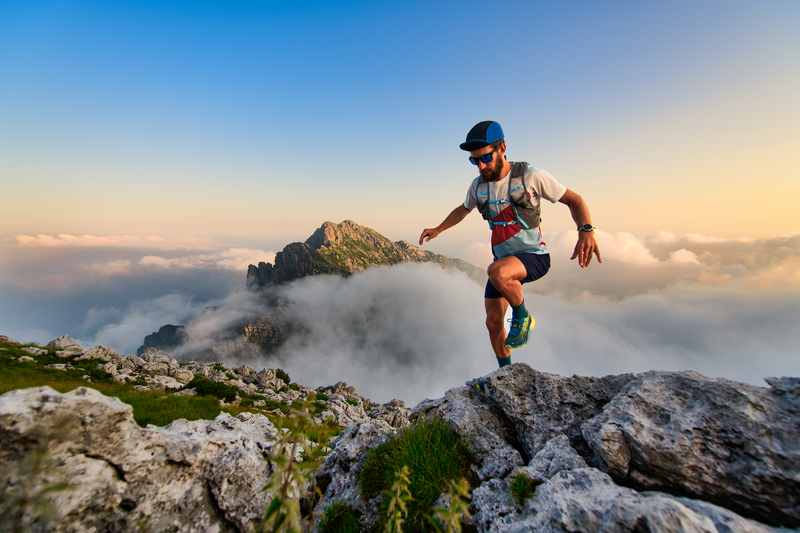
94% of researchers rate our articles as excellent or good
Learn more about the work of our research integrity team to safeguard the quality of each article we publish.
Find out more
REVIEW article
Front. Chem. , 11 April 2022
Sec. Solid State Chemistry
Volume 10 - 2022 | https://doi.org/10.3389/fchem.2022.802890
This article is part of the Research Topic Reviews in Chemistry View all 14 articles
Parts of this article's content have been modified or rectified in:
Erratum: A perspective on perovskite solar cells: emergence, progress, and commercialization
With rapid progress in light-to-electric conversion efficiencies, perovskite solar cells (PSCs) have exhibited great potential as next-generation low-cost, efficient photovoltaic technology. In this perspective, we briefly review the development of PSCs from discovery to laboratory research to commercializing progress. The past several decades have witnessed great achievement in device efficiency and stability due to tremendous research efforts on compositional, process, and interfacial engineering. Regarding commercial applications, we expound the merits and disadvantages of PSCs compared to the existing silicon photovoltaic technologies. Although PSCs promise solution processability and low manufacturing cost, their limited stability and element toxicity should to be addressed on the path to commercialization. Finally, we provide future perspectives on commercialization of PSCs in the photovoltaic marketplace. It is suggested that PSCs will be more promising in low-cost modules and tandem configurations.
Metal halide perovskites (MHPs) have attracted intensive attention as promising photovoltaic materials during the last few decades. The term of “Perovskite” is employed to describe a class of materials with the same crystal structure as the mineral calcium titanate (CaTiO3), which was firstly discovered in the Ural Mountains of Russia in 1839 and named after Lev Perovski, a renowned Russian mineralogist (Chakhmouradian and Woodward, 2014). Now, MHPs have developed into a broad range of materials with the general formula ABX3, where A and B are monovalent and divalent cations, respectively and X stands for anions. Besides, MHPs have been widely used as active materials in various optical and electronic applications, such as photovoltaic devices (PV) (Kojima et al., 2009; Kim et al., 2012; Lee et al., 2012; Jeong et al., 2021), light-emitting diode (Cho et al., 2015; Cao et al., 2018; Lin et al., 2018), photodetector (Li et al., 2020a; Li et al., 2020b), laser (Sutherland and Sargent, 2016; Wei et al., 2019), sensor (Zaza et al., 2019; George et al., 2020), biomedicine (Fang et al., 2021; Zhang et al., 2021; Fang et al., 2022) etc.
The first demonstration of the photovoltaic effect on perovskite materials dates back to 2009 by Miyaska and his co-works, but the power conversion efficiency (PCE) was only 3.8% (Kojima et al., 2009). Although the performance of perovskite solar cells (PSCs) was low at that time, the strong optical absorption of perovskite materials attracted widespread attention in academic circles. However, these cells suffered from rapid degradation of perovskite materials in the liquid electrolyte. In 2012, important breakthroughs in PSCs were realized by (Kim et al., 2012; Lee et al., 2012). In these works, all-solid device configurations were reported with Spiro-MeOTAD [2,2′,7,7′-tetrakis (N,N-di-p-methoxyphenylamine)-9,9′-spirobifluorene] as the hole transport layer to solve the instability problem in liquid electrolytes. The PCEs of about 10% have been reported with improved operation stability. Now, the most recent world record for single-junction PSCs has reached 25.6%, claimed by researchers at South Korea’s Ulsan National Institute of Science and Technology (UNIST) (Jeong et al., 2021). As demonstrated in Figure 1, the laboratory efficiency of PSCs is comparable to that of the first-generation monocrystalline silicon solar cell that takes about 40 years for this level.
Figure 1. Progress of cell efficiency in single crystal and multicrystalline Si cells, perovskite solar cells, perovskite/Si tandem according to Best Research-Cell Efficiency chart from National Renewable Energy Laboratory (NREL, 2020).
In addition, perovskites have been demonstrated as promising candidates in multi-junction cells due their easily tunable bandgap through constituents (Al-Ashouri et al., 2020; Chen et al., 2021; Chen et al., 2022; Lin et al., 2022; Qin et al., 2022). The state-of-the-art perovskite-on-silicon tandem solar cell has achieved a PCE of 29.52% in Oxford PV on approximately 30 × 30 cm2 device area (Al-Ashouri et al., 2020). More recently, the all-perovskite tandem solar cell with a certified efficiency of 26.4% has also been reported by (Lin et al., 2022).
Perovskite materials are excellent light absorbers with outstanding optoelectronic properties such as high light absorption coefficient, long carrier diffusion length (exceeding 1 mm), low non-radiative recombination loss and high defect tolerance (Stranks et al., 2013; Xing et al., 2013; Eperon et al., 2014; Green et al., 2014). The theoretical limit of single-junction PSCs is about 33%, and that of tandem solar cells can reach over 40%. Along with ease of accessibility through low-cost solution processes, there is no doubt that PSCs have become one of the most promising photovoltaic technologies. Since reported in 2009, it was rated as one of the ten scientific breakthroughs by Science. Next year, it was elected as one of the most anticipated scientific and technological breakthroughs by Nature. In the World Economic Forum of 2016, PSCs were honored as one of the top 10 emerging technologies that would break the limitations of silicon-based photovoltaics. In a world, perovskite materials have become a hot topic in both academic and industrial fields.
Since reported in 2009, intensive research efforts have been made to improve the efficiency of PSCs, including compositional, process and interfacial engineering. Perovskite photovoltaic materials are ionic compounds in the general formula of ABX3, as shown in Figure 2A. The crystallographic structure can be deduced by the Goldschmidt tolerance factor (
Figure 2. (A) Cubic perovskite structure with ABX3. Adapted from Green et al. (2014) with permission from Nature springer. (B) UV-vis absorption spectra of PSCs with mixing halide ions and corresponding colorful films. Adapted form Noh et al. (2013) with permission from American Chemical Society.
The optoelectronic properties of MHPs are strongly correlated with ion species. Firstly, the bandgap can be continuously tuned in the range of almost the whole visible spectrum by X-site halide mixing (Noh et al., 2013; Eperon et al., 2014). As shown in Figure 2B, the absorption edge moves from 786 to 544 nm with the substitution of I with Br ions, corresponding to the bandgap increasing from 1.58 to 2.28 eV. The bandgap engineering by halide ions provides an effective strategy to design wide-bandgap perovskites into tandem solar cells. Another significant ion substitution is replacing the MA+ by FA+. Due to the larger ion size of FA+, the replacement expands the perovskite crystal and shortens the Pb-I bond distance, resulting in the reduced bandgap of 1.47 eV. The use of FA+ as the A-site cation in MHPs further increases photocurrents. Unfortunately, phase stability of FAPbI3 still depends on the following chemical management according to Goldschmidt rule. At the ambient conditions, the photoactive α-FAPbI3 is unstable and easily transforms into the yellow δ phase, which seriously deteriorates the device performance. To solve this problem, new strategies are needed for stabilizing the α-phase in FA-based PSCs. Seok and co-workers firstly stabilized α-phase FAPbI3 by incorporation of MAPbBr3 and achieved a PCE of more than 18% PCE (Jeon et al., 2015). Later on, A site cation was further optimized as a “cation cascade” by mixing FA+, MA+ and Rb+ to eliminate the residual δ-phase by Saliba and his co-workers (Saliba et al., 2016). By this method, FA-based PSCs can deliver a stabilized PCE of over 21% and a long shelf lifetime of over 1,000 h. However, the use of MA+, Rb+ and Br− into FAPbI3 perovskites can inevitably widen the bandgap, limiting the device performance. Instead, an anion engineering was introduced to stabilize pure phase FAPbI3 with the pseudo-halide anion (HCOO−) passivating the I- vacancy defects. The resulting solar cells have world-record efficiency of 25.6% and long-term stability over 450 h (Jeong et al., 2021).
The prerequisite for high-efficiency device is to fabricate high-quality perovskite films with a flat and compact morphology. To date, numerous processing techniques have been developed to prepare high-quality perovskite films, including vacuum deposition, one-step solution deposition (antisolvent dripping, solvent-assisted annealing, and precursor engineering), two-step sequential deposition and vapor-assisted deposition. Among these methods, solution processes are promising with low temperature and cost, compatible with flexible electronics. Furthermore, scaling-up methods (slot-die coating, roll-to-roll, and inkjet printing) have also been developed for the industrial manufacturing of PSCs in the future.
The vacuum deposition method was firstly reported by Mitzi and co-workers, in which organic-inorganic compounds were rapidly heated to sublimate, then deposited on the substrate (Mitzi et al., 1999). This technique allows facile control over the film composition and thickness with high reproducibility. With the rapid development of PSCs, Snaith et al. employed dual-source co-evaporation to deposit a uniform MAPbI3-xClx perovskite layer and a PCE of 15% was reported (Figure 3A) (Liu et al., 2013). The perovskite films fabricated by vacuum deposition were proven to be high-quality with excellent film uniformity. Although the vacuum deposition requires expensive equipment, it still holds the advantages of fabricating large-area films without using toxic solvents. This makes it very promising in the production of the perovskite module. Recently, vacuum deposition has witnessed further progress in the perovskites materials including mixed halide (Longo et al., 2018), narrow-bandgap (Igual-Muñoz et al., 2020), and formamidine based perovskite solar cells (Chiang et al., 2020; Feng et al., 2021). The champion efficiency of 21.32% and 18.13% has been reported on the small-size cell (Feng et al., 2021) and mini-modules (effective device area 21 cm2) (Li J. et al., 2020), respectively.
Figure 3. (A) Dual-source thermal evaporation. Adapted from Liu et al. (2013) with permission from Nature springer. (B) One-step and two-step solution process for perovskite film growth. Adapted from Im et al. (2014) with permission from Nature springer. (C) Perovskite film formation through vapor-assisted deposition. Reproduced from Chen et al. (2014) with permission from American Chemical Society.
One-step solution deposition is a commonly used method for fabricating perovskite films in labs. In this method, the precursor solutions are prepared by mixing precursors in solvents such as N, N-dimethylformamide (DMF), then spin-coated on the substrates, followed by thermal annealing to initialize the crystallization (Im et al., 2014). To obtain flat and compact films without pin-holes, antisolvents are usually used to induce homogenous nucleation, for example, toluene (Jeon et al., 2014), chlorobenzene (Bi et al., 2016), or diethyl ether (Li et al., 2017; Gao et al., 2018). One-step deposition is simple to conduct in labs and antisolvents can serve as the carriers for Lewis acid and base additives, which has been demonstrated as an effective strategy for defect passivation. For example, Wu et al. achieved highly efficient inverted PSCs with a perovskite-fullerene graded heterojunction formed by dripping the antisolvent containing fullerenes (Wu et al., 2016). Subsequently, conjugated polymers dissolved in antisolvents were introduced in perovskite films to reduce trap states and non-radiative recombination loss (Rajagopal et al., 2017; Qin et al., 2018; Yang et al., 2018; Chen et al., 2019a; Wu et al., 2019).
Besides one-step solution deposition, two-step sequential deposition is another commonly used method to obtain perovskite films with improved reproducibility (Burschka et al., 2013; Im et al., 2014). As firstly reported by Burschka et al., PbI2 was spin-coated on the substrates followed by sequential deposition of MAI, then the formation of the perovskite was accomplished by thermal annealing, as depicted in Figure 3B. Compared with one-step solution deposition, two-step deposition has better control over the film morphology by relieving effects from the surrounding environment. However, the conversion of PbI2 to the perovskite remains a major challenge to achieve highly efficient and stable devices (Zhang et al., 2015; Chen, 2017; Chiang et al., 2017; Xu et al., 2019). The residual PbI2 at the interfaces was suggested to impede the carrier transportation and induce severe degradation PSCs (Jiang et al., 2016).
This method was firstly proposed by Chen et al. in which in-situ reaction between the as-deposited PbI2 (solid) and MAI vapor was adopted to obtain perovskite films with full coverage and smooth surface, as schematically illustrated in Figure 3C (Chen et al., 2014). The vapor-assisted process is a facile low-temperature method with the slowdown in nucleation and film growth, which delivers increasing grain size and high reproducibility. In addition, vapor-assisted deposition provides the possibility of growing high-quality perovskites on curvature or textured substrates. Zou et al. demonstrated a high-quality perovskite film grown on a fibrous substrate by the improved vapor-assisted deposition and the fiber-shaped perovskite solar cell with a PCE of 10.79% was obtained (Dong et al., 2019).
For commercializing PSCs, the large-scale process method has attracted more attention in recent years. With the development of large-area coating methods, including blade, slot-die and spray coating, the roll-roll (R2R) printed process has been suggested to realize the upscaling of PSCs with low cost and high-throughput (Yang et al., 2021). At first, a sequential slot-die method was developed to fabricate large-area PSCs with a PCE up to 11.96% (Hwang et al., 2015). The process was demonstrated to be incorporated into R2R printing. During the past few years, some researchers tried to produce PSCs by the R2R printed process, but only partial processes were reported at laboratory scale (Dou et al., 2018; Galagan et al., 2018; Kim J. E. et al., 2019; Kim Y. Y. et al., 2019). More recently, Kim et al. demonstrated the fully R2R manufacturing of PSCs with PCEs of 23.5% and 19.1% on planar and flexible substrates, respectively. This work suggested the gravure R2R printed process as a promising method to upscaling PSCs in the near future (Kim et al., 2020; Yang et al., 2021).
Besides compositional and process engineering, interfacial engineering has also contributed to the rapid progress in the efficiency and stability of PSCs. Interfacial engineering mainly focuses on charge transport layers (CTLs) together with interfacial modifications. CTLs play important roles in PSCs, which can not only promote the photogenerated carrier extraction but also suppress the non-radiative recombination. An effective CTL should meet the following requirements: first, matched energy level for the charge extraction from perovskite layer; second, high transparency to avoid energy loss of solar light; and finally, high hole/electron mobility, and the blocking ability of the counter carrier (Ouyang et al., 2019).
Mesoporous TiO2 and small molecule Spiro-MeOTAD were used as effective electron transport layer (ETL) and hole transport layer (HTL) in the pioneering works (Kim et al., 2012; Lee et al., 2012). Significantly, the use of Spiro-MeOTAD solved the corrosive problem of liquid electrolytes, making all-solid cells. However, Spiro-MeOTAD remains far inferior to commercial photovoltaic cells. Under continuous illumination, doped lithium salt tends to cause aggregation of Spiro-MeOTAD, leading to a rapid decrease in the device efficiency (Abate et al., 2013). On the other side, mesoporous TiO2 induces fast decay due to exposed oxygen vacancies under ultraviolet light (Leijtens et al., 2013). Therefore, more stable CTLs with effective charge extraction are still required to address the stability issue. Up till now, a great number of CTLs have been studied to deliver more efficient and stable PSCs, including small molecules, polymers and inorganic materials. In terms of long-term operational stability, inorganic materials would be more suitable for the commercialization of PSCs due to chemical inertness and low cost. You et al. demonstrated air-stable PSCs with p-type NiOx and n-type ZnO as HTL and ETL, respectively. The all-metal-oxide devices retain about 90% of the initial efficiency after 60 days of storage at room temperature (You et al., 2016). Fullerene-derived carbon materials such as PCBM and C60 have been developed as promising ETLs in inverted planar structures (Jeng et al., 2013). Besides, a broad range of metal oxides [ZnO (Liu and Kelly, 2014; Cheng et al., 2015), SnO2 (Jiang et al., 2016; Jiang et al., 2018), Zn2SnO4 (Shin et al., 2015) as ETLs and NiOx (Jiang et al., 2015; Kim et al., 2015; Zhang et al., 2016), NiCo2O4 (Ouyang et al., 2018), CuGaO2 (Chen et al., 2018) as HTLs] are used in PSCs and deliver improved efficiency and stability.
An important aspect of interfacial engineering is the impact of CTLs on perovskite formation. According to the Gibbs free energy [∆G_het = ∆G_hom × f(θ)], nucleation is determined by the interfacial free energy ∆G and wetting angle θ. Adjusting surface energy can control the nucleation and growth process of perovskite materials. Generally, hydrophilic CTLs lead to homogenous nucleation, resulting in perovskite films with high coverage and uniformity. On the contrary, hydrophobic surfaces reduce the nucleation, favoring the growth of large grain size. Huang et al. reported the growth of perovskite grains on non-wetting surfaces (Bi et al., 2015). The large-size perovskite grain featured a high grain size/thickness aspect ratio reaching 2.3–7.9, surpassing the limitation of the film thickness (Figure 4A).
Figure 4. (A) The nucleation and growth of the grains on wetting and non-wetting surface. Adapted from Bi et al. (2015) with permission from Nature springer. (B) Device structure and energy levels of each functional layers in PSCs with PEIE interfacial modification. Reproduced from Zhou et al. (2014) with copyright from Science.
The interfaces between CTLs and perovskite layers are crucial for PSCs, but imperfect with various trap states, which gives rise to intensive research on interfacial modifications. As early as 2014, Zhou et al. demonstrated that the electron extraction process was improved by modifying the interface with polyethyleneimine ethoxylated (Zhou et al., 2014), as shown in Figure 4B. These modifications led to a maximum cell efficiency of over 19%. Furthermore, deep trap states at the interfaces need to be suppressed to reduce interfacial non-radiative recombination loss. Chen et al. proposed that interfacial chlorine avoided the formation of deep delocalized states at the TiO2/perovskite interface (Tan et al., 2017). The interfacial recombination was mitigated by the contact passivation strategy. A similar effect was also observed on alkali chloride treated NiOx/perovskite interfaces that delivered improved ordering and reduced defect density (Chen et al., 2019b).
In 2022, the perovskite-on-silicon tandem solar cell hits the world-record efficiency of 29.5% in Oxford Photovoltaics (Oxford PV), which has been certified by National Renewable Energy Laboratory (NREL) (Al-Ashouri et al., 2020). This not only refreshes the record of 28.0% previously reported by this company but also breaks the performance record of 29.2% in the Helmholtz Zentrum Berlin (HZB). Instead of replacing silicon solar cells, the perovskite-on-silicon tandem configuration provides a more accessible strategy to develop PSCs in the existing photovoltaic market, which has been considered as very promising in the industrial process of PSCs. Oxford PV that was established in 2010 from the University of Oxford, is one of the first photovoltaic companies focusing on the commercialization of perovskite based photovoltaic technology. The mainstream perovskite-on-silicon tandem solar cell technology allows breaking the previous performance limit of cell modules. The perovskite-based tandem technology has shown great potential to be compatible with the current photovoltaic industry with predicted over 30% combined cell efficiency and the ease of integration.
At the same time, photovoltaic companies in China have been devoted to the scale-up production of PSCs. Hangzhou Microquanta Co. Ltd.’s firstly established the production line of 20 MW with 200–800 cm perovskite solar cell modules and achieved 11.98% PCE, breaking the previous world record held by Toshiba. In 2019, Suzhou GCL Nano Technology Co., Ltd. (GCL Nano), a subsidiary of GCL group, built a 10 MW production line for large-area perovskite modules, and completed the material synthesis and manufacturing process. Later, GCL announced to develop the product line of 100 MW production line and planned to realize the commercial production of perovskite modules. GCL Nano announced to achieve the PCE of 15.21% on an effective area of 1,241.16 cm2. Besides, the Jayu Group and its subsidiary Jayu Solar Energy Technology Co., Ltd. also pay considerable attention to the commercialization of the third-generation perovskite photovoltaic technology, and plan a new layout in their future production bases.
With the target of carbon neutrality in 2060, it is scheduled that non-fossil energy should account for 25% in 2030, and renewable energy capacity should increase to 1.2 billion KW or more. Next decade, the photovoltaic industry should reach 70–90 million KW per year compared with 50 million per year in the past 5 years.
Perovskite materials have advantages as photovoltaics such as light absorption coefficient up 104 cm−1, long carrier diffusion length and solution processability. For example, MAPbI3 perovskites have covered a wide solar light range up to 800 nm with high light absorption coefficient. The use of FA+ cation extends the absorption edge to 840 nm, leading to remarkable photocurrent comparable to silicon solar cells (Jena et al., 2019; Min et al., 2019). The manufacturing of PSCs can be conducted through simple solution processes with low-cost earth-abundant elements such as C, H, Pb, I. It is estimated that the manufacturing cost of PSCs is only half of that of silicon solar cells (Meng et al., 2018). Compared with other commercial photovoltaic semiconductors, perovskites also exhibit higher defect tolerance (Ball and Petrozza, 2016), which delivers good reproducibility and reduces production requirements even in a module of 30 W. This merit is extremely desirable in the solution processes of films. In the large-scale integration, perovskite modules can adopt traditional rectangular photovoltaic modules that can be flexible, allowing to be intensively used on building roofs. Due to these advantages, the time horizon of PSCs is expected to be shorter than that of crystalline silicon photovoltaics.
However, there are still limitations that impede the commercialization of PSCs, for example, lead toxicity and long-term stability. Light-induced degradation of perovskites under operating conditions including moisture, oxygen, heat and current stress has become the major concern for outdoor applications of PSCs (Leijtens et al., 2015), even though crystal Si cells face similar problems when exposed to moisture and oxygen. However, the environmental impact can be resolved at the utmost by standard encapsulation technique. The future of PSCs should be bright in consideration of the history of copper indium gallium selenide (CIGS), which is first found to be unstable in the presence of water but has been widely used with long-term stability. Another instability issue is the use of organic interfacial layers such as PEDOT:PSS, Spiro-OMeTAD and so on. To solve these problems, many laboratories have been devoted to developing alternative inorganic interlayers with efficient carrier extraction. It is fortunate to see that, like the rapid development of cell efficiency, the long-term stability of PSCs has also increased a lot in the past several years. This can be attributed to the reduction in the intrinsic degradation of perovskites and the use of various CTLs with good stability. Nowadays, life-cycle assessment (LCA) of PSCs has reached more than 1,000 h (Industrial Standard for New Photovoltaic Technology), even as long as 10,000 h (Shi et al., 2020).
Another concern with perovskite-based photovoltaic technology is the toxicity of lead. Although it has been proposed that one of the main reasons for excellent optoelectronic properties in perovskite materials is the orbital overlap of Pb and I ions, the actual content of lead in PSCs is still lower than that in commercial modules. It is estimated that a perovskite cell module only contains 2 g of lead, while the value of a crystalline silicon cell module is 16 g. Furthermore, encapsulation and recycling techniques have been developed to prevent lead pollution. Obviously, the use of lead inevitably causes the risk of environmental pollution and increases the additional cost in the recycling process. Scientists have always been looking for ideal elements to replace lead in perovskite materials. Tin (Sn) is one of the potential elements to avoid the use of lead, but it is companied by the instability issue because of the oxidation of Sn2+ to Sn4+.
In the past several years, the manufacturing costs of silicon solar cells have dropped significantly that makes it possible to use photovoltaics as the large-scale clean energy supply to replace the fossil fuels. Many studies suggest that the solar energy can completely compete with conventional plants when the cost is below than 32 cents/W in well-equipped regions with sufficient solar light. However, the silicon based photovoltaic technology has approached its cost-effective limit due to thermalization loss, low absorption coefficient, and high material requirements. PSCs provides opportunities to go well beyond silicon based photovoltaic technology. Compare with silicon based photovoltaic technology that requires highly pure raw materials (99.999%), PSCs can be manufactured with relatively low purity (> 99%). More importantly, perovskite films are fabricated by low temperature solution routes that result into less power assumption and environmental pollution. Although the second-generation gallium arsenide thin-film solar cell has higher PCEs of about 30%, the cost is extremely high. It is expected that the manufacturing cost of perovskite modules would be 50% lower than that of monocrystalline silicon. However, it is difficult to displace silicon solar cells in photovoltaic market due to the lack of demonstrated production line. Alternatively, the perovskite-on-silicon tandem configuration should be one of promising technological routes in industrial progress of PSCs. The ease of tuning the bandgap of perovskites and compatible processes make them ideal wide-bandgap materials in the silicon based multi-junction solar cells, although there are some challenges in the transparent conductive layers, tunnel junctions, and light utilization (Li and Zhang, 2020). In the commercialization of PSCs, the supply chain for manufacturing is shorter, and large-scale coating equipment can be developed rapidly. The key technologies for large-area flexible perovskite solar photovoltaic cells will be achieved in about 3 years. The pathways of PSCs from the laboratory to market are emerging with numerous the efforts in academic and industrial field. The commercialization of PSCs is estimated to give rise to at least 100 billion in the photovoltaic market.
PZ organized the database and wrote the first draft of the manuscript. ML and W-CC contributed conception and design of the study and revised the whole manuscript.
This work was supported by the National Natural Science Foundation of China (No. 52003058), Guangdong Basic and Applied Basic Research Foundation (No. 2021A1515010607).
Author PZ is employed by the Beijing JAYU New Energy Technology Development Co., Ltd.
The remaining authors declare that the research was conducted in the absence of any commercial or financial relationships that could be construed as a potential conflict of interest.
All claims expressed in this article are solely those of the authors and do not necessarily represent those of their affiliated organizations, or those of the publisher, the editors and the reviewers. Any product that may be evaluated in this article, or claim that may be made by its manufacturer, is not guaranteed or endorsed by the publisher.
Abate, A., Leijtens, T., Pathak, S., Teuscher, J., Avolio, R., Errico, M. E., et al. (2013). Lithium Salts as “redox Active” P-type Dopants for Organic Semiconductors and Their Impact in Solid-State Dye-Sensitized Solar Cells. Phys. Chem. Chem. Phys. 15 (7), 2572–2579. doi:10.1039/C2CP44397J
Al-Ashouri, A., Köhnen, E., Li, B., Magomedov, A., Hempel, H., Caprioglio, P., et al. (2020). Monolithic Perovskite/Silicon Tandem Solar Cell with >29% Efficiency by Enhanced Hole Extraction. Science 370 (6522), 1300–1309. doi:10.1126/science.abd4016
Ball, J. M., and Petrozza, A. (2016). Defects in Perovskite-Halides and Their Effects in Solar Cells. Nat. Energ. 1 (11), 16149. doi:10.1038/nenergy.2016.149
Bi, C., Wang, Q., Shao, Y., Yuan, Y., Xiao, Z., and Huang, J. (2015). Non-Wetting Surface-Driven High-Aspect-Ratio Crystalline Grain Growth for Efficient Hybrid Perovskite Solar Cells. Nat. Commun. 6 (1), 7747. doi:10.1038/ncomms8747
Bi, D., Yi, C., Luo, J., Décoppet, J.-D., Zhang, F., Zakeeruddin, S. M., et al. (2016). Polymer-Templated Nucleation and Crystal Growth of Perovskite Films for Solar Cells with Efficiency Greater Than 21%. Nat. Energ. 1 (10), 16142. doi:10.1038/nenergy.2016.142
Burschka, J., Pellet, N., Moon, S.-J., Humphry-Baker, R., Gao, P., Nazeeruddin, M. K., et al. (2013). Sequential Deposition as a Route to High-Performance Perovskite-Sensitized Solar Cells. Nature 499 (7458), 316–319. doi:10.1038/nature12340
Cao, Y., Wang, N., Tian, H., Guo, J., Wei, Y., Chen, H., et al. (2018). Perovskite Light-Emitting Diodes Based on Spontaneously Formed Submicrometre-Scale Structures. Nature 562 (7726), 249–253. doi:10.1038/s41586-018-0576-2
Chakhmouradian, A. R., and Woodward, P. M. (2014). Celebrating 175 Years of Perovskite Research: A Tribute to Roger H. Mitchell. Phys. Chem. Minerals 41 (6), 387–391. doi:10.1007/s00269-014-0678-9
Chen, H. (2017). Two-Step Sequential Deposition of Organometal Halide Perovskite for Photovoltaic Application. Adv. Funct. Mater. 27 (8), 1605654. doi:10.1002/adfm.201605654
Chen, Q., Zhou, H., Hong, Z., Luo, S., Duan, H.-S., Wang, H.-H., et al. (2014). Planar Heterojunction Perovskite Solar Cells via Vapor-Assisted Solution Process. J. Am. Chem. Soc. 136 (2), 622–625. doi:10.1021/ja411509g
Chen, W., Li, D., Chen, X., Chen, H., Liu, S., Yang, H., et al. (2021). Surface Reconstruction for Stable Monolithic All-Inorganic Perovskite/Organic Tandem Solar Cells with over 21% Efficiency. Adv. Funct. Mater. 32 (5), 2109321. doi:10.1002/adfm.202109321
Chen, W., Wang, Y., Pang, G., Koh, C. W., Djurišić, A. B., Wu, Y., et al. (2019a). Conjugated Polymer-Assisted Grain Boundary Passivation for Efficient Inverted Planar Perovskite Solar Cells. Adv. Funct. Mater. 29 (27), 1808855. doi:10.1002/adfm.201808855
Chen, W., Zhou, Y., Chen, G., Wu, Y., Tu, B., Liu, F. Z., et al. (2019b). Alkali Chlorides for the Suppression of the Interfacial Recombination in Inverted Planar Perovskite Solar Cells. Adv. Energ. Mater. 9 (19), 1803872. doi:10.1002/aenm.201803872
Chen, W., Zhu, Y., Xiu, J., Chen, G., Liang, H., Liu, S., et al. (2022). Monolithic Perovskite/Organic Tandem Solar Cells with 23.6% Efficiency Enabled by Reduced Voltage Losses and Optimized Interconnecting Layer. Nat. Energ. doi:10.1038/s41560-021-00966-8
Chen, Y., Yang, Z., Wang, S., Zheng, X., Wu, Y., Yuan, N., et al. (2018). Design of an Inorganic Mesoporous Hole-Transporting Layer for Highly Efficient and Stable Inverted Perovskite Solar Cells. Adv. Mater. 30 (52), 1805660. doi:10.1002/adma.201805660
Cheng, Y., Yang, Q.-D., Xiao, J., Xue, Q., Li, H.-W., Guan, Z., et al. (2015). Decomposition of Organometal Halide Perovskite Films on Zinc Oxide Nanoparticles. ACS Appl. Mater. Inter. 7 (36), 19986–19993. doi:10.1021/acsami.5b04695
Chiang, C.-H., Nazeeruddin, M. K., Grätzel, M., and Wu, C.-G. (2017). The Synergistic Effect of H2O and DMF towards Stable and 20% Efficiency Inverted Perovskite Solar Cells. Energy Environ. Sci. 10 (3), 808–817. doi:10.1039/C6EE03586H
Chiang, Y.-H., Anaya, M., and Stranks, S. D. (2020). Multisource Vacuum Deposition of Methylammonium-Free Perovskite Solar Cells. ACS Energ. Lett. 5 (8), 2498–2504. doi:10.1021/acsenergylett.0c00839
Cho, H., Jeong, S.-H., Park, M.-H., Kim, Y.-H., Wolf, C., Lee, C.-L., et al. (2015). Overcoming the Electroluminescence Efficiency Limitations of Perovskite Light-Emitting Diodes. Science 350 (6265), 1222–1225. doi:10.1126/science.aad1818
Dong, B., Hu, J., Xiao, X., Tang, S., Gao, X., Peng, Z., et al. (2019). High-Efficiency Fiber-Shaped Perovskite Solar Cell by Vapor-Assisted Deposition with a Record Efficiency of 10.79%. Adv. Mater. Tech. 4 (7), 1900131. doi:10.1002/admt.201900131
Dou, B., Whitaker, J. B., Bruening, K., Moore, D. T., Wheeler, L. M., Ryter, J., et al. (2018). Roll-to-Roll Printing of Perovskite Solar Cells. ACS Energ. Lett. 3 (10), 2558–2565. doi:10.1021/acsenergylett.8b01556
Eperon, G. E., Stranks, S. D., Menelaou, C., Johnston, M. B., Herz, L. M., and Snaith, H. J. (2014). Formamidinium lead Trihalide: A Broadly Tunable Perovskite for Efficient Planar Heterojunction Solar Cells. Energ. Environ. Sci. 7 (3), 982–988. doi:10.1039/C3EE43822H
Fang, F., Yuan, Y., Wan, Y., Li, J., Song, Y., Chen, W. C., et al. (2022). Near-Infrared Thermally Activated Delayed Fluorescence Nanoparticle: A Metal-Free Photosensitizer for Two-Photon-Activated Photodynamic Therapy at the Cell and Small Animal Levels. Small 18 (6), 2106215. doi:10.1002/smll.202106215
Fang, F., Zhu, L., Li, M., Song, Y., Sun, M., Zhao, D., et al. (2021). Thermally Activated Delayed Fluorescence Material: An Emerging Class of Metal-Free Luminophores for Biomedical Applications. Adv. Sci. 8 (24), 2102970. doi:10.1002/advs.202102970
Feng, J., Jiao, Y., Wang, H., Zhu, X., Sun, Y., Du, M., et al. (2021). High-Throughput Large-Area Vacuum Deposition for High-Performance Formamidine-Based Perovskite Solar Cells. Energ. Environ. Sci. 14 (5), 3035–3043. doi:10.1039/D1EE00634G
Galagan, Y., Di Giacomo, F., Gorter, H., Kirchner, G., de Vries, I., Andriessen, R., et al. (2018). Roll-to-Roll Slot Die Coated Perovskite for Efficient Flexible Solar Cells. Adv. Energ. Mater. 8 (32), 1801935. doi:10.1002/aenm.201801935
Gao, Y., Yang, L., Wang, F., Sui, Y., Sun, Y., Wei, M., et al. (2018). Anti-Solvent Surface Engineering via Diethyl Ether to Enhance the Photovoltaic Conversion Efficiency of Perovskite Solar Cells to 18.76%. Superlattices Microstruct 113, 761–768. doi:10.1016/j.spmi.2017.12.015
George, J. J. K., Halali, V. V., C. G., S., Suvina, V., Sakar, M., and Balakrishna, R. G. (2020). Perovskite Nanomaterials as Optical and Electrochemical Sensors. Inorg. Chem. Front. 7 (14), 2702–2725. doi:10.1039/D0QI00306A
Green, M. A., Ho-Baillie, A., and Snaith, H. J. (2014). The Emergence of Perovskite Solar Cells. Nat. Photonics 8, 506. doi:10.1038/nphoton.2014.134
Hwang, K., Jung, Y.-S., Heo, Y.-J., Scholes, F. H., Watkins, S. E., Subbiah, J., et al. (2015). Toward Large Scale Roll-To-Roll Production of Fully Printed Perovskite Solar Cells. Adv. Mater. 27 (7), 1241–1247. doi:10.1002/adma.201404598
Igual-Muñoz, A. M., Ávila, J., Boix, P. P., and Bolink, H. J. (2020). FAPb0.5Sn0.5I3: A Narrow Bandgap Perovskite Synthesized through Evaporation Methods for Solar Cell Applications. Sol. RRL 4 (2), 2070024. doi:10.1002/solr.202070024
Im, J.-H., Kim, H.-S., and Park, N.-G. (2014). Morphology-Photovoltaic Property Correlation in Perovskite Solar Cells: One-Step versus Two-Step Deposition of CH3NH3PbI3. APL Mater. 2 (8), 081510. doi:10.1063/1.4891275
Jena, A. K., Kulkarni, A., and Miyasaka, T. (2019). Halide Perovskite Photovoltaics: Background, Status, and Future Prospects. Chem. Rev. 119 (5), 3036–3103. doi:10.1021/acs.chemrev.8b00539
Jeng, J.-Y., Chiang, Y.-F., Lee, M.-H., Peng, S.-R., Guo, T.-F., Chen, P., et al. (2013). CH3NH3PbI3Perovskite/Fullerene Planar-Heterojunction Hybrid Solar Cells. Adv. Mater. 25 (27), 3727–3732. doi:10.1002/adma.201301327
Jeon, N. J., Noh, J. H., Kim, Y. C., Yang, W. S., Ryu, S., and Seok, S. I. (2014). Solvent Engineering for High-Performance Inorganic-Organic Hybrid Perovskite Solar Cells. Nat. Mater 13 (9), 897–903. doi:10.1038/nmat4014
Jeon, N. J., Noh, J. H., Yang, W. S., Kim, Y. C., Ryu, S., Seo, J., et al. (2015). Compositional Engineering of Perovskite Materials for High-Performance Solar Cells. Nature 517 (7535), 476–480. doi:10.1038/nature14133
Jeong, J., Kim, M., Seo, J., Lu, H., Ahlawat, P., Mishra, A., et al. (2021). Pseudo-Halide Anion Engineering for α-FAPbI3 Perovskite Solar Cells. Nature 592 (7854), 381–385. doi:10.1038/s41586-021-03406-5
Jiang, F., Choy, W. C. H., Li, X., Zhang, D., and Cheng, J. (2015). Post-Treatment-Free Solution-Processed Non-Stoichiometric NiOx Nanoparticles for Efficient Hole-Transport Layers of Organic Optoelectronic Devices. Adv. Mater. 27 (18), 2930–2937. doi:10.1002/adma.201405391
Jiang, Q., Zhang, L., Wang, H., Yang, X., Meng, J., Liu, H., et al. (2016). Enhanced Electron Extraction Using SnO2 for High-Efficiency Planar-Structure HC(NH2)2PbI3-based Perovskite Solar Cells. Nat. Energ. 2 (1), 16177. doi:10.1038/nenergy.2016.177
Jiang, Q., Zhang, X., and You, J. (2018). SnO2: A Wonderful Electron Transport Layer for Perovskite Solar Cells. Small 14 (31), 1801154. doi:10.1002/smll.201801154
Kim, H. S., Lee, C. R., Im, J. H., Lee, K. B., Moehl, T., Marchioro, A., et al. (2012). Lead Iodide Perovskite Sensitized All-Solid-State Submicron Thin Film Mesoscopic Solar Cell with Efficiency Exceeding 9%. Sci. Rep. 2 (1), 591. doi:10.1038/srep00591
Kim, J. E., Kim, S. S., Zuo, C., Gao, M., Vak, D., and Kim, D. Y. (2019a). Humidity-Tolerant Roll-to-Roll Fabrication of Perovskite Solar Cells via Polymer-Additive-Assisted Hot Slot Die Deposition. Adv. Funct. Mater. 29 (26), 1809194. doi:10.1002/adfm.201809194
Kim, J. H., Liang, P.-W., Williams, S. T., Cho, N., Chueh, C.-C., Glaz, M. S., et al. (2015). High-Performance and Environmentally Stable Planar Heterojunction Perovskite Solar Cells Based on a Solution-Processed Copper-Doped Nickel Oxide Hole-Transporting Layer. Adv. Mater. 27 (4), 695–701. doi:10.1002/adma.201404189
Kim, Y. Y., Yang, T.-Y., Suhonen, R., Kemppainen, A., Hwang, K., Jeon, N. J., et al. (2020). Roll-to-roll Gravure-Printed Flexible Perovskite Solar Cells Using Eco-Friendly Antisolvent Bathing with Wide Processing Window. Nat. Commun. 11 (1), 5146. doi:10.1038/s41467-020-18940-5
Kim, Y. Y., Yang, T. Y., Suhonen, R., Välimäki, M., Maaninen, T., Kemppainen, A., et al. (2019b). Gravure-Printed Flexible Perovskite Solar Cells: Toward Roll-to-Roll Manufacturing. Adv. Sci. 6 (7), 1802094. doi:10.1002/advs.201802094
Kojima, A., Teshima, K., Shirai, Y., and Miyasaka, T. (2009). Organometal Halide Perovskites as Visible-Light Sensitizers for Photovoltaic Cells. J. Am. Chem. Soc. 131 (17), 6050–6051. doi:10.1021/ja809598r
Lee, M. M., Teuscher, J., Miyasaka, T., Murakami, T. N., and Snaith, H. J. (2012). Efficient Hybrid Solar Cells Based on Meso-Superstructured Organometal Halide Perovskites. Science 338 (6107), 643–647. doi:10.1126/science.1228604
Leijtens, T., Eperon, G. E., Noel, N. K., Habisreutinger, S. N., Petrozza, A., and Snaith, H. J. (2015). Stability of Metal Halide Perovskite Solar Cells. Adv. Energ. Mater 5 (20), 1500963. doi:10.1002/aenm.201500963
Leijtens, T., Eperon, G. E., Pathak, S., Abate, A., Lee, M. M., and Snaith, H. J. (2013). Overcoming Ultraviolet Light Instability of Sensitized TiO2 with Meso-Superstructured Organometal Tri-halide Perovskite Solar Cells. Nat. Commun. 4 (1), 2885. doi:10.1038/ncomms3885
Li, C., Lu, X., Ding, W., Feng, L., Gao, Y., and Guo, Z. (2008). Formability of ABX 3 (X = F, Cl, Br, I) Halide Perovskites. Acta Crystallogr. Sect B 64 (6), 702–707. doi:10.1107/s0108768108032734
Li, C., Ma, Y., Xiao, Y., Shen, L., and Ding, L. (2020a). Advances in Perovskite Photodetectors. InfoMat 2 (6), 1247–1256. doi:10.1002/inf2.12141
Li, C., Wang, H., Wang, F., Li, T., Xu, M., Wang, H., et al. (2020b). Ultrafast and Broadband Photodetectors Based on a Perovskite/organic Bulk Heterojunction for Large-Dynamic-Range Imaging. Light Sci. Appl. 9 (1), 31. doi:10.1038/s41377-020-0264-5
Li, H., and Zhang, W. (2020). Perovskite Tandem Solar Cells: From Fundamentals to Commercial Deployment. Chem. Rev. 120 (18), 9835–9950. doi:10.1021/acs.chemrev.9b00780
Li, J., Wang, H., Chin, X. Y., Dewi, H. A., Vergeer, K., Goh, T. W., et al. (2020c). Highly Efficient Thermally Co-Evaporated Perovskite Solar Cells and Mini-Modules. Joule 4 (5), 1035–1053. doi:10.1016/j.joule.2020.03.005
Li, Y., Wang, J., Yuan, Y., Dong, X., and Wang, P. (2017). Anti-Solvent Dependent Device Performance in CH3NH3PbI3 Solar Cells: the Role of Intermediate Phase Content in the As-Prepared Thin Films. Sustain. Energ. Fuels 1 (5), 1041–1048. doi:10.1039/C7SE00125H
Lin, K., Xing, J., Quan, L. N., de Arquer, F. P. G., Gong, X., Lu, J., et al. (2018). Perovskite Light-Emitting Diodes with External Quantum Efficiency Exceeding 20 Per Cent. Nature 562 (7726), 245–248. doi:10.1038/s41586-018-0575-3
Lin, R., Xu, J., Wei, M., Wang, Y., Qin, Z., Liu, Z., et al. (2022). All-perovskite Tandem Solar Cells with Improved Grain Surface Passivation. Nature. doi:10.1038/s41586-021-04372-8
Liu, D., and Kelly, T. L. (2014). Perovskite Solar Cells with a Planar Heterojunction Structure Prepared Using Room-Temperature Solution Processing Techniques. Nat. Photon 8 (2), 133–138. doi:10.1038/nphoton.2013.342
Liu, M., Johnston, M. B., and Snaith, H. J. (2013). Efficient Planar Heterojunction Perovskite Solar Cells by Vapour Deposition. Nature 501 (7467), 395–398. doi:10.1038/nature12509
Longo, G., Momblona, C., La-Placa, M.-G., Gil-Escrig, L., Sessolo, M., and Bolink, H. J. (2018). Fully Vacuum-Processed Wide Band Gap Mixed-Halide Perovskite Solar Cells. ACS Energ. Lett. 3 (1), 214–219. doi:10.1021/acsenergylett.7b01217
Meng, L., You, J., and Yang, Y. (2018). Addressing the Stability Issue of Perovskite Solar Cells for Commercial Applications. Nat. Commun. 9 (1), 5265. doi:10.1038/s41467-018-07255-1
Min, H., Kim, M., Lee, S.-U., Kim, H., Kim, G., Choi, K., et al. (2019). Efficient, Stable Solar Cells by Using Inherent Bandgap of α-phase Formamidinium lead Iodide. Science 366 (6466), 749–753. doi:10.1126/science.aay7044
Mitzi, D. B., Prikas, M. T., and Chondroudis, K. (1999). Thin Film Deposition of Organic−Inorganic Hybrid Materials Using a Single Source Thermal Ablation Technique. Chem. Mater. 11 (3), 542–544. doi:10.1021/cm9811139
Noh, J. H., Im, S. H., Heo, J. H., Mandal, T. N., and Seok, S. I. (2013). Chemical Management for Colorful, Efficient, and Stable Inorganic-Organic Hybrid Nanostructured Solar Cells. Nano Lett. 13 (4), 1764–1769. doi:10.1021/nl400349b
NREL (2020). Best Research-Cell Efficiency Chart [Online]. NREL. Available: https://www.nrel.gov/pv/cell-efficiency.html (Accessed April 19, 2021).
Ouyang, D., Huang, Z., and Choy, W. C. H. (2019). Solution-Processed Metal Oxide Nanocrystals as Carrier Transport Layers in Organic and Perovskite Solar Cells. Adv. Funct. Mater. 29 (1), 1804660. doi:10.1002/adfm.201804660
Ouyang, D., Xiao, J. Y., Ye, F., Huang, Z. F., Zhang, H., Zhu, L., et al. (2018). Strategic Synthesis of Ultrasmall NiCo2O4 NPs as Hole Transport Layer for Highly Efficient Perovskite Solar Cells. Adv. Energy Mater 8 (16), 1702722. doi:10.1002/aenm.201702722
Qin, P.-L., Yang, G., Ren, Z.-W., Cheung, S. H., So, S. K., Chen, L., et al. (2018). Stable and Efficient Organo-Metal Halide Hybrid Perovskite Solar Cells via π-Conjugated Lewis Base Polymer Induced Trap Passivation and Charge Extraction. Adv. Mater. 30 (12), 1706126. doi:10.1002/adma.201706126
Qin, S., Lu, C., Jia, Z., Wang, Y., Li, S., Lai, W., et al. (2022). Constructing Monolithic Perovskite/Organic Tandem Solar Cell with Efficiency of 22.0% via Reduced Open-Circuit Voltage Loss and Broadened Absorption Spectra. Adv. Mater., 2108829. doi:10.1002/adma.202108829
Rajagopal, A., Liang, P.-W., Chueh, C.-C., Yang, Z., and Jen, A. K.-Y. (2017). Defect Passivation via a Graded Fullerene Heterojunction in Low-Bandgap Pb-Sn Binary Perovskite Photovoltaics. ACS Energ. Lett. 2 (11), 2531–2539. doi:10.1021/acsenergylett.7b00847
Saliba, M., Matsui, T., Seo, J.-Y., Domanski, K., Correa-Baena, J.-P., Nazeeruddin, M. K., et al. (2016). Cesium-containing Triple Cation Perovskite Solar Cells: Improved Stability, Reproducibility and High Efficiency. Energ. Environ. Sci. 9 (6), 1989–1997. doi:10.1039/C5EE03874J
Shi, L., Bucknall, M. P., Young, T. L., Zhang, M., Hu, L., Bing, J., et al. (2020). Gas Chromatography -mass Spectrometry Analyses of Encapsulated Stable Perovskite Solar Cells. Science 368 (6497), eaba2412. doi:10.1126/science.aba2412
Shin, S. S., Yang, W. S., Noh, J. H., Suk, J. H., Jeon, N. J., Park, J. H., et al. (2015). High-performance Flexible Perovskite Solar Cells Exploiting Zn2SnO4 Prepared in Solution below 100 °C. Nat. Commun. 6 (1), 7410. doi:10.1038/ncomms8410
Stranks, S. D., Eperon, G. E., Grancini, G., Menelaou, C., Alcocer, M. J. P., Leijtens, T., et al. (2013). Electron-Hole Diffusion Lengths Exceeding 1 Micrometer in an Organometal Trihalide Perovskite Absorber. Science 342 (6156), 341–344. doi:10.1126/science.1243982
Sutherland, B. R., and Sargent, E. H. (2016). Perovskite Photonic Sources. Nat. Photon 10 (5), 295–302. doi:10.1038/nphoton.2016.62
Tan, H., Jain, A., Voznyy, O., Lan, X., García de Arquer, F. P., Fan, J. Z., et al. (2017). Efficient and Stable Solution-Processed Planar Perovskite Solar Cells via Contact Passivation. Science 355 (6326), 722–726. doi:10.1126/science.aai9081
Wei, Q., Li, X., Liang, C., Zhang, Z., Guo, J., Hong, G., et al. (2019). Recent Progress in Metal Halide Perovskite Micro- and Nanolasers. Adv. Opt. Mater. 7 (17), 1900080. doi:10.1002/adom.201900080
Wu, T., Wang, Y., Li, X., Wu, Y., Meng, X., Cui, D., et al. (2019). Efficient Defect Passivation for Perovskite Solar Cells by Controlling the Electron Density Distribution of Donor-π-Acceptor Molecules. Adv. Energ. Mater. 9 (17), 1803766. doi:10.1002/aenm.201803766
Wu, Y., Yang, X., Chen, W., Yue, Y., Cai, M., Xie, F., et al. (2016). Perovskite Solar Cells with 18.21% Efficiency and Area over 1 cm2 Fabricated by Heterojunction Engineering. Nat. Energ. 1 (11), 16148. doi:10.1038/nenergy.2016.148
Xing, G., Mathews, N., Sun, S., Lim, S. S., Lam, Y. M., Grätzel, M., et al. (2013). Long-Range Balanced Electron- and Hole-Transport Lengths in Organic-Inorganic CH 3 NH 3 PbI 3. Science 342 (6156), 344–347. doi:10.1126/science.1243167
Xu, X., Li, M., Xie, Y.-M., Ma, Y., Ma, C., Cheng, Y., et al. (2019). Porous and Intercrossed PbI2-CsI Nanorod Scaffold for Inverted Planar FA-Cs Mixed-Cation Perovskite Solar Cells. ACS Appl. Mater. Inter. 11 (6), 6126–6135. doi:10.1021/acsami.8b20933
Yang, G., Qin, P., Fang, G., and Li, G. (2018). A Lewis Base-Assisted Passivation Strategy towards Highly Efficient and Stable Perovskite Solar Cells. Sol. RRL 2 (8), 1800055. doi:10.1002/solr.201800055
Yang, T.-Y., Kim, Y. Y., and Seo, J. (2021). Roll-to-roll Manufacturing toward Lab-To-Fab-Translation of Perovskite Solar Cells. APL Mater. 9 (11), 110901. doi:10.1063/5.0064073
You, J., Meng, L., Song, T.-B., Guo, T.-F., Yang, Y., Chang, W.-H., et al. (2016). Improved Air Stability of Perovskite Solar Cells via Solution-Processed Metal Oxide Transport Layers. Nat. Nanotech 11 (1), 75–81. doi:10.1038/nnano.2015.230
Zaza, F., Pallozzi, V., and Serra, E. (2019). Optimization of Working Conditions for Perovskite-Based Gas Sensor Devices by Multiregression Analysis. J. Nanotechnol 2019, 4628765. doi:10.1155/2019/4628765
Zhang, H., Cheng, J., Lin, F., He, H., Mao, J., Wong, K. S., et al. (2016). Pinhole-Free and Surface-Nanostructured NiOx Film by Room-Temperature Solution Process for High-Performance Flexible Perovskite Solar Cells with Good Stability and Reproducibility. ACS Nano 10 (1), 1503–1511. doi:10.1021/acsnano.5b07043
Zhang, H., Mao, J., He, H., Zhang, D., Zhu, H. L., Xie, F., et al. (2015). A Smooth CH3NH3PbI3Film via a New Approach for Forming the PbI2Nanostructure Together with Strategically High CH3NH3I Concentration for High Efficient Planar-Heterojunction Solar Cells. Adv. Energ. Mater. 5 (23), 1501354. doi:10.1002/aenm.201501354
Zhang, Y., Gao, X., Wu, Y., Gui, J., Guo, S., Zheng, H., et al. (2021). Self-powered Technology Based on Nanogenerators for Biomedical Applications. Exploration 1 (1), 90–114. doi:10.1002/EXP.10.1002/exp.20210152
Keywords: perovskite, solar cells, composition engineering, process engineering, interfacial engineering, industrial progress, commercialization
Citation: Zhang P, Li M and Chen W-C (2022) A Perspective on Perovskite Solar Cells: Emergence, Progress, and Commercialization. Front. Chem. 10:802890. doi: 10.3389/fchem.2022.802890
Received: 27 October 2021; Accepted: 17 February 2022;
Published: 11 April 2022.
Edited by:
Wenwu Qin, Northeast Normal University, ChinaReviewed by:
Miaoqiang Lyu, The University of Queensland, AustraliaCopyright © 2022 Zhang, Li and Chen. This is an open-access article distributed under the terms of the Creative Commons Attribution License (CC BY). The use, distribution or reproduction in other forums is permitted, provided the original author(s) and the copyright owner(s) are credited and that the original publication in this journal is cited, in accordance with accepted academic practice. No use, distribution or reproduction is permitted which does not comply with these terms.
*Correspondence: Menglin Li, bWVuZ2xpbmxpOTBAYml0LmVkdS5jbg==; Wen-Cheng Chen, d2VuY2NoZW5AZ2R1dC5lZHUuY24=
Disclaimer: All claims expressed in this article are solely those of the authors and do not necessarily represent those of their affiliated organizations, or those of the publisher, the editors and the reviewers. Any product that may be evaluated in this article or claim that may be made by its manufacturer is not guaranteed or endorsed by the publisher.
Research integrity at Frontiers
Learn more about the work of our research integrity team to safeguard the quality of each article we publish.