition of SARS-CoV-2 PLpro (Osipiuk et al., 2021). However, none of them show more potent inhibition of SARS-CoV-2 PLpro compared with GRL0617. Later, Ma et al. reported two lead compounds, Jun9-13-7 and Jun9-13-9, that exhibits similar potency compared with GRL0617 (Ma et al., 2021). Encouragingly, two of the optimized forms, Jun9-72-2 and Jun9-75-4, show more potent enzymatic inhibition and antiviral activity compared to GRL0617. The IC50 and EC50 values of Jun9-72-2 and Jun9-54-7 are several-fold lower than those of GRL0617 (Table 1), thus making them very effective PLpro inhibitors. Jun9-72-2 adopts a similar binding model with GRL0617 (Figures 3A,C). Shan et al. focused on the structure-based optimization of GRL0617 for searching for improved GRL0617 analogues (Shan et al., 2021), as GRL0617 lacks sufficient potency for development as an antiviral agent. Finally, 9 GRL0617 analogues carrying a shared naphthyl subunit were found to be more potent than GRL0617. Among these, analogue 19 exhibits an inhibitory activity against SARS-CoV-2 PLpro with the IC50 value of 0.44 μM and an antiviral activity against SARS-CoV-2 with the IC50 value of 0.18 μM (Table 1). Moreover, analogue 19 shows no significant cross-inhibition against another 10 deubiquitinating enzymes (DUB) or DUB-like proteases even at the concentration of 10 μM (Shan et al., 2021). Collectively, analogue 19 is a promising SARS-CoV-2 PLpro inhibitor in virtue of its high degree of potency and selectivity.
In addition to GRL0617, another three previously identified naphthalene-based inhibitors, namely, rac3j, rac3k, and rac5c (the racemic version of 3j, 3k, and 5c, respectively), also show a promising inhibitory activity against SARS-CoV-2 PLpro. Rac5c is the best one with an in vitro IC50 value of 0.81 μM (Table 1) (Klemm et al., 2020). In the antiviral assay, rac5c could protect SARS-CoV-2 infected Vero cells from cytopathic effect without causing cell toxicity at a concentration of 11 μM, indicating the striking antiviral effects.
XR8-23/XR8-24
Shen et al. attached more attention in optimizing the potency of GRL0617 by exploring the engagement of additional binding sites beyond those utilized by GRL0617 (Shen et al., 2021). ZN-2–184, a derivative of GRL0617 with an azetidine substitution on the phenyl ring, yielded a 2-fold improved affinity through extending interaction with Glu167 of PLpro. ZN-3-80, a derivative of ZN-2-184 with the naphthyl group replaced by a biaryl group, showed improved metabolic stability compared with GRL0617. Further attempt explored the derivatization of the 2-phenylthiophene scaffold (ZN-3-80) to exploit additional interactions with the BL2 groove, a promising site that has not yet been recognized by other PLpro inhibitors. Among the newly synthesized 2-phenylthiophene inhibitors, two well-designed robust ones, XR8-23 and XR8-24, display low nanomolar potency against SARS-CoV-2 PLpro (IC50 values of 0.39 and 0.56 μM, respectively) and low micromolar potency against viral infection in human lung epithelial A549 cells (EC50 values of 1.4 and 1.2 μM, respectively) (Table 1) (Shen et al., 2021), which improved greatly over GRL0617. Furthermore, XR8-23 and X8-24 shows satisfactory bioavailability after intraperitoneal injection in a mouse model, even though their in vivo antiviral activities remain to be investigated.
XR8-23 has a basic amine side chain extending from the thiophene group, which leads to dissociation rates slower and potency stronger than those of ZN-3-80 and GRL0617. XR8-24 contains a pyrrolidine ring extending from the thiophene group, which results in the formation of a putative hydrogen bond with Tyr264 and accordingly accounts for its superior potency. XR8-24 do not access the active site, but engage the BL2 groove to enforce the sealing of the active site, as proved by the crystal structure of XR8-24 in complex with SARS-CoV-2 PLpro (Figures 3A,D) (Shen et al., 2021).
YM155
A high-throughput screen also identified a new lead YM155, a phase I clinical trial antineoplastic drug. It inhibits SARS-CoV-2 PLpro with an IC50 value of 2.47 μM and shows robust antiviral activity with an EC50 value of 0.17 μM (Table 1) (Zhao et al., 2021). The crystal structure of SARS-CoV-2 PLpro (with a C111S mutation) in complex with YM155 unravels a unique interaction mechanism, which may explain the strong inhibition achieved by YM155 against PLpro. In addition to targeting the substrate-binding pocket like GRL0617, YM155 also targets the thumb domain and the zinc finger motif (Figures 3A,E). Thus, YM155 not only blocks the entrance of substrate into the active site, but also hampers the molecular interactions between PLpro and ISG15 and affects the stability of the zinc-finger motif and enzyme activity.
Natural Products
A wide range of natural products provide an ideal library for the screen and identification of new drug candidates targeting SARS-CoV-2 PLpro. Flavonoids are a kind of widely distributed plant secondary metabolites with more than 9,000 structures currently identified (Wang et al., 2018). As the largest group of polyphenolic compounds in higher plants, flavonoids have shown PLpro inhibitory effects and antiviral activities against SARS-CoV and MERS-CoV in vitro (Jo et al., 2020; Solnier and Fladerer, 2020). In the setting of SARS-CoV-2, several members of flavonoids, including coumaroyltyramine, cryptotanshinone, kaempferol, moupinamide, N-cis-feruloyltyramine, quercetin, and tanshinone IIa, were identified as SARS-CoV-2 PLpro ligands using an in silico docking analysis (Zhang D.-h. et al., 2020), despite the lack of in vitro evidence for their efficacy. These compounds might interfere with substrate entering the active sites through interacting with the region between the thumb and palm domains (Zhang D.-h. et al., 2020). Cryptotanshinone, one of the active ingredient from the Chinese herbal medicine, Salvia miltiorrhiza, was emphasized for its potential in inhibiting SARS-CoV-2 PLpro in another study (Zhao et al., 2021). In vitro, cryptotanshinone inhibits PLpro with an IC50 value of 5.63 μM and display antiviral activity with an EC50 value of 0.70 μM (Table 1), which is comparable to that of remdesivir (0.77 μM). Structurally similar with cryptotanshinone, tanshinone I possesses similar inhibition against SARS-CoV-2 PLpro (Diniz et al., 2021; Zhao et al., 2021). In addition, dihydrotanshinone I, another tanshinone derivative, could effectively inhibit the SARS-CoV-2 proliferation at an EC50 of 8 µM and is not cytotoxic even at high concentrations (Lim et al., 2021). Thus, tanshinones and its analogues have the potential to treat SARS-CoV-2 infection. Actually, “Xuebijing”, a complex traditional Chinese preparation consisting of Salvia miltiorrhiza, has been included in the Chinese clinical treatment strategy for COVID-19 and reduces multiple organ damage through anti-inflammation and improving immune function (Tong et al., 2020). Other natural compounds, such as EGCG (a Green Tea Catechin) (Chourasia et al., 2021), cyanovirin-N (Naidoo et al., 2021), several Terpene compounds (Diniz et al., 2021), and propolis derivatives (Yosri et al., 2021), were also extensively studied in molecular simulations and exhibited high binding affinities with SARS-CoV-2 PLpro. But further in vitro and in vivo studies are needed to verify their potential. Collectively, it indicates that these herbal compounds can be potential antivirals against SARS-CoV-2. Further studies on the mode of interaction between PLpro and these compounds may shed light on future drug discovery.
Approved Drugs
Repurposing approved drugs is a potential alternative strategy to restrict SARS-CoV-2 infection. 6-Thioguanine (6-TG) is an orally-delivered anti-leukemia and immunosuppressant agent (Bayoumy et al., 2020). A recent study showed that 6-TG could inhibit viral replication of SARS-CoV-2 with an EC50 of 2.13 μM, which is similar to that of remdesivir and approximately 15-fold lower than that of GRL0617 (Swaim et al., 2021). Four clinically approved hepatitis C protease inhibitors (simeprevir, vaniprevir, paritaprevir, and grazoprevir) have also recently been shown to inhibit SARS-CoV-2 PLpro in vitro and viral replication in Vero-E6 cells (Bafna et al., 2021). The blockade of viral infection for these drugs display synergistic effects to the antiviral activity of remdesivir with the EC50 values ranging from 4.25 to 10.8 μM. In addition, simeprevir, vaniprevir, and grazoprevir exert dual inhibition of both Mpro and PLpro, while paritaprevir only targets PLpro (Bafna et al., 2021). Losartan is a clinically available drug used to treat several diseases including diabetic nephropathy and primary hypertension (Mulla and Siddiqui, 2021). Despite of a weak inhibitory effect on deubiquitinase or deISGylase activity of the SARS-CoV-2 PLpro, Losartan is effective to decrease viral replication and the EC50 value is 13.7 μM in the pre-infection treatment experiment (Nejat et al., 2021), suggesting that its anti-SARS-CoV-2 activity not purely depend on PLpro inhibition. Another approved drug, disulfiram, is an thiol reagent designed for alcohol aversion therapy by targeting hepatic aldehyde dehydrogenase (Lipsky et al., 2001; Krampe and Ehrenreich, 2010). It has been recently repurposed as an inhibitor of other cysteine-containing enzymes such as methyltransferase, urease, and cysteine protease of coronoviruses (Paranjpe et al., 2014; Diaz-Sanchez et al., 2016; Lin et al., 2018; Ma et al., 2020; Sargsyan et al., 2020), indicating broad-spectrum characteristics. According to pieces of evidences, disulfiram could doubly inhibit Mpro and PLpro of SARS-CoV-2 with the IC50 values of 9.35 and 7.52 μM, respectively (Sargsyan et al., 2020; Weglarz-Tomczak et al., 2021). The mechanism of action by disulfiram may involve the covalent interaction with Zn2+-bound or catalytic cysteine.
VIR250/VIR251
The peptidomimetic inhibitors VIR250 and VIR251 are the covalent inhibitors of SARS-CoV-2 PLpro identified from a combinatorial substrate library (Rut et al., 2020). These two covalent inhibitors show good inhibition against both SARS-CoV and SARS-CoV-2 PLpros with little cross-reactive to human deubiquitinating enzymes. Crystal structures of VIR250 and VIR251 in complex with PLpro were resolved to 2.79 Å and 1.65 Å, respectively (Rut et al., 2020). They have very similar molecular structures and accordingly adopt similar binding modes with PLpro (Figure 4A). Specifically, β-carbon of the glycine vinyl methyl ester (GlyVME) at the P1 position covalently links to the sulfur atom of Cys111 of SARS-CoV-2 PLpro (Figures 4B,C). In addition, P1-P3 moieties of both inhibitors engage SARS-CoV-2 PLpro mainly through polar interactions and hydrogen bonds, while P4 moiety engages PLpro through hydrophobic interactions. However, the extension of these two inhibitors at the P4 position adopts opposite orientations. Thus, additional binding space in this pocket remains to be exploited, which may inspire further drug optimization. Based on the structure of VIR251 in complex with SARS-CoV-2 PLpro, several potential inhibitors were screened against PLpro with a similar interaction mode (Delre et al., 2020; Pang et al., 2020), suggesting that VIR251, as well as VIR250, can be a good starting point for the discovery of new drugs.
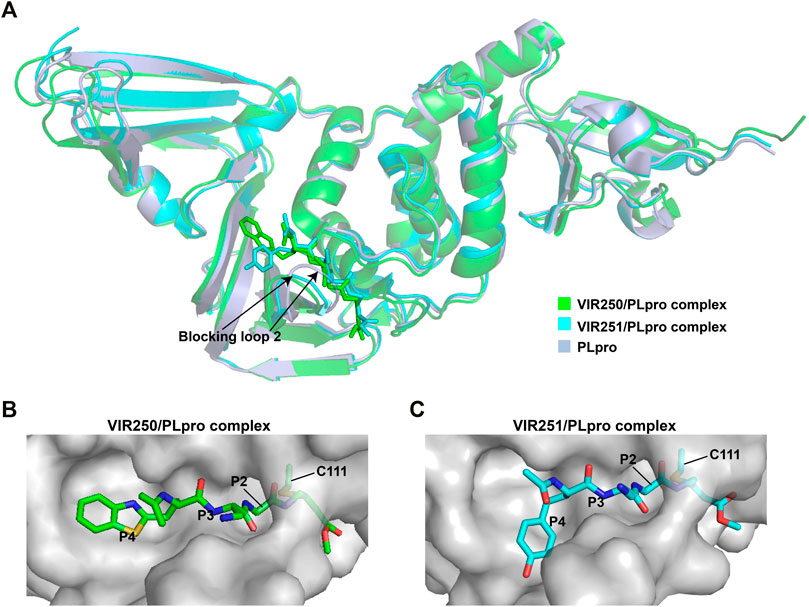
FIGURE 4. Crystal structures of SARS-CoV-2 PLpro in complex with covalent inhibitors. (A) Superposition of SARS-CoV-2 PLpro (light blue; PDB ID: 6WZU) with VIR250/PLpro (green; PDB ID: 6WUU) and VIR251/PLpro (cyan; PDB ID: 6WX4) complexes. The blocking loop 2 is indicated. (B) Surface presentation of VIR250 binding pocket. PLpro is shown as gray surface, while VIR250 is shown as sticks. The catalytic cysteine of PLpro forms a covalent bond with VIR250. The P2-P4 positions are labeled. (C) Surface presentation of VIR251 binding pocket. PLpro is shown as gray surface, while VIR251 is shown as sticks. The catalytic cysteine of PLpro forms a covalent bond with VIR251. The P2-P4 positions are labeled.
Ebselen
Ebselen is a low-molecular-weight selenoorganic drug with excellent properties in antioxidation, anti-inflammation, antiatherosclerosis, and cytoprotective effects (Azad and Tomar, 2014; Belvisi et al., 2000; Singh et al., 2017). This compound shows inhibition against Mpro and PLpro of SARS-CoV-2 with the IC50 values of 0.67 and 2.36 μM, respectively (Sargsyan et al., 2020; Ma et al., 2020; Jin et al., 2020; Weglarz-Tomczak et al., 2021), which are slightly more potent than disulfiram. In a cell-based assay, ebselen exhibits promising antiviral activity with the EC50 of 4.67 µM (Ma et al., 2020; Jin et al., 2020). The inhibition of ebselen against PLpro is an irreversible process that seems to associate with the covalent interaction between selenium atom from ebselen and sulphur atom from catalytic cysteine (Sargsyan et al., 2020; Weglarz-Tomczak et al., 2021). Subsequent modification of ebselen results in significantly increased inhibitory potency against PLpro from SARS-CoV-2. Four ebselen derivatives, namely 1d, 1e, 2d, and 2e, have IC50 constants in the nanomolar range, which is one order of magnitude lower than that of ebselen. Among these, 1d and 1e appear to be the most potent derivatives with the IC50 values of 236 and 256 nM, respectively (Table 1). The hydroxy (1d) or methoxy group (1e) substitution in the ortho position of the phenyl ring of ebselen may adopt additional interactions with the conserved residues in the active site, thus contributing to the increased potency (Weglarz-Tomczak et al., 2021). As ebselen has inhibitory activity against numerous targets, it is unlikely to be a viable candidate for further development as a clinical PLpro inhibitor.
Discussion
The outbreak of COVID-19 has caused a global concern and seriously affects the living styles of most people. PLpro, a multi-functional protease of SARS-CoV-2, can not only digest the polyprotein precursor to generate non-structural proteins but also mitigate the RIG-I-mediated innate immunity triggered by viral infection (Klemm et al., 2020; Shin et al., 2020). Its immunomodulatory effects play an important role in disease progression. Moreover, PLpro is a highly conserved protein among coronaviruses, thus is an ideal target to develop broad-spectrum inhibitors against SARS-CoV, SARS-CoV-2 and future emerged coronavirus strains. This review updates the research progress in developing novel and potent inhibitors of SARS-CoV-2 PLpro, which may reveal some insights into future drug discovery and new strategies for the COVID-19 combat.
Generally, PLpro seems to be a greatly overlooked drug target that lacks research. Numerous research efforts have focused on developing inhibitors of Mpro, another cysteine protease of SARS-CoV-2, but relatively few have focused on PLpro inhibition. Part of the reason can be that PLpro is relatively more challenging to target in the comparison of Mpro. One of the challenges is the presence of homologous host DUBs. Thus, the specificity of the PLpro inhibitor is an important parameter that deserves more attention in the PLpro inhibitor discovery.
Even though a variety of inhibitors against SARS-CoV-2 PLpro have been found as mentioned above, a large portion of hits compounds identified in high-throughput screening and virtual screening have not been further evaluated. For those newly identified inhibitors, in vitro studies in a SARS-CoV-2 setting are required to determine its potency. Many studies only evaluated the inhibition of PLpro protease activity by the inhibitor, but ignored the evaluation of its antiviral activities which significantly contribute to the ultimate drug efficacy. As far as the antiviral activity is concerned, most studies conducted this cell-based assay in vero E6 cells which is responsible for the limitation. It is better to perform the antiviral assay in human cells, such as Calu3 cells or normal human airway epithelial cells, the result of which would give different but more clinically relevant EC50 values. Moreover, little has been done to test the absorption, distribution, metabolism, and excretion (ADME) and toxicity of these inhibitors. It is necessary to establish a suitable animal model for more rigorous in vivo effect evaluation. Then subsequent clinical candidate selection can be possible.
As for mechanistic studies, few structures of inhibitors in complex with PLpro have been resolved. Co-crystallization combined with computational methods can accelerate the disclosure of interaction modes between the inhibitors and PLpro, which can inform future structure-based drug design. Some achievements have been made in the optimization of lead compounds such as GRL0617 and ebselen. Also, additional in vitro and in vivo evaluations of these potent derivatives are needed in the future.
Drug repurposing is an important strategy in drug discovery. Several existing broad-spectrum antiviral drugs such as dual inhibitors, disulfiram and ebselen, have shown robust inhibition of viral protease and are undergoing clinical trials. These drugs may favour the first line of defence for rapid response. As the high infectivity of SARS-CoV-2 has provoked continuous outbreaks all over the world, the comprehensive applications of drug repurposing, new leads identification, and structure-based leads optimization are expected to further accelerate the discovery process and develop specific inhibitors that are safe, effective and well-tolerated for COVID-19 therapy.
In conclusion, though some encouraging results are available, the drug discovery of SARS-CoV-2 PLpro is still far from meeting the needs. Future researches need to fill the above-mentioned vacancies for developing a promising clinical candidate and an effective PLpro inhibitor with both potency and selectivity eventually.
Author Contributions
HJ: conceived the topic and wrote the original draft. PY: assisted in writing and organizing the draft. JZ: conceived the topic, supervised the work and approved the final draft. All authors read and approved the final draft.
Funding
JZ was supported by the Thousand Young Talents Program of China, the National Natural Science Foundation of China (Grant No. 31770795; Grant No. 81974514), and the Jiangxi Province Natural Science Foundation (Grant No. 20181ACB20014).
Conflict of Interest
The authors declare that the research was conducted in the absence of any commercial or financial relationships that could be construed as a potential conflict of interest.
Publisher’s Note
All claims expressed in this article are solely those of the authors and do not necessarily represent those of their affiliated organizations, or those of the publisher, the editors, and the reviewers. Any product that may be evaluated in this article, or claim that may be made by its manufacturer, is not guaranteed or endorsed by the publisher.
References
Aherfi, S., Pradines, B., Devaux, C., Honore, S., Colson, P., Scola, B. L., et al. (2021). Drug Repurposing against SARS-CoV-1, SARS-CoV-2 and MERS-CoV. Future Microbiol. 16, 1341–1370. doi:10.2217/fmb-2021-0019
Aljoundi, A., Bjij, I., El Rashedy, A., and Soliman, M. E. S. (2020). Covalent versus Non-covalent Enzyme Inhibition: Which Route Should We Take? A Justification of the Good and Bad from Molecular Modelling Perspective. Protein J. 39 (2), 97–105. doi:10.1007/s10930-020-09884-2
Amin, S. A., Ghosh, K., Gayen, S., and Jha, T. (2021). Chemical-informatics Approach to COVID-19 Drug Discovery: Monte Carlo Based QSAR, Virtual Screening and Molecular Docking Study of Some In-House Molecules as Papain-like Protease (PLpro) Inhibitors. J. Biomol. Struct. Dyn. 39 (13), 4764–4773. doi:10.1080/07391102.2020.1780946
Anirudhan, V., Lee, H., Cheng, H., Cooper, L., and Rong, L. (2021). Targeting SARS‐CoV‐2 Viral Proteases as a Therapeutic Strategy to Treat COVID‐19. J. Med. Virol. 93 (5), 2722–2734. doi:10.1002/jmv.26814
Azad, G. K., and Tomar, R. S. (2014). Ebselen, a Promising Antioxidant Drug: Mechanisms of Action and Targets of Biological Pathways. Mol. Biol. Rep. 41 (8), 4865–4879. doi:10.1007/s11033-014-3417-x
Bafna, K., White, K., Harish, B., Rosales, R., Ramelot, T. A., Acton, T. B., et al. (2021). Hepatitis C Virus Drugs that Inhibit SARS-CoV-2 Papain-like Protease Synergize with Remdesivir to Suppress Viral Replication in Cell Culture. Cel Rep. 35 (7), 109133. doi:10.1016/j.celrep.2021.109133
Bayoumy, A. B., Simsek, M., Seinen, M. L., Mulder, C. J. J., Ansari, A., Peters, G. J., et al. (2020). The Continuous Rediscovery and the Benefit-Risk Ratio of Thioguanine, a Comprehensive Review. Expert Opin. Drug Metab. Toxicol. 16 (2), 111–123. doi:10.1080/17425255.2020.1719996
Belvisi, M. G., Haddad, E.-B., Battram, C., Birrell, M., Foster, M., and Webber, S. (2000). Anti-inflammatory Properties of Ebselen in a Model of Sephadex-Induced Lung Inflammation. Eur. Respir. J. 15 (3), 579–581. doi:10.1034/j.1399-3003.2000.15.25.x
Bonilla, P. J., Hughes, S. A., and Weiss, S. R. (1997). Characterization of a Second Cleavage Site and Demonstration of Activity in Trans by the Papain-like Proteinase of the Murine Coronavirus Mouse Hepatitis Virus Strain A59. J. Virol. 71 (2), 900–909. doi:10.1128/jvi.71.2.900-909.1997
Chafekar, A., and Fielding, B. (2018). MERS-CoV: Understanding the Latest Human Coronavirus Threat. Viruses 10 (2), 93. doi:10.3390/v10020093
Chen, N., Zhou, M., Dong, X., Qu, J., Gong, F., Han, Y., et al. (2020). Epidemiological and Clinical Characteristics of 99 Cases of 2019 Novel Coronavirus Pneumonia in Wuhan, China: a Descriptive Study. The Lancet 395 (10223), 507–513. doi:10.1016/s0140-6736(20)30211-7
Chen, Z., Wang, Y., Ratia, K., Mesecar, A. D., Wilkinson, K. D., and Baker, S. C. (2007). Proteolytic Processing and Deubiquitinating Activity of Papain-like Proteases of Human Coronavirus NL63. J. Virol. 81 (11), 6007–6018. doi:10.1128/jvi.02747-06
Cho, C. C., Li, S. G., Lalonde, T. J., Yang, K. S., Yu, G., Qiao, Y., et al. (2021). Drug Repurposing for the SARS-CoV-2 Papain-like Protease. ChemMedChem 17, e202100455. doi:10.1002/cmdc.202100455
Choudhury, S., Moulick, D., Borah, A., Saikia, P., and Mazumder, M. K. (2021). In Search of Drugs to Alleviate Suppression of the Host's Innate Immune Responses against SARS-CoV-2 Using a Molecular Modeling Approach. Silico Pharmacol. 9 (1), 26. doi:10.1007/s40203-021-00085-y
Chourasia, M., Koppula, P., Battu, A., Ouseph, M., and Singh, A. (2021). EGCG, a Green Tea Catechin, as a Potential Therapeutic Agent for Symptomatic and Asymptomatic SARS-CoV-2 Infection. Molecules 26 (5), 1200. doi:10.3390/molecules26051200
Cragg, G. M., and Newman, D. J. (2013). Natural Products: a Continuing Source of Novel Drug Leads. Biochim. Biophys. Acta (Bba) - Gen. Subjects 1830 (6), 3670–3695. doi:10.1016/j.bbagen.2013.02.008
Delre, P., Caporuscio, F., Saviano, M., and Mangiatordi, G. F. (2020). Repurposing Known Drugs as Covalent and Non-covalent Inhibitors of the SARS-CoV-2 Papain-like Protease. Front. Chem. 8, 594009. doi:10.3389/fchem.2020.594009
Díaz-Sánchez, Á., Alvarez-Parrilla, E., Martínez-Martínez, A., Aguirre-Reyes, L., Orozpe-Olvera, J., Ramos-Soto, M., et al. (2016). Inhibition of Urease by Disulfiram, an FDA-Approved Thiol Reagent Used in Humans. Molecules 21 (12), 1628. doi:10.3390/molecules21121628
Diniz, L. R. L., Perez-Castillo, Y., Elshabrawy, H. A., Filho, C. d. S. M. B., and de Sousa, D. P. (2021). Bioactive Terpenes and Their Derivatives as Potential SARS-CoV-2 Proteases Inhibitors from Molecular Modeling Studies. Biomolecules 11 (1), 74. doi:10.3390/biom11010074
Fehr, A. R., and Perlman, S. (2015). Coronaviruses: an Overview of Their Replication and Pathogenesis. Methods Mol. Biol. 1282, 1–23. doi:10.1007/978-1-4939-2438-7_1
Freitas, B. T., Durie, I. A., Murray, J., Longo, J. E., Miller, H. C., Crich, D., et al. (2020). Characterization and Noncovalent Inhibition of the Deubiquitinase and deISGylase Activity of SARS-CoV-2 Papain-like Protease. ACS Infect. Dis. 6 (8), 2099–2109. doi:10.1021/acsinfecdis.0c00168
Fu, Z., Huang, B., Tang, J., Liu, S., Liu, M., Ye, Y., et al. (2021). The Complex Structure of GRL0617 and SARS-CoV-2 PLpro Reveals a Hot Spot for Antiviral Drug Discovery. Nat. Commun. 12 (1), 488. doi:10.1038/s41467-020-20718-8
Gao, X., Qin, B., Chen, P., Zhu, K., Hou, P., Wojdyla, J. A., et al. (2021). Crystal Structure of SARS-CoV-2 Papain-like Protease. Acta Pharmaceutica Sinica B 11 (1), 237–245. doi:10.1016/j.apsb.2020.08.014
Gogoi, M., Borkotoky, M., Borchetia, S., Chowdhury, P., Mahanta, S., and Barooah, A. K. (2021). Black tea Bioactives as Inhibitors of Multiple Targets of SARS-CoV-2 (3CLpro, PLpro and RdRp): a Virtual Screening and Molecular Dynamic Simulation Study. J. Biomol. Struct. Dyn. 1-24. doi:10.1080/07391102.2021.1897679
Goyzueta-Mamani, L. D., Barazorda-Ccahuana, H. L., Mena-Ulecia, K., and Chávez-Fumagalli, M. A. (2021). Antiviral Activity of Metabolites from Peruvian Plants against SARS-CoV-2: An In Silico Approach. Molecules 26 (13), 3882. doi:10.3390/molecules26133882
Hajbabaie, R., Harper, M. T., and Rahman, T. (2021). Establishing an Analogue Based In Silico Pipeline in the Pursuit of Novel Inhibitory Scaffolds against the SARS Coronavirus 2 Papain-like Protease. Molecules 26 (4), 1134. doi:10.3390/molecules26041134
Hijikata, A., Shionyu, C., Nakae, S., Shionyu, M., Ota, M., Kanaya, S., et al. (2021). Current Status of Structure-Based Drug Repurposing against COVID-19 by Targeting SARS-CoV-2 Proteins. Biophysics 18, 226–240. doi:10.2142/biophysico.bppb-v18.025
Hosseini, M., Chen, W., Xiao, D., and Wang, C. (2021). Computational Molecular Docking and Virtual Screening Revealed Promising SARS-CoV-2 Drugs. Precis Clin. Med. 4 (1), 1–16. doi:10.1093/pcmedi/pbab001
Huang, C., Wang, Y., Li, X., Ren, L., Zhao, J., Hu, Y., et al. (2020). Clinical Features of Patients Infected with 2019 Novel Coronavirus in Wuhan, China. The Lancet 395 (10223), 497–506. doi:10.1016/s0140-6736(20)30183-5
Jade, D., Ayyamperumal, S., Tallapaneni, V., Joghee Nanjan, C. M., Barge, S., Mohan, S., et al. (2021). Virtual High Throughput Screening: Potential Inhibitors for SARS-CoV-2 PLPRO and 3CLPRO Proteases. Eur. J. Pharmacol. 901, 174082. doi:10.1016/j.ejphar.2021.174082
Jamalan, M., Barzegari, E., and Gholami-Borujeni, F. (2021). Structure-Based Screening to Discover New Inhibitors for Papain-like Proteinase of SARS-CoV-2: An In Silico Study. J. Proteome Res. 20 (1), 1015–1026. doi:10.1021/acs.jproteome.0c00836
James, T. W., Frias-Staheli, N., Bacik, J.-P., Levingston Macleod, J. M., Khajehpour, M., Garcia-Sastre, A., et al. (2011). Structural Basis for the Removal of Ubiquitin and Interferon-Stimulated Gene 15 by a Viral Ovarian Tumor Domain-Containing Protease. Proc. Natl. Acad. Sci. 108 (6), 2222–2227. doi:10.1073/pnas.1013388108
Jin, Z., Du, X., Xu, Y., Deng, Y., Liu, M., Zhao, Y., et al. (2020). Structure of Mpro from SARS-CoV-2 and Discovery of its Inhibitors. Nature 582 (7811), 289–293. doi:10.1038/s41586-020-2223-y
Jo, S., Kim, S., Shin, D. H., and Kim, M.-S. (2020). Inhibition of SARS-CoV 3CL Protease by Flavonoids. J. Enzyme Inhib. Med. Chem. 35 (1), 145–151. doi:10.1080/14756366.2019.1690480
Kanjanahaluethai, A., and Baker, S. C. (2000). Identification of Mouse Hepatitis Virus Papain-like Proteinase 2 Activity. J. Virol. 74 (17), 7911–7921. doi:10.1128/jvi.74.17.7911-7921.2000
Khailany, R. A., Safdar, M., and Ozaslan, M. (2020). Genomic Characterization of a Novel SARS-CoV-2. Gene Rep. 19, 100682. doi:10.1016/j.genrep.2020.100682
Klein, S., Cortese, M., Winter, S. L., Wachsmuth-Melm, M., Neufeldt, C. J., Cerikan, B., et al. (2020). SARS-CoV-2 Structure and Replication Characterized by In Situ Cryo-Electron Tomography. Nat. Commun. 11 (1), 5885. doi:10.1038/s41467-020-19619-7
Klemm, T., Ebert, G., Calleja, D. J., Allison, C. C., Richardson, L. W., Bernardini, J. P., et al. (2020). Mechanism and Inhibition of the Papain-like Protease, PLpro, of SARS-CoV-2. EMBO J. 39 (18), e106275. doi:10.15252/embj.2020106275
Knoops, K., Kikkert, M., Worm, S. H. E. v. d., Zevenhoven-Dobbe, J. C., van der Meer, Y., Koster, A. J., et al. (2008). SARS-coronavirus Replication Is Supported by a Reticulovesicular Network of Modified Endoplasmic Reticulum. Plos Biol. 6 (9), e226. doi:10.1371/journal.pbio.0060226
Kouznetsova, V. L., Zhang, A., Tatineni, M., Miller, M. A., and Tsigelny, I. F. (2020). Potential COVID-19 Papain-like Protease PLpro Inhibitors: Repurposing FDA-Approved Drugs. PeerJ 8, e9965. doi:10.7717/peerj.9965
Krampe, H., and Ehrenreich, H. (2010). Supervised Disulfiram as Adjunct to Psychotherapy in Alcoholism Treatment. Curr. Pharm. Des. 16 (19), 2076–2090. doi:10.2174/138161210791516431
Kumar, V., Parate, S., Yoon, S., Lee, G., and Lee, K. W. (2021). Computational Simulations Identified Marine-Derived Natural Bioactive Compounds as Replication Inhibitors of SARS-CoV-2. Front. Microbiol. 12, 647295. doi:10.3389/fmicb.2021.647295
Lei, J., Kusov, Y., and Hilgenfeld, R. (2018). Nsp3 of Coronaviruses: Structures and Functions of a Large Multi-Domain Protein. Antiviral Res. 149, 58–74. doi:10.1016/j.antiviral.2017.11.001
Li, L., Ma, L., Hu, Y., Li, X., Yu, M., Shang, H., et al. (2022). Natural Biflavones Are Potent Inhibitors against SARS-CoV-2 Papain-like Protease. Phytochemistry 193, 112984. doi:10.1016/j.phytochem.2021.112984
Lim, C. T., Tan, K. W., Wu, M., Ulferts, R., Armstrong, L. A., Ozono, E., et al. (2021). Identifying SARS-CoV-2 Antiviral Compounds by Screening for Small Molecule Inhibitors of Nsp3 Papain-like Protease. Biochem. J. 478 (13), 2517–2531. doi:10.1042/bcj20210244
Lin, M.-H., Moses, D. C., Hsieh, C.-H., Cheng, S.-C., Chen, Y.-H., Sun, C.-Y., et al. (2018). Disulfiram Can Inhibit MERS and SARS Coronavirus Papain-like Proteases via Different Modes. Antiviral Res. 150, 155–163. doi:10.1016/j.antiviral.2017.12.015
Lipsky, J. J., Shen, M. L., and Naylor, S. (2001). In Vivo inhibition of Aldehyde Dehydrogenase by Disulfiram. Chemico-Biological Interactions 130-132 (1-3), 93–102. doi:10.1016/s0009-2797(00)00225-8
Ma, C., Hu, Y., Townsend, J. A., Lagarias, P. I., Marty, M. T., Kolocouris, A., et al. (2020). Ebselen, Disulfiram, Carmofur, PX-12, Tideglusib, and Shikonin Are Nonspecific Promiscuous SARS-CoV-2 Main Protease Inhibitors. ACS Pharmacol. Transl. Sci. 3 (6), 1265–1277. doi:10.1021/acsptsci.0c00130
Ma, C., Sacco, M. D., Xia, Z., Lambrinidis, G., Townsend, J. A., Hu, Y., et al. (2021). Discovery of SARS-CoV-2 Papain-like Protease Inhibitors through a Combination of High-Throughput Screening and a FlipGFP-Based Reporter Assay. ACS Cent. Sci. 7 (7), 1245–1260. doi:10.1021/acscentsci.1c00519
Madewell, Z. J., Yang, Y., Longini, I. M., Halloran, M. E., and Dean, N. E. (2020). Household Transmission of SARS-CoV-2. JAMA Netw. Open 3 (12), e2031756. doi:10.1001/jamanetworkopen.2020.31756
McClain, C. B., and Vabret, N. (2020). SARS-CoV-2: the many Pros of Targeting PLpro. Sig Transduct Target. Ther. 5 (1), 223. doi:10.1038/s41392-020-00335-z
Mielech, A. M., Chen, Y., Mesecar, A. D., and Baker, S. C. (2014). Nidovirus Papain-like Proteases: Multifunctional Enzymes with Protease, Deubiquitinating and deISGylating Activities. Virus. Res. 194, 184–190. doi:10.1016/j.virusres.2014.01.025
Mslati, H., Gentile, F., Perez, C., and Cherkasov, A. (2021). Comprehensive Consensus Analysis of SARS-CoV-2 Drug Repurposing Campaigns. J. Chem. Inf. Model. 61 (8), 3771–3788. doi:10.1021/acs.jcim.1c00384
Naidoo, D., Kar, P., Roy, A., Mutanda, T., Bwapwa, J., Sen, A., et al. (2021). Structural Insight into the Binding of Cyanovirin-N with the Spike Glycoprotein, Mpro and PLpro of SARS-CoV-2: Protein-Protein Interactions, Dynamics Simulations and Free Energy Calculations. Molecules 26 (17), 5114. doi:10.3390/molecules26175114
Nejat, R., Sadr, A. S., Freitas, B., Crabttree, J., Pegan, S. D., Tripp, R. A., et al. (2021). Losartan Inhibits SARS-CoV-2 Replication In Vitro. J. Pharm. Pharm. Sci. 24, 390–399. doi:10.18433/jpps31931
Osipiuk, J., Azizi, S.-A., Dvorkin, S., Endres, M., Jedrzejczak, R., Jones, K. A., et al. (2021). Structure of Papain-like Protease from SARS-CoV-2 and its Complexes with Non-covalent Inhibitors. Nat. Commun. 12 (1), 743. doi:10.1038/s41467-021-21060-3
Pang, J., Gao, S., Sun, Z., and Yang, G. (2020). Discovery of Small Molecule PLpro Inhibitor against COVID-19 Using Structure-Based Virtual Screening, Molecular Dynamics Simulation, and Molecular mechanics/Generalized Born Surface Area (MM/GBSA) Calculation. Struct. Chem. 1-8, 1–8. doi:10.1007/s11224-020-01665-y
Paranjpe, A., Zhang, R., Ali-Osman, F., Bobustuc, G. C., and Srivenugopal, K. S. (2014). Disulfiram Is a Direct and Potent Inhibitor of Human O6-Methylguanine-DNA Methyltransferase (MGMT) in Brain Tumor Cells and Mouse Brain and Markedly Increases the Alkylating DNA Damage. Carcinogenesis 35 (3), 692–702. doi:10.1093/carcin/bgt366
Patel, K. P., Vunnam, S. R., Patel, P. A., Krill, K. L., Korbitz, P. M., Gallagher, J. P., et al. (2020). Transmission of SARS-CoV-2: an Update of Current Literature. Eur. J. Clin. Microbiol. Infect. Dis. 39 (11), 2005–2011. doi:10.1007/s10096-020-03961-1
Perng, Y.-C., and Lenschow, D. J. (2018). ISG15 in Antiviral Immunity and beyond. Nat. Rev. Microbiol. 16 (7), 423–439. doi:10.1038/s41579-018-0020-5
Pushpakom, S., Iorio, F., Eyers, P. A., Escott, K. J., Hopper, S., Wells, A., et al. (2019). Drug Repurposing: Progress, Challenges and Recommendations. Nat. Rev. Drug Discov. 18 (1), 41–58. doi:10.1038/nrd.2018.168
Quimque, M. T. J., Notarte, K. I. R., Fernandez, R. A. T., Mendoza, M. A. O., Liman, R. A. D., Lim, J. A. K., et al. (2021). Virtual Screening-Driven Drug Discovery of SARS-CoV2 Enzyme Inhibitors Targeting Viral Attachment, Replication, post-translational Modification and Host Immunity Evasion Infection Mechanisms. J. Biomol. Struct. Dyn. 39 (12), 4316–4333. doi:10.1080/07391102.2020.1776639
Rahul, S., and Sarkar, A. (2021). Microbial Based Natural Compounds as Potential Inhibitors for SARS-CoV-2 Papain-like Protease (PLpro): a Molecular Docking and Dynamic Simulation Study. J. Biomol. Struct. Dyn. 1-11. doi:10.1080/07391102.2021.1997815
Ratia, K., Pegan, S., Takayama, J., Sleeman, K., Coughlin, M., Baliji, S., et al. (2008). A Noncovalent Class of Papain-like Protease/deubiquitinase Inhibitors Blocks SARS Virus Replication. Proc. Natl. Acad. Sci. 105 (42), 16119–16124. doi:10.1073/pnas.0805240105
Ruan, S. (2020). Likelihood of Survival of Coronavirus Disease 2019. Lancet Infect. Dis. 20 (6), 630–631. doi:10.1016/s1473-3099(20)30257-7
Rudrapal, M., Issahaku, A. R., Agoni, C., Bendale, A. R., Nagar, A., Soliman, M. E. S., et al. (2021). In Silico screening of Phytopolyphenolics for the Identification of Bioactive Compounds as Novel Protease Inhibitors Effective against SARS-CoV-2. J. Biomol. Struct. Dyn. 1-17. doi:10.1080/07391102.2021.1944909
Rut, W., Lv, Z., Zmudzinski, M., Patchett, S., Nayak, D., Snipas, S. J., et al. (2020). Activity Profiling and crystal Structures of Inhibitor-Bound SARS-CoV-2 Papain-like Protease: A Framework for Anti-COVID-19 Drug Design. Sci. Adv. 6 (42), eabd4596. doi:10.1126/sciadv.abd4596
Sargsyan, K., Lin, C.-C., Chen, T., Grauffel, C., Chen, Y.-P., Yang, W.-Z., et al. (2020). Multi-targeting of Functional Cysteines in Multiple Conserved SARS-CoV-2 Domains by Clinically Safe Zn-Ejectors. Chem. Sci. 11 (36), 9904–9909. doi:10.1039/d0sc02646h
Shamsi, A., Mohammad, T., Anwar, S., Amani, S., Khan, M. S., Husain, F. M., et al. (2021). Potential Drug Targets of SARS-CoV-2: From Genomics to Therapeutics. Int. J. Biol. Macromolecules 177, 1–9. doi:10.1016/j.ijbiomac.2021.02.071
Shan, H., Liu, J., Shen, J., Dai, J., Xu, G., Lu, K., et al. (2021). Development of Potent and Selective Inhibitors Targeting the Papain-like Protease of SARS-CoV-2. Cel Chem. Biol. 28 (6), 855–865. doi:10.1016/j.chembiol.2021.04.020
Shen, Z., Ratia, K., Cooper, L., Kong, D., Lee, H., Kwon, Y., et al. (2021). Design of SARS-CoV-2 PLpro Inhibitors for COVID-19 Antiviral Therapy Leveraging Binding Cooperativity. J. Med. Chem. doi:10.1021/acs.jmedchem.1c01307
Shende, P., Khanolkar, B., and Gaud, R. S. (2021). Drug Repurposing: New Strategies for Addressing COVID-19 Outbreak. Expert Rev. Anti-infective Ther. 19 (6), 689–706. doi:10.1080/14787210.2021.1851195
Shin, D., Mukherjee, R., Grewe, D., Bojkova, D., Baek, K., Bhattacharya, A., et al. (2020). Papain-like Protease Regulates SARS-CoV-2 Viral Spread and Innate Immunity. Nature 587 (7835), 657–662. doi:10.1038/s41586-020-2601-5
Singh, V. P., Poon, J.-f., Yan, J., Lu, X., Ott, M. K., Butcher, R. J., et al. (2017). Nitro-, Azo-, and Amino Derivatives of Ebselen: Synthesis, Structure, and Cytoprotective Effects. J. Org. Chem. 82 (1), 313–321. doi:10.1021/acs.joc.6b02418
Smith, E., Davis-Gardner, M. E., Garcia-Ordonez, R. D., Nguyen, T.-T., Hull, M., Chen, E., et al. (2020). High-Throughput Screening for Drugs that Inhibit Papain-like Protease in SARS-CoV-2. SLAS DISCOVERY: Advancing Sci. Drug Discov. 25 (10), 1152–1161. doi:10.1177/2472555220963667
Solnier, J., and Fladerer, J. P. (2020). Flavonoids: A Complementary Approach to Conventional Therapy of COVID-19? Phytochem. Rev., 1–23. doi:10.1007/s11101-020-09720-6
Stadler, K., Masignani, V., Eickmann, M., Becker, S., Abrignani, S., Klenk, H.-D., et al. (2003). SARS - Beginning to Understand a New Virus. Nat. Rev. Microbiol. 1 (3), 209–218. doi:10.1038/nrmicro775
Stasiulewicz, A., Maksymiuk, A. W., Nguyen, M. L., Bełza, B., and Sulkowska, J. I. (2021). SARS-CoV-2 Papain-like Protease Potential Inhibitors-In Silico Quantitative Assessment. Ijms 22 (8), 3957. doi:10.3390/ijms22083957
Su, S., Wong, G., Shi, W., Liu, J., Lai, A. C. K., Zhou, J., et al. (2016). Epidemiology, Genetic Recombination, and Pathogenesis of Coronaviruses. Trends Microbiol. 24 (6), 490–502. doi:10.1016/j.tim.2016.03.003
Swaim, C. D., Canadeo, L. A., Monte, K. J., Khanna, S., Lenschow, D. J., and Huibregtse, J. M. (2020). Modulation of Extracellular ISG15 Signaling by Pathogens and Viral Effector Proteins. Cel Rep. 31 (11), 107772. doi:10.1016/j.celrep.2020.107772
Swaim, C. D., Dwivedi, V., Perng, Y.-C., Zhao, X., Canadeo, L. A., Harastani, H. H., et al. (2021). 6-Thioguanine Blocks SARS-CoV-2 Replication by Inhibition of PLpro. iScience 24 (10), 103213. doi:10.1016/j.isci.2021.103213
Swatek, K. N., Aumayr, M., Pruneda, J. N., Visser, L. J., Berryman, S., Kueck, A. F., et al. (2018). Irreversible Inactivation of ISG15 by a Viral Leader Protease Enables Alternative Infection Detection Strategies. Proc. Natl. Acad. Sci. USA 115 (10), 2371–2376. doi:10.1073/pnas.1710617115
Tian, D., Liu, Y., Liang, C., Xin, L., Xie, X., Zhang, D., et al. (2021). An Update Review of Emerging Small-Molecule Therapeutic Options for COVID-19. Biomed. Pharmacother. 137, 111313. doi:10.1016/j.biopha.2021.111313
Tong, T., Wu, Y.-Q., Ni, W.-J., Shen, A.-Z., and Liu, S. (2020). The Potential Insights of Traditional Chinese Medicine on Treatment of COVID-19. Chin. Med. 15, 51. doi:10.1186/s13020-020-00326-w
V. C. Guido, R., Oliva, G., and D. Andricopulo, A. (2011). Modern Drug Discovery Technologies: Opportunities and Challenges in lead Discovery. Cchts 14 (10), 830–839. doi:10.2174/138620711797537067
Wang, C., Horby, P. W., Hayden, F. G., and Gao, G. F. (2020). A Novel Coronavirus Outbreak of Global Health Concern. The Lancet 395 (10223), 470–473. doi:10.1016/s0140-6736(20)30185-9
Wang, T.-y., Li, Q., and Bi, K.-s. (2018). Bioactive Flavonoids in Medicinal Plants: Structure, Activity and Biological Fate. Asian J. Pharm. Sci. 13 (1), 12–23. doi:10.1016/j.ajps.2017.08.004
Wang, Z., Yang, L., and Zhao, X.-E. (2021). Co-crystallization and Structure Determination: An Effective Direction for Anti-SARS-CoV-2 Drug Discovery. Comput. Struct. Biotechnol. J. 19, 4684–4701. doi:10.1016/j.csbj.2021.08.029
Weglarz-Tomczak, E., Tomczak, J. M., Talma, M., Burda-Grabowska, M., Giurg, M., and Brul, S. (2021). Identification of Ebselen and its Analogues as Potent Covalent Inhibitors of Papain-like Protease from SARS-CoV-2. Sci. Rep. 11 (1), 3640. doi:10.1038/s41598-021-83229-6
Woo, P. C. Y., Huang, Y., Lau, S. K. P., and Yuen, K.-Y. (2010). Coronavirus Genomics and Bioinformatics Analysis. Viruses 2 (8), 1804–1820. doi:10.3390/v2081803
Wu, A., Peng, Y., Huang, B., Ding, X., Wang, X., Niu, P., et al. (2020b). Genome Composition and Divergence of the Novel Coronavirus (2019-nCoV) Originating in China. Cell Host & Microbe 27 (3), 325–328. doi:10.1016/j.chom.2020.02.001
Wu, F., Zhao, S., Yu, B., Chen, Y.-M., Wang, W., Song, Z.-G., et al. (2020a). A New Coronavirus Associated with Human Respiratory Disease in China. Nature 579 (7798), 265–269. doi:10.1038/s41586-020-2008-3
Wu, Z., and McGoogan, J. M. (2020). Characteristics of and Important Lessons from the Coronavirus Disease 2019 (COVID-19) Outbreak in China. JAMA 323 (13), 1239–1242. doi:10.1001/jama.2020.2648
Xu, Y., Chen, K., Pan, J., Lei, Y., Zhang, D., Fang, L., et al. (2021). Repurposing Clinically Approved Drugs for COVID-19 Treatment Targeting SARS-CoV-2 Papain-like Protease. Int. J. Biol. Macromolecules 188, 137–146. doi:10.1016/j.ijbiomac.2021.07.184
Yadav, R., Chaudhary, J. K., Jain, N., Chaudhary, P. K., Khanra, S., Dhamija, P., et al. (2021). Role of Structural and Non-structural Proteins and Therapeutic Targets of SARS-CoV-2 for COVID-19. Cells 10 (4), 821. doi:10.3390/cells10040821
Ye, Z.-W., Yuan, S., Yuen, K.-S., Fung, S.-Y., Chan, C.-P., and Jin, D.-Y. (2020). Zoonotic Origins of Human Coronaviruses. Int. J. Biol. Sci. 16 (10), 1686–1697. doi:10.7150/ijbs.45472
Yosri, N., Abd El-Wahed, A. A., Ghonaim, R., Khattab, O. M., Sabry, A., Ibrahim, M. A. A., et al. (2021). Anti-Viral and Immunomodulatory Properties of Propolis: Chemical Diversity, Pharmacological Properties, Preclinical and Clinical Applications, and In Silico Potential against SARS-CoV-2. Foods 10 (8), 1776. doi:10.3390/foods10081776
Zhang, D.-h., Wu, K.-l., Zhang, X., Deng, S.-q., and Peng, B. (2020b). In Silico screening of Chinese Herbal Medicines with the Potential to Directly Inhibit 2019 Novel Coronavirus. J. Integr. Med. 18 (2), 152–158. doi:10.1016/j.joim.2020.02.005
Zhang, R. H., Ai, X., Liu, Y., Li, C. H., and Zhang, H. L. (2020a). Genomic Characterization and Phylogenetic Evolution of the SARS-CoV-2. Acta Virol. 64 (4), 496–500. doi:10.4149/av_2020_403
Zhao, Y., Du, X., Duan, Y., Pan, X., Sun, Y., You, T., et al. (2021). High-throughput Screening Identifies Established Drugs as SARS-CoV-2 PLpro Inhibitors. Protein Cell 12 (11), 877–888. doi:10.1007/s13238-021-00836-9
Zheng, Y., and Gao, C. (2019). E3 Ubiquitin Ligases, the Powerful Modulator of Innate Antiviral Immunity. Cell Immunol. 340, 103915. doi:10.1016/j.cellimm.2019.04.003
Zheng, Y., and Gao, C. (2020). Fine-tuning of Antiviral Innate Immunity by Ubiquitination. Adv. Immunol. 145, 95–128. doi:10.1016/bs.ai.2019.11.004
Zhou, P., Yang, X.-L., Wang, X.-G., Hu, B., Zhang, L., Zhang, W., et al. (2020). A Pneumonia Outbreak Associated with a New Coronavirus of Probable Bat Origin. Nature 579 (7798), 270–273. doi:10.1038/s41586-020-2012-7
Keywords: COVID-19, SARS-CoV-2, papain-like protease, inhibitor, crystal structure
Citation: Jiang H, Yang P and Zhang J (2022) Potential Inhibitors Targeting Papain-Like Protease of SARS-CoV-2: Two Birds With One Stone. Front. Chem. 10:822785. doi: 10.3389/fchem.2022.822785
Received: 26 November 2021; Accepted: 28 January 2022;
Published: 23 February 2022.
Edited by:
Jun Wang, The State University of New Jersey, United StatesReviewed by:
Jerry M. Parks, Oak Ridge National Laboratory (DOE), United StatesM. Joanne Lemieux, University of Alberta, Canada
Copyright © 2022 Jiang, Yang and Zhang. This is an open-access article distributed under the terms of the Creative Commons Attribution License (CC BY). The use, distribution or reproduction in other forums is permitted, provided the original author(s) and the copyright owner(s) are credited and that the original publication in this journal is cited, in accordance with accepted academic practice. No use, distribution or reproduction is permitted which does not comply with these terms.
*Correspondence: Haihai Jiang, aGFpaGFpamlhbmcyMDIwQG5jdS5lZHUuY24=; Jin Zhang, emhhbmd4aWFva29uZ0Bob3RtYWlsLmNvbQ==
†These authors contribute equally to this work