- Shanghai Key Laboratory of Materials Protection and Advanced Materials in Electric Power, Shanghai University of Electric Power, Shanghai, China
We report a facile and effective inorganic polycondensation combined with aerosol-spray strategy towards high-performance photocatalyst by fabricating mesoporous Ti1-xSnxO2 (0 < x < 1) solid solution. Such Ti1-xSnxO2 nanocrystals with high Sn-doped contents are self-assembled into mesoporous spheres can effectively promote visible-light harvest and high quantum yield, leading a longer lifetime of the photoelectron-hole pairs and less recombination. Such the photocatalysts enhanced photocatalytic activity for the degradation of Rhodamine B (RhB). The representative Ti0.9Sn0.1O2 and Ti0.8Sn0.2O2 compounds reach an optimum degradation of ≈50% and 70%, respectively, after 120 min irradiation under visible irradiation. The mesoporous Ti1-xSnxO2 solid solution could inhibit the recombination of electron-hole pairs, which promote reaction thermodynamics and kinetics for RhB degradation.
1 Introduction
Rapid development and the transition to a carbon-neutral society have highlighted the need for photovoltaic cells to use high-efficiency photocatalysts under visible light (>380 nm) (Liu et al., 2016; Galvao et al., 2018; González-Gutiérrez et al., 2019). Transition metal oxides (such as TiO2 and SnO2) have attracted considerable attention from researchers due to their excellent photocatalytic activity, high natural abundance, and cost-effectiveness (Sharon et al., 2016). Owing to two oxides with different band-gap energies (Eg) of 3.2 eV for TiO2 and 3.8 eV for SnO2 (Nuansing et al., 2006), respectively, the enhanced charge separation occurs in their mixture where electrons are injected from the conduction band of SnO2 to the valence band of TiO2, leading to better performance than individual semiconductor oxides due to their synergistic effects. To better regulate their band gap and obtain high efficiency, more efforts should be focused on building a desired heterostructure and tuning electron correlation by varying the stoichiometry of oxides Luo et al. (2018). Heterostructures were mainly fabricated by the assembly of two or more oxides that were physically (e.g., Van der Wall’s force) or chemically bonded together, exhibiting superiority of geometry and junction interface (Li et al., 2021; Liu et al., 2023). For heterostructure-based metal oxide, element doping coupled with hierarchy design achieved visible benefits due to the formation of a single phase rather than a combination of two oxide compounds. For example, the pre-synthesized SnO2 quantum dots directed growth on titania show slow charge transfer, low active-sensitizer loading and poor uniformity control for deposition on high aspect-ratio TiO2 nanostructures (Wang et al., 2022). Doping titania with either inorganic or metallic species can generate donor or acceptor states in the band gap of TiO2 and impurity states that enhance visible-light adsorption (Albornoz et al., 2021; Wu et al., 2022). Non-etheless, metal oxides with doping heterostructure often suffered from thermal instability, fast recombination rates, or the requirement of an expensive ion-implantation facility for more elaborate control, and even showed much larger formation energy due to the substitution of large-radius metal ions (Singh et al., 2021; Liu et al., 2022). Many essential questions remain, such as the effect of the structure-function correction, composition, and interfaces on the photocatalytic activity, which remains challenging.
One promising strategy to obtain a heterostructure-based titania photocatalyst is to fabricate a substitutional solid solution using metal atoms with a comparable ionic radius (VI-coordinated) with titania (Reddy et al., 2008). Such a metal-doped TiO2 solid not only retains the benefits from the quantum structures of crystalline titania during photocatalytic processes but further enhances photoinduced surface redox reactions one. Compared with the other cationic doping (such as Cr, V, and Fe), Sn ion shows a more favorable effect on photocatalytic activity in water-splitting and oxidation of organic compounds (Liang et al., 2012). To date, few examples of tin-doping titania (denoted as Ti1-xSnxO2, 0 < x < 1) have been reported, especially on nanoscale systems (Asokan et al., 2010). Such compounds have been generally synthesized by solid-state reaction at high temperatures beyond 1,200°C or chemical deposition technology, such as hydrothermal (Chen et al., 2022; Zhao et al., 2016; Ruiz Esquius et al., 2020), sol-gel (Pudukudy et al., 2017; Liu et al., 2019), electrospinning (Yu et al., 2018), and polyol-mediated methods (Pelicano et al., 2022). However, these methods are limited because of the difficulty in elaborate control of doping contents and nanoparticle aggregation and the incapability of constructing hierarchical pore structures, leading to an increased probability of recombination. Besides, most compounds prepared using the above-mentioned methods have a rutile tetragonal crystalline structure with no anatase analog due to the existence of Sn (Qin et al., 2021). The latter has been proposed to have higher photoactivity than the former, but it is still limited by a less Sn-doping content (<0.3).
To address this challenge, we report a facile and effective doping method to prepare a hierarchically mesoporous Ti1-xSnxO2 compound for high-performance photocatalyst under visible light irradiation. Inspired by the Ziegler-Natta catalyst mechanism, Figure 1 illustrates an inorganic polycondensation process that occurs between titanium chloride and tin chloride liquid in a liquid nitrogen bath. The ultra-low temperature effectively suppresses chloride salt hydrolysis and causes chloride ions from tin chloride to fill the empty orbital of a 6-coordinated Ti atom. Then, Ti atoms and Sn atoms were bridged to two chlorine atoms to obtain successive -Ti-Cl-Sn-Cl-Ti- chains, allowing for high Sn-contents. Subsequently, the mesoporous Ti1-xSnxO2 spheres are synthesized using a low-cost aerosol-spray method. Ti1-xSnxO2 nanocrystals generated from such a preformed aqueous solution are self-assembled into mesoporous spheres. Such a hierarchical heterostructure endows several advantages to the Ti1-xSnxO2 compound. Firstly, mesoporous Ti1-xSnxO2 spheres inherit the anatase crystalline structure from titania and small nanocrystal size, which achieves a high surface area and many active sites. Second, hierarchically porous architecture coupled with high Sn-contents can effectively promote visible-light harvest and high quantum yield. Finally, the mesoporous structure throughout the spheres endows effective transport of charge carriers, allowing for longer lifetimes of the photoelectron-hole pairs and less recombination. Such a strategy could be easily extended to other photocatalyst designs and large-scale fabrication.
2 Experiment section
2.1 Materials preparation
Pluronic surfactant F127 (EO106PO70EO106), titanium chloride (TiCl4; ≥98%), and tin (IV) chloride (SnCl4, ≥99%) were purchased from Sigma-Aldrich Corp. All chemicals were used without further purification. The Ti1-xSnxO2 (0 < x < 1) solid solutions and the undoped TiO2, SnO2 were all synthesized by an aerosol-spraying process using TiCl4, SnCl4 and tri-block copolymer F127 aqueous solution as a precursor. Such a stable and homogeneous precursor was achieved by a polycondensation process using various mole ratios (Ti: Sn = 1-x: x (0 < x < 1) under liquid nitrogen for 2 h and then slowly injecting 100 ml of F127 (0.5 g) aqueous solution. The mole fraction of Sn-containing precursor in the mixture is denoted by x = mmol Sn/(mmol Ti + mmol Sn). The mixture solution was vigorously stirred for 30 min and subsequently used an aerosol-assisted self-assembly process to continuously generate droplets from the precursors. The droplets were then sent through a furnace and reacted at 450°C to form homogenous particles. Calcination was carried out in a box furnace at 350°C for 3 h and then 550°C for another 3 h under the air atmosphere. The heating rate for the whole process was kept at 2°C·min−1. As-made products were milled and grounded into fine powder.
2.2 Material characterization and measurement
X-ray diffraction (XRD) patterns were taken on a Panalytical X'Pert Pro X-ray powder diffractometer powders diffractometer using copper Kα radiation (λ = 1.54 Å). Nitrogen sorption isotherms were measured at 77 K with a Micromeritics ASAP 2020 analyzer. The samples were degassed in vacuum at 180°C for 3 h before measurements were taken. The specific surface areas were calculated by the Brunauer–Emmett–Teller (BET) method using the adsorption branch in the relative pressure range from 0.04 to 0.25. The pore size distributions (Dp) were derived from the adsorption branch using the Barrett–Joyner–Halenda (BJH) model. Scanning electron microscopy (SEM) images and energy-dispersive spectroscopy (EDX) were obtained using a JEOL JSM-6700 FE-SEM at 200 kV to obtain morphology and elemental information of the products. Transmission electron microscopy (TEM) images were obtained using a Philips CM120 microscope operated at 120 kV. Surface composition was analyzed by X-ray photoelectron spectroscopy (XPS). Core level photoemission spectra of C 1 s and O 1 s lines were collected with a PHI 3057 spectrometer using Mg Kα X-rays at 1,286.6 eV. All XPS spectra were taken in small area mode with an acceptance angle of 78° and 23.5 eV pass energy. All spectra were referenced to the C 1 s peak of the graphitic carbon atom at 285.0 eV. The integrated areas of the Ti 2p and Sn 3 days photoemission peaks, divided by their sensitivity factors 0.665/1.334 and 3.286/4.725, were used to determine surface atomic percentages. UV-vis absorption spectra were recorded on a Shimadzu UV-1700 spectrometer with a resolution of 2.0 nm.
2.3 Photocatalytic activity measurement
Under visible light irradiation, the photocatalytic activities of the Ti1-xSnxO2 (0 < x < 1) solutions were evaluated by the photodegradation of organic contaminants, and Rhodamine B (RhB, Mw: 479.02) was used as the model pollutant. A set of photocatalytic activity experiments on samples were performed with the following procedures: 0.1 g of photocatalyst was put into 30 ml of DI-water and stirred at room temperature for 1 h to make the photocatalyst powder uniformly dispersed. Then RhB was added into the suspension to keep a concentration of 10−5 mol·L−1. Then the mixture was stirred in the dark for 30 min to reach adsorption–desorption equilibrium. From the top, the suspension was vertically irradiated with simulated visible light from a 500 W Xe lamp equipped with a UV cutoff filter (>400 nm) with distance of 15 cm between the lamp and the suspension surface. During the photo reaction, the samples were collected at different time intervals; 0 min, 10 min, 30 min, 60 min, 90 min, and 120 min for analysis. In order to estimate the photocatalytic stability, a cycle photocatalytic degradation experiment was conducted as follows: the original concentration of RhB dye in the reactor was tuned to 10−5 mol·L−1 every 120 min as one cycle, and then the samples were picked up at an interval of 45 min for analysis. The concentration of the RhB was determined by monitoring the height of the maximum (λ = 554) of the absorption in UV-vis spectra.
3 Results and discussion
Figure 2 compares the crystallinity of as-synthesized Ti1-xSnxO2 nanocrystals with various Sn-doped contents. The diffraction pattern of as-synthesized TiO2 nanocrystals match well to that of anatase structure with intense (101) (004), and (200) reflections, corresponding to the 25.4°, 37.8° and 48.1° 2θ angles (JCPD No. 04-0477). Consistently, as-synthesized SnO2 nanocrystals show crystallized rutile structure with well-defined peaks at 25.3° and 27.4°corresponding to (101) and (110) plane (JPCD No. 84-1286), respectively. Upon increasing Sn-doped, the reflection of Ti1-xSnxO2 compounds (x ≤ 0.05) almost retain the anatase phase but a shift toward lower 2θ angels (∼0.1°) at (101) peak position. While Ti1-xSnxO2 (0.05 < x ≤ 0.4) exhibit two crystalline structures with strong anatase and weak rutile, indicative of crystalline phase transition. For Ti1-xSnxO2 (0.6 < x ≤ 0.8), their characteristic peak positions are consistent with rutile SnO2 (110) peaks at 26.6° and anatase TiO2 reflection gradually disappeared.
To further confirm the Ti1-xSnxO2 formed in a single crystalline phase, Figure 3 compares experimental lattice parameters (a, c) and unit cell volume (V = ac2) of the anatase Ti1-xSnxO2 (x ≤ 0.05) and the rutile Ti1-xSnxO2 (0.5 < x ≤ 0.8) according to Vegard’s law (Kong et al., 2019). The Vegard’s law is an approximate empirical rule that predicts the relationship between lattice constant and the concentration of the constituent elements solid solution, as describe as:
where a is the lattice parameter for the solid solution, a1 and a2 are lattice parameters of individual oxide, and x1 is the mole fraction of metal ions. Figure 3A shows the linear trend of lattice parameters a for the Ti1-xSnxO2 compounds, indicating that the hetero-Sn atom-doped into the TiO2 lattice brings the lattice expansion. Similarly, the lattice parameter c of rutile Ti1-xSnxO2 with high Sn-contents (0.5 < x ≤ 0.8) retains the identical trend, as shown in Figure 3B. In contrast, the lattice parameters c of anatase Ti1-xSnxO2 with low concentration of Sn-contents shows linearly decrease, implying large anisotropy compressibility occurs throughout anatase TiO2 crystal. The different compressibility (κ, κa > κc) causes more denser cell lattice with increasing Sn contents, which is consistent with impact of the external stress on solid solution structure (Prasad and Mikula, 2000). Additionally, the phase separation may cause certain positive deviate on due to spinodal deposition. Table 1 summarized the anatase-rutile phase distribution of TiO2, Ti1-xSnxO2 (0 < x < 1), and SnO2 according to calculation of the lattice parameters and mass fraction of rutile phase.
where WR is the mass fraction of rutile phase, IR is correction factor which indicates the difference of scattering intensities between crystalline structures, and IA and IR represent the integrated intensities of the anatase (101) and rutile (110) reflection, respectively. The mass fraction of rutile phase continuously increases from 14.8 wt% for Ti0.9Sn0.1O2 to 60.6 wt% for Ti0.4Sn0.1O2. Indeed, Sn atoms in TiO2 lattices could provide large number of dissimilar boundaries, which would suppress the crystal growth of TiO2 in the dealloying process, finally leading to extremely fine nanostructure in TiO2-SnO2 solid solution (Sayılkan et al., 2008; Wu et al., 2017). Hence, such a series of Ti1-xSnxO2 (0 < x < 1) compounds underwent pure anatase, mixed anatase-rutile to pure rutile phases, respectively, which provide a fundamental understanding of the relationship between electronic structure and dye-sensitized photocatalytic degradation.
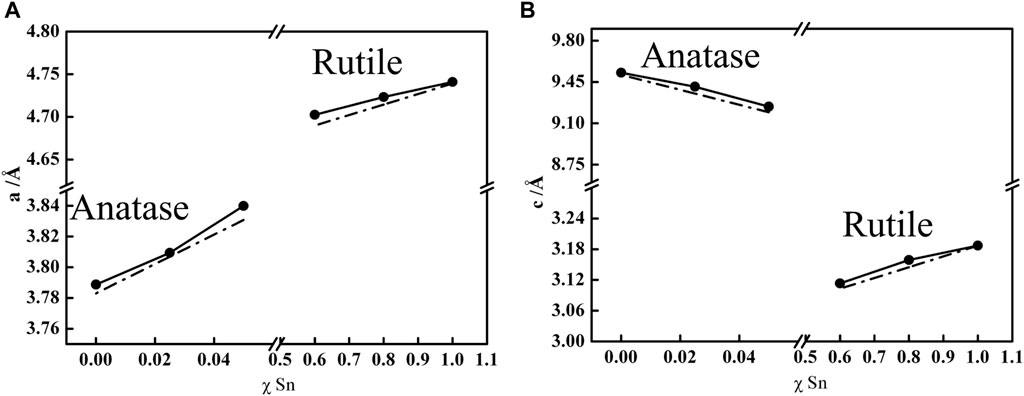
FIGURE 3. Dependence of the mole fraction of Sn (χ) on the a (A) and c (B) lattice parameters of Ti1-xSnxO2 (0 < x < 1) solid solution (solid lines). Dash dot lines represent the theoretical values according to Vegard’s law calculation.
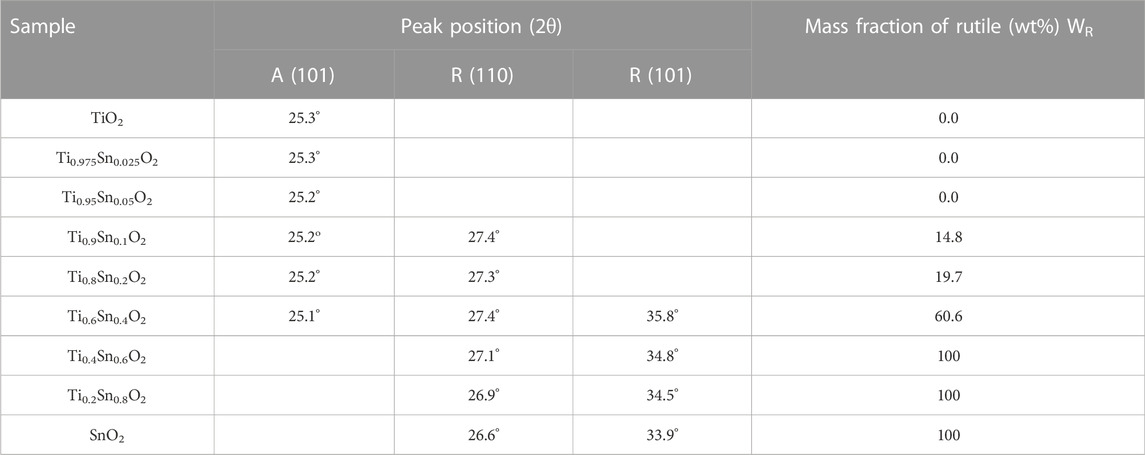
TABLE 1. The summary of lattice parameters of (101), rutile (110) and (101) plane from anatase lattice; and mass fraction of rutile phase (WR).
The morphology and elements analysis of Ti1-xSnxO2 (0 < x < 1) compounds obtained after self-assembly are characterized by scanning electron microscope (SEM) combined with energy-dispersive X-ray (EDX), as shown in Figures 4A,B. All samples show similarly spherical morphology with a diameter range from 150 to 500 nm, exemplified by Ti0.8Sn0.2O2. The bestrewed pot-holes on the spheres surface are also observed arising from the stacking of hard-sphere crystals. The uniform distribution of each element suggests that the presence of Ti and Sn atoms throughout crystalline structure. Transmission electron microscopic (TEM) image clearly displays the as-sprayed Ti0.8Sn0.2O2 particles with non-porous spherical morphology before sintering (Figure 4C). After sintering, the compounds become highly porous due to removal of surfactant, and each sphere is composed of uniform nanocrystals with diameter around 5–6 nm. High-resolution TEM (HRTEM) in Figure 4D shows the ultrafine nanocrystals with an interlayer spacing of 2.32 Å, corresponding to the (200) plane of rutile TiO2 (∼2.29 Å). This result is consistent with the XRD patterns, showing initial extension of c axis occurs during the anatase-rutile conversion at higher Sn-contents doping. Such hierarchically porous design and highly crystalline structure can provide high surface area and fast kinetics, which favors the transport of charge carriers and prevent the recombination of photoelectron-hole pairs.
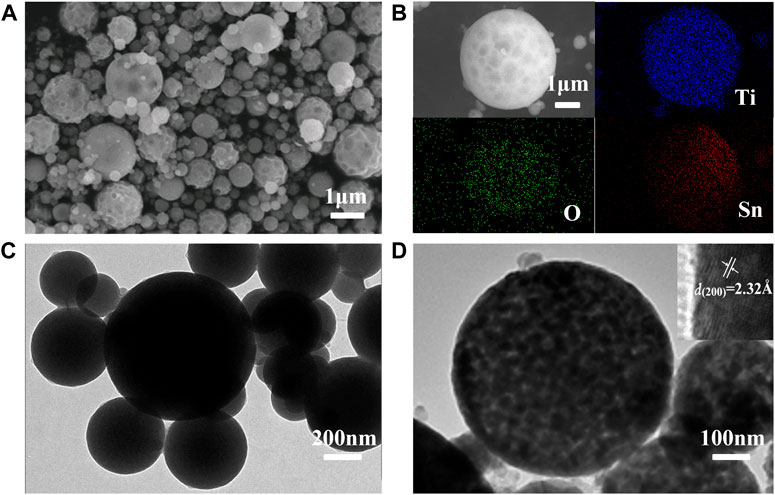
FIGURE 4. (A,B) SEM and elemental mapping images and (C,D) TEM images of the Ti0.8Sn0.2O2 solid-solution after sintering at 550°C for 3 h.
Nitrogen sorption experiment was further conducted to probe the pore structure. Figure 5 shows the nitrogen sorption isotherms and pore size distribution of anatase Ti0.8Sn0.2O2 solid solution with rutile-phased fraction of 20 wt%, confirming a hierarchical pore structure including micropore, mesopore, and macropores arising from particles aggregation. The significant nitrogen uptake at the relative pressures at 0.4–0.6 is consistent with a mesopore network with a narrow pore distribution of ∼10 nm (inset). Table 2 summarizes the surface area and pore structure parameters of Ti1-xSnxO2 (0 < x ≤ 0.4). Upon increasing the Sn-doped contents, the surface areas of Ti1-xSnxO2 compounds shows significantly decrease from 77.58 m2·g−1–44.96 m2·g−1 due to introduction of heavier Sn atoms. Additionally, the average pore volumes of Ti1-xSnxO2 maintain approximately ∼0.15 cm2·g−1.
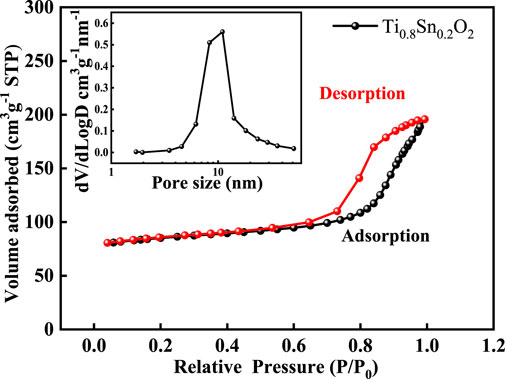
FIGURE 5. Nitrogen sorption isotherms and pore size distribution (inset) of the Ti0.8Sn0.2O2 compound.
To further understand the electronic properties depended transformation between anatase and rutile phase, the elemental composition and valence state in anatase Ti0.95Sn0.05O2 and anatase-rutile Ti0.8Sn0.2O2 are conducted by X-ray photoelectron spectra (XPS), as shown in Figure 6. Figure 6A shows the Ti 2p fitting curves for, which presents two Ti 2p peaks at E = 458.2 eV and E = 463.7 eV for Ti 2p3/2 and Ti 2p1/2 lines, respectively. Which is consistent with the values of Ti4+ reported previously (Fan et al., 2021). Meanwhile, Figure 6B represents typical Sn 3d curves for Ti0.95Sn0.05O2 and Ti0.8Sn0.2O2 with both peaks at 494.2 eV and 485.9 eV for Sn 3d3/2 and Sn 3d5/2 lines, interpolating peak positions of the metallic Sn and SnO2 at 493.1/495.0 eV and 484.7/486.4 eV for Sn 3d3/2/Sn 3d5/2 lines, which can be ascribed to the Sn4+ species. These results indicate no changes in valence state of Sn and Ti during phase transformation, implying the distorted degree in tetragonal structure is neglected at low Sn concentration. Together with XPS analysis, Table 3 compares the atomic concentration ratios of O 1 s and Ti 2p for undoped TiO2, Ti0.95Sn0.05O2 and Ti0.8Sn0.2O2. The concentration ratio of Ti 2p to O 1 s decrease with increasing Sn contents, suggesting the Sn-doped in the tetragonal TiO2 lattice for formation of Ti-O-Sn bonding.
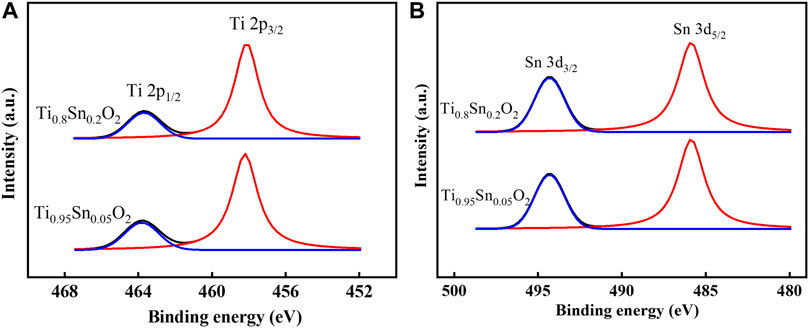
FIGURE 6. XPS curves fitted of (A) Ti 2p spectra of Ti0.95Sn0.05O2 and Ti0.8Sn0.2O2, (B) Sn 3d spectra of Ti0.95Sn0.05O2 and Ti0.8Sn0.2O2.
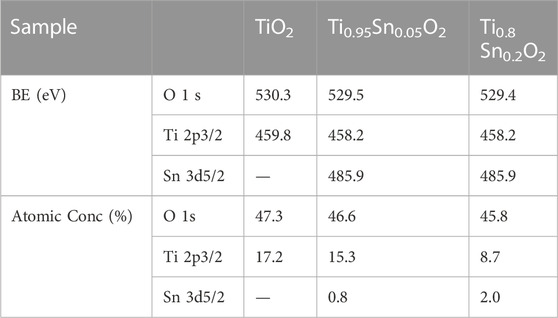
TABLE 3. States of Ti0.95Sn0.05O2 and Ti0.8Sn0.2O2 solid solution and elemental surface composition determined by XPS.
To demonstrate this intricate structure-function correction, we evaluate the photoactivity of the porous Ti1-xSnxO2 (0.05 ≤ x ≤ 0.4) nanoparticles in degradation of RhB dye under visible light irradiation, as shown in Figure 7. The blank experiment showed that the concentration of RhB solution without catalyst maintain during 90 min, indicating the RhB molecules are relatively stable and hard to decompose. Before photocatalytic degradation, it is necessary to the eliminate an adsorption effect by stirring the mixtures in the dark for 1 h. Then the Ti1-xSnxO2 compounds were added in RhB solution being treated in the dark for another 60 min. All the samples have strong physical adsorption abilities, but porous solid solution can enhance adsorption ability than the blank samples. After adsorption, the next photocatalytic degradation experiments kept the RhB concentration at equilibrium as the starting point. Upon increasing Sn-doped contents, the Ti0.9Sn0.1O2 and Ti0.8Sn0.2O2 solutions with mixed anatase-rutile phases exhibit the higher photoactivity (RhB degradation yield ≈50% and 70%, respectively after irradiation for 120 min) than that of the anatase Ti0.95Sn0.05O2 solution (RhB degradation yield <10% after irradiation for 120 min). However, the photocatalytic activity of Ti0.6Sn0.4O2 with higher rutile phased of 60 wt% decrease with increasing Sn content because the RhB degradation yield is only about 15% after irradiation for 120 min.
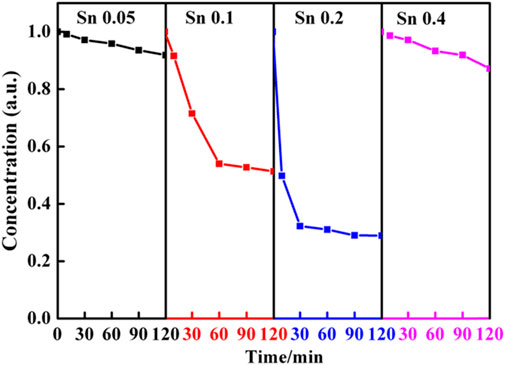
FIGURE 7. Dependence of the Sn-doped contents on the reaction time of Ti1-xSnxO2 (0.05 ≤ x ≤ 0.4) compounds with RhB under visible-light harvester.
In the heterojunction composed of TiO2 and SnO2, the photogenerated electrons can transfer from TiO2 to SnO2 phase with no applied voltage (Fan et al., 2016). Because of their identical rutile phase, tetragonal crystal structures, ionic radius (0.690 for Sn4+ and 0.605 for Ti4+), and electronic characteristics, SnO2 and TiO2 may also form solid solutions coupling system (Sayılkan, 2007; Huang et al., 2015). Thus, it is very essential to probe the relationship between electronic structure and photoactivity in TixSn1-xO2 solutions. Figure 8 illustrates the dependence of band gap of Ti1-xSnxO2 (0 ≤ x ≤ 1) with increasing Sn-doping. Theoretical considerations by Long et al. suggest that for substitutionally Sn-doped anatase TiO2, the band gap increases ∼0.13 eV owing to high formation energy while maintain anatase phase (Long et al., 2009). However, the substitution of Sn for rutile TiO2 brings a red-shift of the adsorption edge deriving from the decrease of Sn 5s gap state in the conduction band (Chang et al., 2019). Consistent with the theoretical analysis, the experimental band gaps of the anatase Ti1-xSnxO2 (x = 0.05 and 0.025, respectively) solid solutions indeed increase to 3.22 and 3.29 eV, respectively (Ren et al., 2020). High Sn-doped contents causes a transition from anatase to rutile phase, and the band gap of solution with mixed phase should be reasonably neutralized in between that of single phase using their mass fraction (Chen et al., 2010; Mohammad et al., 2021). However, it is worth noting that the band gap values of Ti0.9Sn0.1O2 and Ti0.8Sn0.2O2 decreases to 2.98 and 2.92 eV, respectively. This is possibly attributed to transition of photoexcited electrons and holes from rutile to energy states of anatase phase, which needs very low energy about 0.2∼0.25 eV. Meanwhile, we discovered that the presence of crystalline interface within mixed phases effectively reduces electron-hole recombination and creates more oxygen vacancies to enhance visible-light harvesting ability.
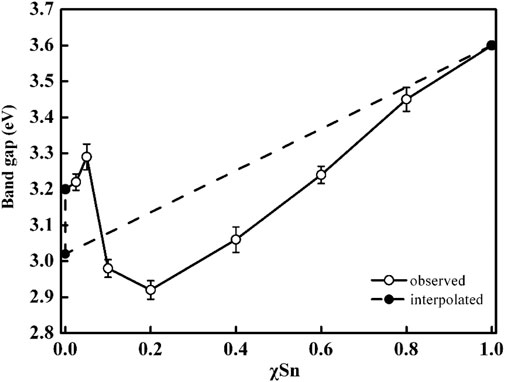
FIGURE 8. Variation in optical band gap of Ti1-xSnxO2 (0 < x < 1) samples. Dashed line is a linear interpolation between the band gap values for anatase TiO2 (3.2 eV), rutile TiO2 (3.0 eV), and SnO2 (3.60 eV).
Continuation of this process gradually separates photoexcited electron-hole pairs, sequentially providing more photo-charges needed for the photocatalytic reaction and fast charge migration, as shown in Scheme of Figure 9. The rapid increased band gap values of Ti1-xSnxO2 (0.4 ≤ x ≤ 0.8) is attributed to the more Sn doping, leading the conduction band to shift toward a higher energy, which accounts for the observed decreasing photocatalytic activity at a higher Sn content (e.g., x ≥ 0.8). Rather than facilitate charge migration and reduce charge recombination, the charge trapping may take effect as centers for electro-hole recombination and absorb the visible light, described as follow reaction 1 and 2 (Golestanbagh et al., 2018; Shi et al., 2007):
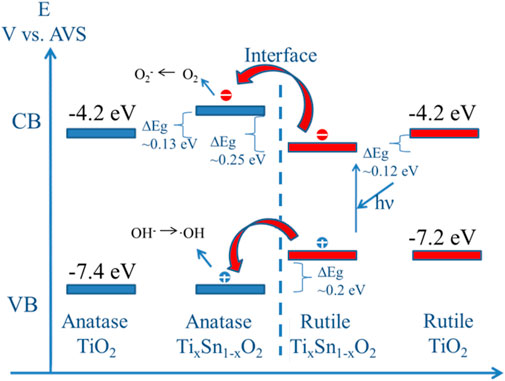
FIGURE 9. Scheme of electron transition between mixed Ti1-xSnxO2 (0.1 ≤ x ≤ 0.4) solutions. The absolute valence band and conduction band edges relative to vacuum level of TiO2 are obtained from the reference.
The visible-spectra sensitization of Sn-doped TiO2 is attribute from charge transfer bands of peroxotitanium or the formation of Sn II) with stable lower oxidation state (Serpone., 2006). The loss of O atoms creates the formation of color center due to the charge trapped in cavity (Vo) left behind (reaction 3). Such the impacted effects on visible-light driven reactivity for color centers are even more important than the narrowing band gap caused by doping Bjelajac et al. (2018).
For the improved spectral response in the visible region, the Ti0.8Sn0.2O2 solution also shows relatively stable photodegradation performance, as shown in Figure 10. The preformed powder is centrifuged and collected after every irradiation cycle and subsequently dispersed in a fresh RhB aqueous solution without any further purification. Compare with the first cycle, the photocatalyst displays a less than 10% decrease in activity after seven cycle times, endowing a cycling stability for solution with a mixed phase.
4 Conclusion
In summary, a facile and effective aerosol-spray strategy is developed to prepare high-performance photocatalyst by fabricating mesoporous Ti1-xSnxO2 (0 < x < 1) solid solution. Ti1-xSnxO2 nanocrystals with various Sn-doped contents are self-assembled into mesoporous spheres provide effective transport of charge carrier. As-prepared hierarchical architecture shows several advantages as a visible-light photocatalyst. First, mesoporous Ti1-xSnxO2 spheres enhance the advantageous features of conventional titania and tin oxides. Second, heterostructure-based titania compounds further enhance photoinduced surface redox reactions and kinetic process in the visible region. Third, with increasing Sn-doped concentration, the mechanism between the anatase-rutile phase transition of Ti1-xSnxO2 compounds and photocatalytic activity is further revealed. Therefore, as-prepared mesoporous Ti1-xSnxO2 solid solution can effectively enhanced charge separation, and accelerated proton mass transfer. This demonstration of creating solid solutions offers a viable, cost-efficient method for the photocatalytic degradation of RhB, and this prototype has the potential to inspire the development of novel photocatalysts, such as splitting water, reducing CO2, and fixing N2.
Data availability statement
The original contributions presented in the study are included in the article/supplementary material, further inquiries can be directed to the corresponding author.
Author contributions
WY and NG contributed to design of the study and organized the database. WY wrote the first draft of the manuscript. JH and WY wrote sections of the manuscript. All authors contributed to manuscript revision, read, and approved the submitted version.
Funding
This work was financially supported by funding from the National Natural Science Foundation of China (NSFC 51972207), Science and Technology Commission of Shanghai Municipality (No. 19DZ2271100), and Science and Technology Commission of Shanghai Municipality (No. 21ZR1425100).
Conflict of interest
The authors declare that the research was conducted in the absence of any commercial or financial relationships that could be construed as a potential conflict of interest.
Publisher’s note
All claims expressed in this article are solely those of the authors and do not necessarily represent those of their affiliated organizations, or those of the publisher, the editors and the reviewers. Any product that may be evaluated in this article, or claim that may be made by its manufacturer, is not guaranteed or endorsed by the publisher.
References
Albornoz, L. L., Bortolozzi, J. P., Banús, E. D., Brussino, P., da Silva, S. W., Bernardes, A. M., et al. (2021). Synthesis and characterization of immobilized titanium-zirconium Sn-doped oxides onto metallic meshes and their photocatalytic activity for erythromycin mineralization. Chem. Eng. J. 414, 128891. doi:10.1016/j.cej.2021.128891
Asokan, K., Park, J. Y., Choi, S., Chang, C., and Kim, S. S. (2010). Stabilization of the anatase phase of Ti1−x Sn x O2 (x. Nano Res. 3, 256–263. doi:10.1007/s12274-010-1028-y
Bjelajac, A., Petrović, R., Djokic, V., Matolin, V., Vondraček, M., Dembele, K., et al. (2018). Enhanced absorption of TiO2 nanotubes by N-doping and CdS quantum dots sensitization: Insight into the structure. RSC Adv. 8, 35073–35082. doi:10.1039/c8ra06341a
Chang, J., Jiang, Z. Y., Zhang, Z. Y., Lin, Y. M., Tian, P. L., Zhou, B., et al. (2019). Theoretical studies of photocatalytic behaviors of isoelectronic C/Si/Ge/Sn-doped TiO2: Dft + U. Appl. Surf. Sci. 484, 1304–1309. doi:10.1016/j.apsusc.2018.12.252
Chen, L. C., Huang, C. M., Hsiao, M. C., and Tsai, F. R. (2010). Mixture design optimization of the composition of S, C, SnO2-codoped TiO2 for degradation of phenol under visible light. Chem. Eng. J. 165, 482–489. doi:10.1016/j.cej.2010.09.044
Chen, H., Tian, P., Fu, L., Wan, S., and Liu, Q. (2022). Hollow spheres of solid solution Fe7Ni3S11/CN as advanced anode materials for sodium ion batteries. Chem. Eng. J. 430, 132688. doi:10.1016/j.cej.2021.132688
Fan, W., Yu, X., Lu, H. C., Bai, H., Zhang, C., and Shi, W. (2016). Fabrication of TiO2/RGO/Cu2O heterostructure for photoelectrochemical hydrogen production. Appl. Catal. B Environ. 181, 7–15. doi:10.1016/j.apcatb.2015.07.032
Fan, H., Wang, R., Xu, Z., Duan, H., and Chen, D. (2021). Migration and enrichment behaviors of Ca and Mg elements during cooling and crystallization of boron-Bearing titanium slag melt. Crystals 11, 888. doi:10.3390/cryst11080888
Galvão, T. L., Sousa, I., Wilhelm, M., Carneiro, J., Opršal, J., Kukačková, H., et al. (2018). Improving the functionality and performance of AA2024 corrosion sensing coatings with nanocontainers. Chem. Eng. J. 341, 526–538. doi:10.1016/j.cej.2018.02.061
Golestanbagh, M., Parvini, M., and Pendashteh, A. (2018). Preparation, characterization and photocatalytic properties of visible-light-driven CuO/SnO2/TiO2 photocatalyst. Catal. Lett. 148, 2162–2178. doi:10.1007/s10562-018-2385-5
González-Gutiérrez, A. G., Pech-Canul, M. A., Chan-Rosado, G., and Sebastian, P. J. (2019). Studies on the physical and electrochemical properties of Ni-P coating on commercial aluminum as bipolar plate in PEMFC. Fuel 235, 1361–1367. doi:10.1016/j.fuel.2018.08.104
Huang, M., Yu, J., Li, B., Deng, C., Wang, L., Wu, W., et al. (2015). Intergrowth and coexistence effects of TiO2-SnO2 nanocomposite with excellent photocatalytic activity. J. Alloys Compd. 629, 55–61. doi:10.1016/j.jallcom.2014.11.225
Kong, L., Guo, J., Makepeace, J. W., Xiao, T., Greer, H. F., Zhou, W., et al. (2019). Rapid synthesis of BiOBrxI1-x photocatalysts: Insights to the visible-light photocatalytic activity and strong deviation from Vegard's law. Catal. Today 335, 477–484. doi:10.1016/j.cattod.2019.02.013
Liang, J., Wei, W., Zhong, D., Yang, Q., Li, L., and Guo, L. (2012). One-step in situ synthesis of SnO2/graphene nanocomposites and its application as an anode material for Li-ion batteries. ACS Appl. Mat. Interfaces 4, 454–459. doi:10.1021/am201541s
Liu, Y., Yao, W., Wang, G., Wang, Y., Moita, A. S., Han, Z., et al. (2016). Reversibly switchable wettability on aluminum alloy substrate corresponding to different pH droplet and its corrosion resistance. Chem. Eng. J. 303, 565–574. doi:10.1016/j.cej.2016.06.038
Liu, J., Zhao, Z., Xu, C., and Liu, J. (2019). Structure, synthesis, and catalytic properties of nanosize cerium-zirconium-based solid solutions in environmental catalysis. Chin. J. Catal. 40, 1438–1487. doi:10.1016/s1872-2067(19)63400-5
Li, Y., Zhang, J., Chen, Q., Xia, X., and Chen, M. (2021). Emerging of heterostructure materials in energy storage: A review. Adv. Mat. 33, 2100855. doi:10.1002/adma.202100855
Liu, C., Zhang, Y., Wu, J., Dai, H., Ma, C., Zhang, Q., et al. (2022). Ag-Pd alloy decorated ZnIn2S4 microspheres with optimal Schottky barrier height for boosting visible-light-driven hydrogen evolution. J. Mater. Sci. Technol. 114, 81–89. doi:10.1016/j.jmst.2021.12.003
Liu, C., Zhang, Q., and Zou, Z. (2023). Recent advances in designing ZnIn2S4-based heterostructured photocatalysts for hydrogen evolution. J. Mater. Sci. Technol. 139, 167–188. doi:10.1016/j.jmst.2022.08.030
Long, R., Dai, Y., Meng, G., and Huang, B. (2009). Energetic and electronic properties of X- (Si, Ge, Sn, Pb) doped TiO2 from first-principles. Phys. Chem. Chem. Phys. 11, 8165–8172. doi:10.1039/b903298c
Luo, M., Xu, Y. E., and Song, Y. X. (2018). Modulation of band gap by normal strain in SiC-based heterostructures. Optik 154, 634–639. doi:10.1016/j.ijleo.2017.10.044
Mohammad, A., Khan, M. E., Cho, M. H., and Yoon, T. (2021). Fabrication of binary SnO2/TiO2 nanocomposites under a sonication-assisted approach: Tuning of band-gap and water depollution applications under visible light irradiation. Ceram. Int. 47, 15073–15081. doi:10.1016/j.ceramint.2021.02.065
Nuansing, W., Ninmuang, S., Jarernboon, W., Maensiri, S., and Seraphin, S. (2006). Structural characterization and morphology of electrospun TiO2 nanofibers. Mater. Sci. Eng. B 131, 147–155. doi:10.1016/j.mseb.2006.04.030
Pelicano, C. M., Saruyama, M., Takahata, R., Sato, R., Kitahama, Y., Matsuzaki, H., et al. (2022). Bimetallic synergy in ultrafine cocatalyst alloy nanoparticles for efficient photocatalytic water splitting. Adv. Funct. Mater. 32, 2202987. doi:10.1002/adfm.202202987
Prasad, L. C., and Mikula, A. (2000). Concentration fluctuations and interfacial adhesion at the solid-liquid interface between Al2O3 and al-Sn liquid alloys. High. Temp. Mat. Process. 19, 61–69. doi:10.1515/htmp.2000.19.1.61
Pudukudy, M., Yaakob, Z., Mazuki, M. Z., Takriff, M. S., and Jahaya, S. S. (2017). One-pot sol-gel synthesis of MgO nanoparticles supported nickel and iron catalysts for undiluted methane decomposition into COx free hydrogen and nanocarbon. Appl. Catal. B Environ. 218, 298–316. doi:10.1016/j.apcatb.2017.04.070
Qin, Q., Wang, J., Xia, Y., Yang, D., Zhou, Q., Zhu, X., et al. (2021). Synthesis and characterization of Sn/Ni single doped and Co-doped anatase/rutile mixed-crystal nanomaterials and their photocatalytic performance under UV-visible light. Catalysts 11, 1341. doi:10.3390/catal11111341
Reddy, B. M., Saikia, P., and Bharali, P. (2008). Highly dispersed Ce x Zr1−x O2 nano-oxides over alumina, silica and titania supports for catalytic applications. Catal. Surv. Asia 12, 214–228. doi:10.1007/s10563-008-9053-5
Ren, B., Jin, Q., Li, Y., Li, Y., Cui, H., and Wang, C. (2020). Activating titanium dioxide as a new efficient electrocatalyst: From theory to experiment. ACS Appl. Mat. Interfaces 12, 11607–11615. doi:10.1021/acsami.9b21575
Ruiz Esquius, J., Algara-Siller, G., Spanos, I., Freakley, S. J., Schlögl, R., and Hutchings, G. J. (2020). Preparation of solid solution and layered IrOx-Ni(OH)2 oxygen evolution catalysts: Toward optimizing iridium efficiency for OER. ACS Catal. 10, 14640–14648. doi:10.1021/acscatal.0c03866
Sayılkan, H. (2007). Improved photocatalytic activity of Sn4+-doped and undoped TiO2 thin film coated stainless steel under UV- and VIS-irradiation. Appl. Catal. A General 319, 230–236. doi:10.1016/j.apcata.2006.12.012
Sayılkan, F., Asiltürk, M., Tatar, P., Kiraz, N., Şener, Ş., Arpaç, E., et al. (2008). Photocatalytic performance of Sn-doped TiO2 nanostructured thin films for photocatalytic degradation of malachite green dye under UV and VIS-lights. Mater. Res. Bull. 43, 127–134. doi:10.1016/j.materresbull.2007.02.012
Serpone, N. (2006). Is the band gap of pristine TiO2 narrowed by anion- and cation-doping of titanium dioxide in second-generation photocatalysts? J. Phys. Chem. B 110, 24287–24293. doi:10.1021/jp065659r
Sharon, M., Modi, F., Modi, M., and Sharon, M. (2016). Titania based nanocomposites as a photocatalyst: A review. Mat. Sci. 3, 1236–1254. doi:10.3934/matersci.2016.3.1236
Shi, Z. M., Yan, L., Jin, L. N., Lu, X. M., and Zhao, G. (2007). The phase transformation behaviors of Sn2+-doped Titania gels. J. Non-Crystalline Solids 353, 2171–2178. doi:10.1016/j.jnoncrysol.2007.02.048
Singh, A., Sikarwar, S., Verma, A., and Chandra Yadav, B. C. (2021). The recent development of metal oxide heterostructures based gas sensor, their future opportunities and challenges: A review. Sensors Actuators A Phys. 332, 113127. doi:10.1016/j.sna.2021.113127
Wang, P., Song, T., Gao, G., Matras-Postolek, K., and Yang, P. (2022). SnO2 clusters embedded in TiO2 nanosheets: Heterostructures and gas sensing performance. Sensors Actuators B Chem. 357, 131433. doi:10.1016/j.snb.2022.131433
Wu, L., Yan, H., Li, X., and Wang, X. (2017). Characterization and photocatalytic properties of SnO 2 -TiO 2 nanocomposites prepared through gaseous detonation method. Ceram. Int. 43, 1517–1521. doi:10.1016/j.ceramint.2016.10.124
Wu, Y., Chen, Y., Li, D., Sajjad, D., Chen, Y., Sun, Y., et al. (2022). Interface engineering of organic-inorganic heterojunctions with enhanced charge transfer. Appl. Catal. B Environ. 309, 121261. doi:10.1016/j.apcatb.2022.121261
Yu, D. G., Li, J. J., Williams, G. R., and Zhao, M. (2018). Electrospun amorphous solid dispersions of poorly water-soluble drugs: A review. J. Control. Release 292, 91–110. doi:10.1016/j.jconrel.2018.08.016
Keywords: TiO2, solid solution, aerosol-spray, self-assembly, photocatalyst
Citation: Yu W, Geng N, Han J, Yu W and Peng Y (2022) Mesoporous crystalline Ti1-xSnxO2 (0 < x < 1) solid solution for a high-performance photocatalyst under visible light irradiation. Front. Chem. 10:1111435. doi: 10.3389/fchem.2022.1111435
Received: 29 November 2022; Accepted: 05 December 2022;
Published: 14 December 2022.
Edited by:
Chao Liu, Yancheng Institute of Technology, ChinaReviewed by:
Xiaolei Yuan, Nantong University, ChinaJinchen Fan, University of Shanghai for Science and Technology, China
Copyright © 2022 Yu, Geng, Han, Yu and Peng. This is an open-access article distributed under the terms of the Creative Commons Attribution License (CC BY). The use, distribution or reproduction in other forums is permitted, provided the original author(s) and the copyright owner(s) are credited and that the original publication in this journal is cited, in accordance with accepted academic practice. No use, distribution or reproduction is permitted which does not comply with these terms.
*Correspondence: Yiting Peng, cHl0XzExMDhAc2hpZXAuZWR1LmNu