- 1Hebei Provincial Laboratory of Inorganic Nonmetallic Materials, College of Materials Science and Engineering, North China University of Science and Technology, Tangshan, China
- 2Traditional Chinese Medical College, North China University of Science and Technology, Tangshan, China
- 3School of Pharmacy, North China University of Science and Technology, Tangshan, China
- 4James Watt School of Engineering, University of Glasgow, Glasgow, United Kingdom
Photocatalytic synthesis of hydrogen peroxide under mild reaction conditions is a promising technology. This article will review the recent research progress in the design of photocatalytic H2O2 synthesis systems. A comprehensive discussion of the strategies that could solve two essential issues related to H2O2 synthesis. That is, how to improve the reaction kinetics of H2O2 formation via 2e− oxygen reduction reaction and inhibit the H2O2 decomposition through a variety of surface functionalization methods. The photocatalyst design and the reaction mechanism will be especially stressed in this work which will be concluded with an outlook to show the possible ways for synthesizing high-concentration H2O2 solution in the future.
1 Introduction
H2O2 is an indispensable chemical in daily life. It has many applications in fields such as biology (Chang et al., 2021; Noh et al., 2020; Guarino et al., 2019), medicine (Andersen et al., 2006; Kozlova et al., 2015; Wang Y. et al., 2020), chemical industry (Chung et al., 2020; Zhang et al., 2021), environmental protection (Dinakar et al., 2020; Moreno, 2011). As a clean oxidant, the decomposition of H2O2 only yields H2O, which does not pose an environmental risk. Currently, the anthraquinone (AQ) method is the main method for the industrial production of H2O2 (Sterenchuk et al., 2018). The AQ method for H2O2 synthesis includes two steps: hydrogenation and oxidation (Campos-Martin et al., 2006; Halder and Lawal, 2007; Gao et al., 2020). In this method, AQ is used as an intermediate, and the hydrogenation reaction is first performed with palladium catalyst (Chen, 2008; Edwards and Hutchings, 2008; Han et al., 2015). Then, oxygen is added to oxidize the hydroanthraquinone (AQH2) back to AQ and produce H2O2 (Figure 1). However, the AQ method not only has the risk of explosion, but consumes a lot of energy and organic solvent (Chen, 2008; Jia et al., 2018). Therefore, it is crucial to develop a safe and direct method to synthesize H2O2. The methods of direct H2O2 synthesis mainly includes electrocatalysis (Apaydin et al., 2018; Du et al., 2020; Sun et al., 2020; Shu et al., 2021), photocatalysis (Chen et al., 2018; Kormann et al.), and thermal catalysis (Adams et al., 2021). The electrocatalytic H2O2 synthesis has a high yield but it needs to consume useful electricity. Thermocatalytic H2O2 synthesis from oxygen and hydrogen also faces the risk of explosion when mixing the gases. The emerging photocatalytic H2O2 synthesis only uses solar energy to drive reaction without introducing hydrogen.
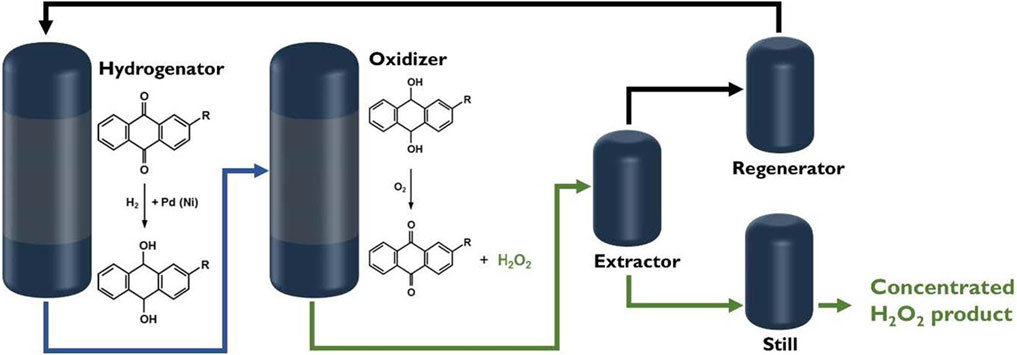
FIGURE 1. Flow chart of synthesis of H2O2 by AQ method (Yang et al., 2018).
During photocatalytic H2O2 synthesis, the electron is first excited from the valence band to the conduction band of the photocatalyst. Then, it participates in oxygen reduction reactions (ORR) on the surface to generate H2O2. H2O2 synthesis via oxygen reduction can undergo two pathways. The step-by-step single-electron pathway is the first one (Eqs. 1–3), which is characterized by the presence of superoxide (HO2•) intermediate. The other is the direct two-electron (2e–) pathway (Eq. 1 and Eq. 4). Which one of these occurs can be confirmed by detecting the intermediate HO2• (Viswanathan et al., 2012; Baran et al., 2018; Fukuzumi et al., 2018; Haider et al., 2019; Anantharaj et al., 2021; Yang, 2021; Guo et al., 2022).
Photocatalytic H2O2 generation is also accompanied by a decomposition reaction, which is the root cause of poor reaction stability. The decomposition process includes photolysis and light-independent decomposition. Taking TiO2 as an example, photolysis can occur mainly in four ways (Ⅰ) photogenerated electrons reduce H2O2 to OH−and •OH; (Ⅱ) photogenerated holes oxidize hydrogen peroxide to O2 or superoxide radical •O2−; (Ⅲ) The titanium peroxide complex (Ti-OOH) formed on the surface by the interaction of TiO2 and H2O2 gradually degrades under visible light; (Ⅳ) direct decomposition of H2O2 under ultraviolet light. H2O2 can also be decomposed in ways independent of light, such as pH and temperature.
The formation and decomposition performance of hydrogen peroxide are closely related to the surface properties of semiconductor photocatalysts. First, the high selectivity of cocatalysts to 2e− ORR is needed to improve the photocatalytic H2O2 formation. Second, the functional modifier on photocatalyst can inhibit the decomposition of H2O2. These strategies indicate that surface functionalization of photocatalysts is very important. Considering these issues, we review the recent advances in the design of photocatalysts for H2O2 synthesis in this work.
2 Effect of cocatalyst on photocatalytic activity
2.1 Noble metal cocatalysts
Precious metals are widely used as cocatalysts in electrocatalysis and photocatalysis, while they also show excellent performance in ORR (Zinola et al., 1995; Chen et al., 2017a; Cai et al., 2019; Ignaczak et al., 2019; Jeon et al., 2020). Pt has good ORR performance and strong binding ability to intermediates such as O2 and OH•. When using Pt, generating H2O via 4e–ORR is favored, but it has poor selectivity for 2e–ORR (Kim J. et al., 2018; Chen J. Y. et al., 2020). Among these noble metals, Au has the best selectivity for 2e− ORR, which has achieved efficient photocatalytic H2O2 synthesis in photocatalytic reaction (Jirkovsky et al., 2010; Jirkovsky et al., 2011; Zuo et al., 2019a; Ignaczak et al., 2019; Sun et al., 2020). Zuo et al. studied the influence of a series of noble metal co-catalysts (Pd, Pt, Au, and Ag) on the performance of photocatalytic H2O2 synthesis over g-C3N4. They found that the maximum activity could be achieved when the Au loading amount is very low (0.01 wt%) on g-C3N4 (Figure 2A) (Zuo et al., 2019a). A similar study showed that Au cocatalyst has the highest activity among different precious metals modified g-C3N4 samples (Kim H. I. et al., 2018). Similar high activity was observed over Au loaded TiO2-based photocatalysts (Tsukamoto et al., 2012), (Li L. et al., 2021; Feng et al., 2021).
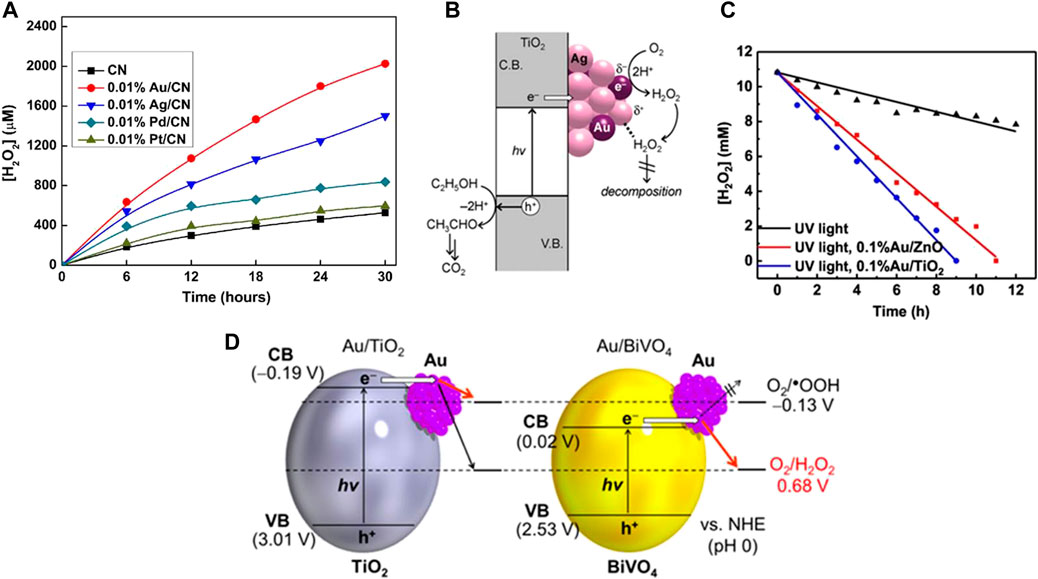
FIGURE 2. (A) Under visible light irradiation (λ > 420 nm), different noble metal loaded CN photocatalyzed H2O2 activity (Zuo et al., 2019a). (B) Mechanism for photocatalytic production of H2O2 on Au-Ag/TiO2 catalyst (Tsukamoto et al., 2012). (C) Photodecomposition of H2O2 under UV light with and without photocatalysts (Meng et al., 2020); (D) Energy diagrams for Au/TiO2 and Au/BiVO4, and reduction potential of O2 (Hirakawa et al., 2016).
The H2O2 yield of Au-Ag alloy cocatalyst supported on the surface of TiO2 was 2.3 times and 3.4 times higher than that of single Au or Ag cocatalyst. The reason was that the loaded Au-Ag alloy was conducive to the separation of electron holes, and the efficient photocatalytic reduction of O2 on Au atom promotes the formation of H2O2 (Figure 2B) (Tsukamoto et al., 2012). However, the activity of Au deposition on ZnO was better than that on TiO2, which is attributed to the more inert surface properties of ZnO than TiO2 when decomposing H2O2 (Figure 2C) (Meng et al., 2020).
Hirakawa et al. (Hirakawa et al., 2016) suggested that activity of Au cocatalyst is affected by the band structure of semiconductor photocatalytsts. They employed Au/BiVO4 photocatalyst to successfully produce H2O2 under visible light irradiation (λ> 420 nm). Since the conduction band potential of BiVO4 (0.02 V vs SHE) is more positive than the one-electron ORR potential (-0.13V) and more negative than the 2e− ORR (0.68 V vs SHE), the 2e− ORR can be selectively promoted while the one-electron ORR is inhibited. Compared with TiO2, BiVO4 has a narrower band gap, which indicates that BiVO4 has a better ability to utilize visible light and 2e ORR selectivity than TiO2 (Figure 2D).
2.2 Non-precious metal cocatalysts
Considering the scarcity and high cost of precious metals, developing non-precious metal co-catalysts for 2e–ORR is crucial (Zhang J. et al., 2020; Yan et al., 2020). For example, the surface of g-C3N4 was loaded with AQ as a cocatalyst. Its activity reached 361 μm/h, which was 4.4 times that of pure g-C3N4 and comparable to some precious metals. This is because, in addition to the 2e− ORR reaction catalyzed by pure g-C3N4, another H2O2 synthesis pathway via hydrogenation (AQ + 2H+ + 2e−→AQH2) and dehydrogenation (AQH2 + O2→AQ + H2O2) plays a key role in the photocatalytic reaction (Kim H. I. et al., 2018). For CoP loaded on g-C3N4, the catalytic activity of CoP/g-C3N4 (70 μM•h−1) was similar to that of Au/g-C3N4 (67.56 μM•h−1) (Zuo et al., 2019a). This can be attributed to the accelerated separation and transfer of g-C3N4 photogenerated charge by CoP (Peng et al., 2017). The method of loading quantum dots to improve visible light absorption and electron mobility is also beneficial to photocatalytic synthesis of H2O2 (Zheng et al., 2018; Zhang M. M. et al., 2020; Liu et al., 2021a). Table 1 summarizes the effects of cocatalysts on hydrogen peroxide production activity.
3 Effect of surface modification on photocatalytic activity
In addition to increasing the activity of H2O2 production by the deposition of co-catalysts, decreasing the decomposition rate via surface modification is essential to maximize the final concentration of H2O2.
3.1 Surface passivation modification
TiO2 can catalyze the decomposition of H2O2 under visible light. Ti-OOH that form on the surface due to the interaction of TiO2 and H2O2 gradually degrade under visible light. This is the main reason for the decrease of H2O2 concentration during reactions (Li et al., 2001; Teranishi et al., 2010). Surface passivation can effectively inhibit the decomposition of H2O2. It can be carried out either by metal oxide passivation or non-metal passivation. Passivation of a photocatalyst with a metal oxide leads to the formation of a heterojunction (Zeng et al., 2017; Zuo et al., 2019b; Awa et al., 2020; Feng et al., 2021). For example, the surface of anatase TiO2 and rutile TiO2 were modified with SnO2 to form SnO2-TiO2 heterojunction. Then, the surface was functionalized with gold nanoparticles, and it was found that the formation activity of H2O2 was improved. This is because the decomposition of H2O2 on the TiO2 surface is inhibited (Figure 3A) (Zuo et al., 2019b). However, the H2O2 photocatalytic synthesis reaction rate of Au modified Bi2O3-TiO2 was better than that of Au/SnO2-TiO2. This is because not only the decomposition of H2O2 is inhibited, but also the carrier recombination in Bi2O3 is inhibited (Feng et al., 2021). A similar phenomenon is found in the heterojunction formed on g-C3N4 (Chen et al., 2017b; Chen X. L. et al., 2020; Liu et al., 2021b).
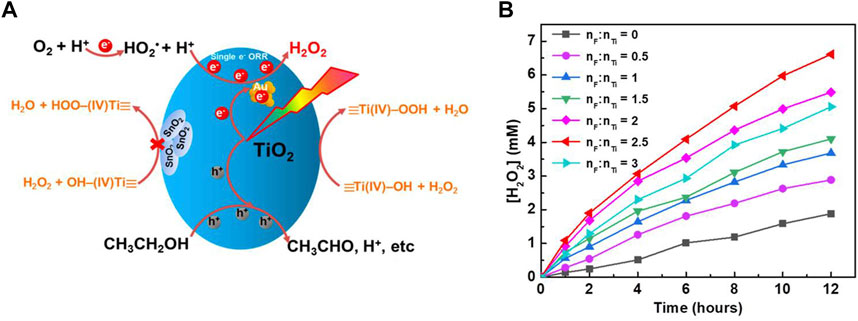
FIGURE 3. (A) Schematic illustration of H2O2 synthesis and decomposition over Au/SnO2 -TiO2 (Zuo et al., 2019b). (B) Photocatalytic H2O2 production over 0.1% Au/F-TiO2 prepared with different F/Ti ratios (Li L. et al., 2021).
Non—metallic surface modification was also effective for improving photocatalytic activity (Maurino et al., 2005; Moon et al., 2014; Zhang M. M. et al., 2020; Li L. et al., 2021). For example, by hydrothermal treatment of TiO2 and NaF to obtain F-TiO2, the decomposition of H2O2 is inhibited. This was due to the fact that the F ion fixed on the TiO2 surface competes with the Ti-OOH formation, thus reducing the Ti-OOH formation. Therefore, it was no longer necessary to add NaF to the photocatalytic reaction medium (Figure 3B) (Li L. et al., 2021).
3.2 Organic molecular modification
Imine organic molecules can be used to modify the surface of g-C3N4 to inhibit the electron-hole pair recombination of g-C3N4 (Shiraishi et al., 2014a; Kofuji et al., 2016; Yang et al., 2017; Goclon and Winkler, 2018; Guo et al., 2020; Zeng et al., 2020). For example, modification of the surface of g-C3N4 with homobendiimide and bibendiimide increased the synthesis activity of H2O2 (Figures 4A–C) (Shiraishi et al., 2014a; Kofuji et al., 2016). Besides the pure g-C3N4 reaction, another H2O2 synthesis pathway (•OH +•OH→H2O2) also plays a key role in the polyimide modified g-C3N4 nanosheets (Yang et al., 2017). In another publication, it was found that the modification of g-C3N4 by β-cyclodextrin can increase its hydrophobicity and affinity for oxygen, thus increasing the yield of H2O2 (Figure 4D) (Zuo et al., 2020).
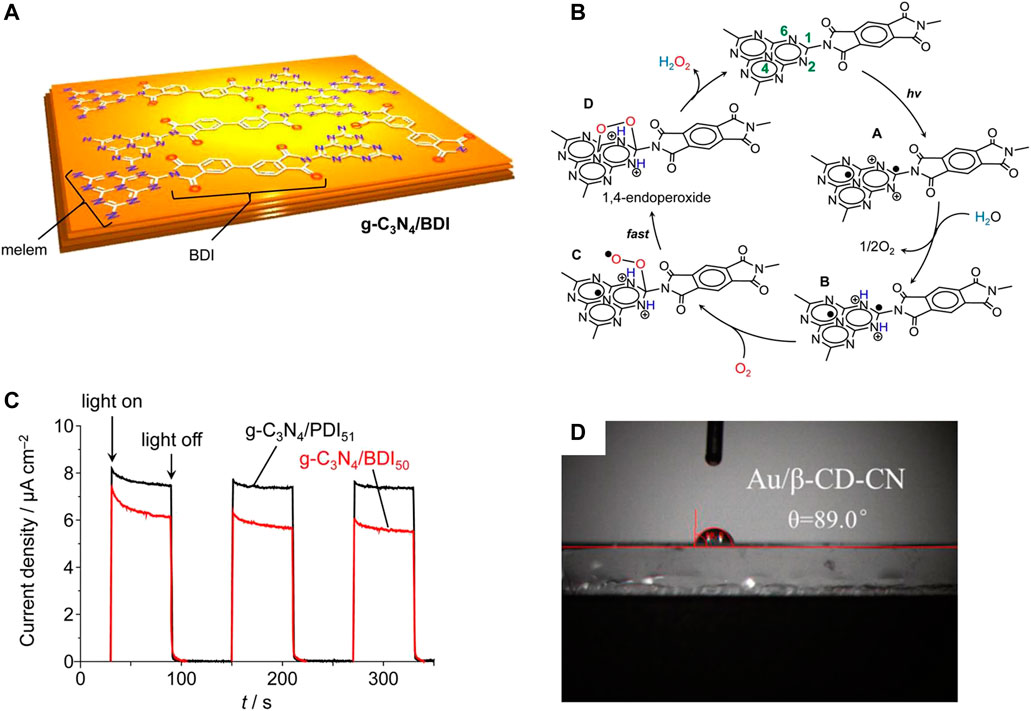
FIGURE 4. (A) Three-dimensional structure of g-C3N4/BDI. (B) Proposed Mechanism for H2O2 Formation on the Photoexcited g-C3N4/BDI Catalyst. (C) Photocurrent response of g-C3N4/BDI50 and g-C3N4/PDI51 in 0.1 M Na2SO4 solution under visible light (λ >420 nm) at a bias of 0.5 V vs. Ag/AgCl (Kofuji et al., 2016). (D) The water contact angle of Au/β-CD-CN (Zuo et al., 2020).
Metal organic frameworks (MOFs) are promising materials that can be used to modify photocatalysts. This is because metal nodes and organic linkers of MOFs can be easily modified to improve photon absorption and catalytic activity. Therefore, various modification strategies have been devised, such as double substrate metal-organic framework, metal nanoparticles and MOF composite, etc (Wang Z. et al., 2020; Duan et al., 2020; Younis et al., 2020; Fang et al., 2021). The results showed that the activity of H2O2 synthesis was improved by modification of ZIF (Chang et al., 2020) and MIL (Isaka et al., 2019) type metal-organic framework materials. It was mainly attributed to the wider bandgap energy. Titanium-zirconium MOFs were prepared and used for photocatalytic production of H2O2 in two phase system (water/benzoic acid). Ti species effectively promoted electron transfer from the photoexcited linkers of MOFs to Ti and inhibited the recombination of electron-hole pairs in the hydrophobic MOFs matrix (Chen et al., 2020c). Table 2 summarizes the effects of different surface modifications on hydrogen peroxide production activity.
4 Effect of doping on the photocatalytic activity
Doping elements can effectively reduce the band gap of photocatalysts to improve the utilization of solar light (Akpan and Hameed, 2010; Zhao et al., 2017). Studies have shown that doping can change the number of active sites, reduce the formation energy of •OOH intermediates, and promote the formation of H2O2 (Li X. et al., 2021). Therefore, incorporating metal and non-metal ions in the photocatalyst can improve the photocatalytic synthesis activity of H2O2 (Table 3).
4.1 Metal ion incorporation
Incorporating metal ions in the photocatalyst can improve the photocatalytic synthesis activity of H2O2 (Wu et al., 2017; Kim S. et al., 2018; Qu et al., 2018; Feng et al., 2020; Hu et al., 2020). For example, incorporating g-C3N4 with K+ can be used to photocatalyze water decomposition to produce H2 and H2O2 simultaneously without any sacrificial agent (Figure 5A). K+ was coordinated into the big C-N rings by forming the N-bridge, which inhibits the crystal growth of g-C3N4, promotes the specific surface area, increases the visible light absorption. More importantly, the CB and VB can be adjusted to the best position (Figure 5B) (Hu et al., 2020). A similar phenomenon of band gap adjustment was observed in g-C3N4 co-incorporated with K+and Na+. After the band gap adjustment, not only CB electrons can reduce O2 to produce H2O2, but also VB holes can oxidize OH− to •OH for H2O2 synthesis. This made the generation mechanism of photocatalytic H2O2 change from “single channel pathway” (O2 + 2e− + 2H+ → H2O2) to “dual channel pathway” (O2 + 2e− + 2H+ → H2O2 and •OH+•OH→H2O2 reaction pathways) (Qu et al., 2018).
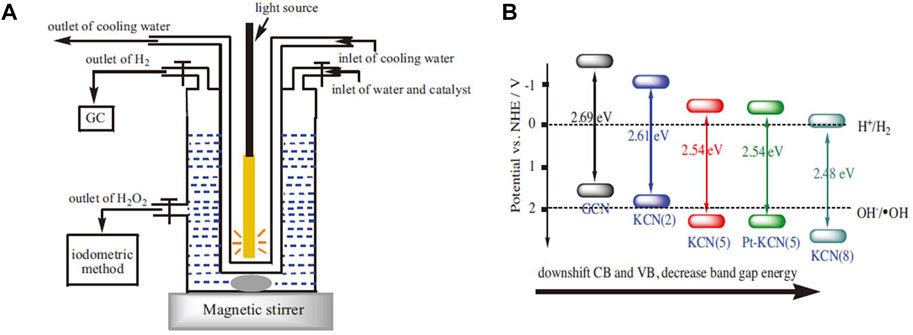
FIGURE 5. (A) The schematic diagram of the reactor. (B) The band position of K+/g-C3N4 (Hu et al., 2020).
4.2 Non-metal ion doping
Non-metal ion doping photocatalyst can effectively improve the synthetic activity of H2O2 (Moon et al., 2014; Wei et al., 2018; Zhang et al., 2018; Wang L. C. et al., 2019; Ye et al., 2021). For example, halogens (Cl and Br) were incorporated into g-C3N4 by hydrothermal method (Figure 6A), and it was found that g-C3N4 incorporated with Br was more conducive to H2O2 synthesis. This is mainly due to the larger specific surface area and higher charge separation rate after incorporating (Figure 6B) (Zhang et al., 2018). A similar phenomenon was observed in the co-doping of metal ions and non-metals (K and P) (Figure 6C). Compared with g-C3N4 incorporated with P (NH4H2PO4/GCN) or K+ (K2SO4/GCN), the H2O2 generation of g-C3N4 after co-incorporating was 10.98 times of the former and 5.2 times of the latter, respectively (Figure 6D) (Tian et al., 2019). Similarly, co-doping can also improve the catalytic activity of TiO2. For example, Fe and S were co-doped into TiO2 by one-step anodic oxidation, and it was found that the synthesis activity of TiO2 was improved after doping. This is mainly attributed to the fact that Fe-S co doped TiO2 had a narrower band gap than pure TiO2, resulting in a wider visible light absorption range (Momeni and Akbarnia, 2021).
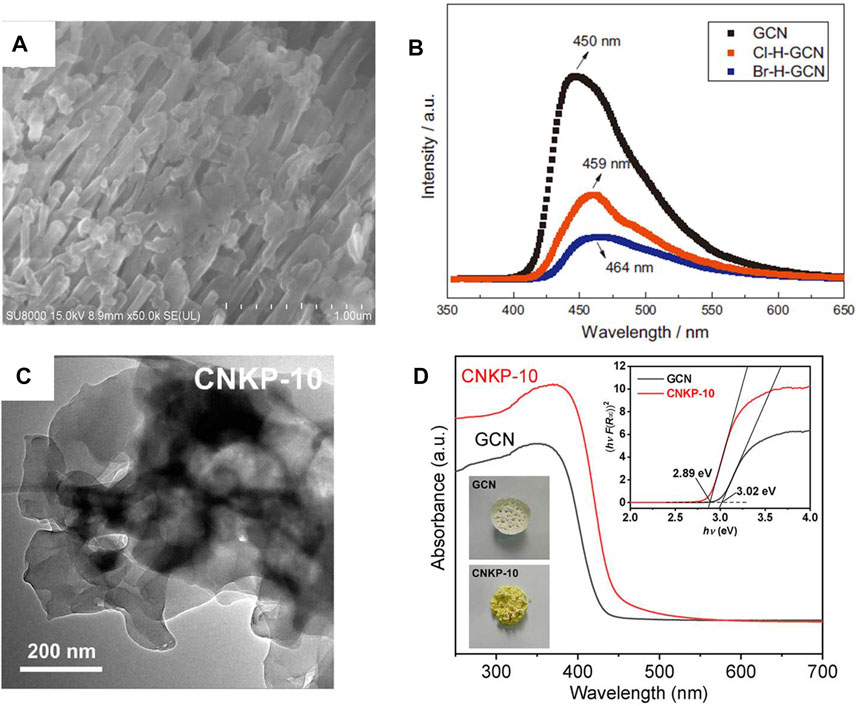
FIGURE 6. (A) The SEM images of Br-H-GCN. (B) PL spectra of GCN, Cl-H-GCN and Br-H-GCN (Zhang et al., 2018). (C) TEM images of the CNKP-10 catalysts. (D) UV–vis DRS spectra of the GCN and CNKP-10 catalysts (Tian et al., 2019).
5 Effect of reaction environment on photocatalytic activity
5.1 Effects of temperature and pH
One study investigated the effect of temperature and pH on the photoactivity of H2O2 generation by using Au/TiO2 photocatalyst. The results showed that when pH value (pH = 2) or temperature (5°C) was low, it was more beneficial to improve the photoactivity. The main reason was that the thermal catalytic decomposition of H2O2 by Au/TiO2 can be effectively inhibited at low pH value or low temperature (Teranishi et al., 2016). In another study, it was found that low pH also increased the H2O2 synthesis activity of MOFs materials. At the same temperature, when the pH value of MOFs material was as low as 0.3, the formation of H2O2 was more favorable. (Isaka et al., 2019).
5.2 Effects of sacrificial agents
For the photocatalytic production of H2O2, a certain amount of sacrificial agent is usually added to act as hole scavenger and prevent the recombination of electron–hole pairs. The sacrificial agents were mainly alcohols, which provided hydrogen source for photocatalytic H2O2 generation (Kormann et al.). However, the ability of aliphatic alcohols (such as ethanol and methanol, which act as electron donors) to improve photoactivity is limited. The results showed that g-C3N4 can effectively synthesize H2O2 in deionized water containing oxygen under visible light irradiation. This was due to the efficient formation of 1, 4-endoperoxide on the surface of g-C3N4. The addition of ethanol inhibited the one-electron reduction of O2 (formation of superoxide radicals) and selectively promoted the two-electron reduction of O2. At the same time, the photodecomposition of hydrogen peroxide formed subsequently was inhibited (Shiraishi et al., 2014b). Kim et al, (2016) also investigated whether the addition of electron donors affects photocatalytic activity. The results showed that when no sacrificial agent (methanol) was added to the system, the generation activity of H2O2 was extremely low. This result confirmed that using sacrificial agents such as methanol is important. When methanol (5 vol%) was present in the system, H2O2 was generated together with formaldehyde (CH3OH + O2 → HCHO + H2O2). Meanwhile, ethanol and 2-propanol were tested further, and the results showed that these alcohols worked effectively as electron donors.
However, aliphatic alcohols (such as ethanol and methanol) as electron donors have limited improvement in photocatalytic activity for hydrogen peroxide synthesis. Therefore, some studies have tried to use aromatic alcohol (benzyl alcohol) as a sacrifice agent, compared with fatty alcohol. The results showed that, during photoreaction with aliphatic alcohol, the carbon radical was rapidly removed and leaved superoxo radical (the f→i process in Figure 7), resulting in very low for H2O2 formation. In the aqueous phase containing benzyl alcohol, the carbon free radical was stably transformed into an oxygen bridge complex (f→g→h process in Figure 7), which generates a large number of peroxides and improves the synthesis activity of H2O2. The results showed that benzyl alcohol as electron donor can improve the reactivity. (Shiraishi et al., 2013).
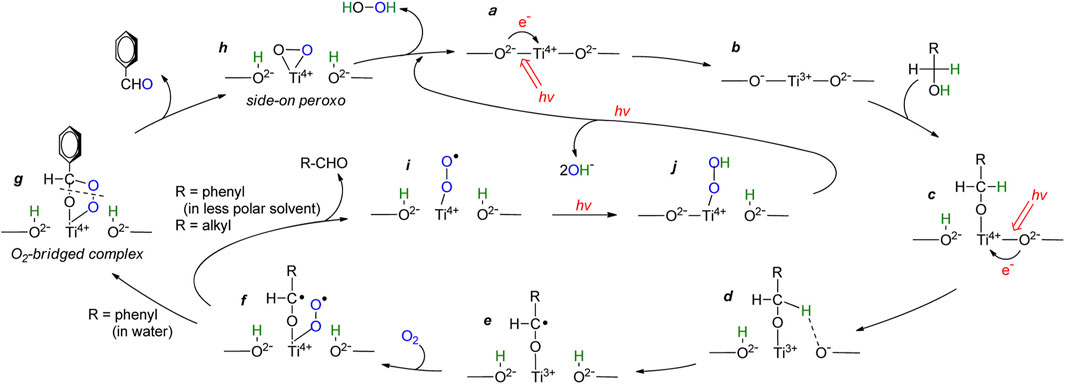
FIGURE 7. Proposed mechanism for photocatalytic oxidation of alcohols with O2 on the TiO2 Surface (Shiraishi et al., 2013).
Conclusions and outlook
In future, the photocatalytic H2O2 synthesis system still need to improve the reaction activity and sustainability. We should consistently increase the upper limit of H2O2 production concentration in long-term photocatalytic reaction. The kinetics of photocatalytic H2O2 decomposition should be especially concerned. It is also urgent to develop efficient non-precious cocatalysts with two-electron ORR selectivity.
The activity of H2O2 synthesis was very unsatisfactory in most pure water systems. As a compromise for adding sacrificial reagents, the photocatalytic H2O2 synthesis system could be coupled with other valuable photocatalytic selective oxidation reaction to maximize its value, such as coupling with selective oxidation or photocatalytic degradation reactions.
Author contributions
Conceptualization, XM, XX, and JZ; writing—original draft preparation, HW, and SH; writing—review and editing, XM and VR; funding acquisition, XM. HW and SH contributed equally to this work. All authors have read and agreed to the published version of the manuscript.
Funding
This work received financial support from the National Natural Science Foundation of China (51872091), the Natural Science Foundation of Hebei Province (H2022209089), Basic Scientific Research Expenses of Universities in Hebei Province (JYG2021003 and JYG 2022001), and Tangshan Talent Funding Project (A202202007).
Conflict of interest
The authors declare that the research was conducted in the absence of any commercial or financial relationships that could be construed as a potential conflict of interest.
Publisher’s note
All claims expressed in this article are solely those of the authors and do not necessarily represent those of their affiliated organizations, or those of the publisher, the editors and the reviewers. Any product that may be evaluated in this article, or claim that may be made by its manufacturer, is not guaranteed or endorsed by the publisher.
References
Adams, J. S., Kromer, M. L., Rodriguez-Lopez, J., and Flaherty, D. W. (2021). Unifying concepts in electro- and thermocatalysis toward hydrogen peroxide production. J. Am. Chem. Soc. 143, 7940–7957. doi:10.1021/jacs.0c13399
Akpan, U. G., and Hameed, B. H. (2010). The advancements in sol–gel method of doped-TiO2 photocatalysts. Appl. Catal. A General 375, 1–11. doi:10.1016/j.apcata.2009.12.023
Anantharaj, S., Pitchaimuthu, S., and Noda, S. (2021). A review on recent developments in electrochemical hydrogen peroxide synthesis with a critical assessment of perspectives and strategies. Adv. Colloid Interface Sci. 287, 102331. doi:10.1016/j.cis.2020.102331
Andersen, B. M., Rasch, M., Hochlin, K., Jensen, F. H., Wismar, P., and Fredriksen, J. E. (2006). Decontamination of rooms, medical equipment and ambulances using an aerosol of hydrogen peroxide disinfectant. J. Hosp. Infect. 62, 149–155. doi:10.1016/j.jhin.2005.07.020
Apaydin, D. H., Seelajaroen, H., Pengsakul, O., Thamyongkit, P., Sariciftci, N. S., Kunze-Liebhauser, J., et al. (2018). Photoelectrocatalytic synthesis of hydrogen peroxide by molecular copper-porphyrin supported on titanium dioxide nanotubes. ChemCatChem 10, 1793–1797. doi:10.1002/cctc.201702055
Awa, K., Naya, S.-I., Fujishima, M., and Tada, H. (2020). A three-component plasmonic photocatalyst consisting of gold nanoparticle and TiO2–SnO2 nanohybrid with heteroepitaxial junction: Hydrogen peroxide synthesis. J. Phys. Chem. C 124, 7797–7802. doi:10.1021/acs.jpcc.9b11875
Baran, T., Wojtyla, S., Vertova, A., Minguzzi, A., and Rondinini, S. (2018). Photoelectrochemical and photocatalytic systems based on titanates for hydrogen peroxide formation. J. Electroanal. Chem. 808, 395–402. doi:10.1016/j.jelechem.2017.06.044
Cai, J., Huang, J., Wang, S., Iocozzia, J., Sun, Z., Sun, J., et al. (2019). Crafting mussel-inspired metal nanoparticle-decorated ultrathin graphitic carbon nitride for the degradation of chemical pollutants and production of chemical resources. Adv. Mater 31, e1806314. doi:10.1002/adma.201806314
Campos-Martin, J. M., Blanco-Brieva, G., and Fierro, J. L. (2006). Hydrogen peroxide synthesis: An outlook beyond the anthraquinone process. Angew. Chem. Int. Ed. Engl. 45, 6962–6984. doi:10.1002/anie.200503779
Chang, A. L., Nguyen, V. H., Lin, K. Y. A., and Hu, C. C. (2020). Selective synthesis of ZIFs from zinc and nickel nitrate solution for photocatalytic H2O2 production. Arabian J. Chem. 13, 8301–8308. doi:10.1016/j.arabjc.2020.04.027
Chang, S. L., Li, H., Liu, J. N., Zhao, M. X., Tan, M. H., Xu, P. W., et al. (2021). Effect of hydrogen peroxide treatment on the quality of epsilon-poly-L-lysine products. Biochem. Eng. J. 171, 108017. doi:10.1016/j.bej.2021.108017
Chang, X. Y., Yang, J. J., Han, D. D., Zhang, B., Xiang, X., and He, J. (2018). Enhancing light-driven production of hydrogen peroxide by anchoring Au onto C3N4 catalysts. Catalysts 8, 147. doi:10.3390/catal8040147
Chen, J. Y., Cao, J. M., Zhou, J., Wang, W. Q., Zhang, Y. F., Liu, J. F., et al. (2020a). A computational evaluation of MoS2-based materials for the electrocatalytic oxygen reduction reaction. New J. Chem. 44, 14189–14197. doi:10.1039/d0nj02621b
Chen, Q. (2008). Development of an anthraquinone process for the production of hydrogen peroxide in a trickle bed reactor—from bench scale to industrial scale. Chem. Eng. Process. Process Intensif. 47, 787–792. doi:10.1016/j.cep.2006.12.012
Chen, S., Tu, R., Li, J., and Lu, X. (2018). Pd catalysts supported on rGO-TiO2 composites for direct synthesis of H2O2 : Modification of Pd2+/Pd0 ratio and hydrophilic property. Chin. J. Chem. Eng. 26, 534–539. doi:10.1016/j.cjche.2017.07.016
Chen, X., Hu, S. Z., Li, P., Li, W., Ma, H. F., and Lu, G. (2017). Photocatalytic production of hydrogen peroxide using g-C3N4 coated MgO-Al2O3-Fe2O3 heterojunction catalysts prepared by a novel molten salt-assisted microwave process. Acta Physico-Chimica Sin. 33, 2532–2541. doi:10.3866/pku.Whxb201706153
Chen, X. L., Kuwahara, Y., Mori, K., Louis, C., and Yamashita, H. (2020b). A hydrophobic titanium doped zirconium-based metal organic framework for photocatalytic hydrogen peroxide production in a two-phase system. J. Mater. Chem. A 8, 1904–1910. doi:10.1039/c9ta11120d
Chen, X., Zhang, W., Zhang, L., Feng, L., Zhang, C., Jiang, J., et al. (2020). Sacrificial agent-free photocatalytic H2O2 evolutionviatwo-electron oxygen reduction using a ternary α-Fe2O3/CQD@g-C3N4 photocatalyst with broad-spectrum response. J. Mater. Chem. A 8, 18816–18825. doi:10.1039/d0ta05753c
Chen, Y. M., Gu, W. Q., Tan, L., Ao, Z. M., An, T. C., and Wang, S. B. (2021). Photocatalytic H2O2 production using Ti3C2 MXene as a non-noble metal cocatalyst. Appl. Catal. a-General 618, 118127. doi:10.1016/j.apcata.2021.118127
Chen, Z., Li, C., Ni, Y., Kong, F., Kong, A., and Shan, Y. (2017). Ag-Enhanced catalytic performance of ordered mesoporous Fe–N-graphitic carbons for oxygen electroreduction. Catal. Lett. 147, 2745–2754. doi:10.1007/s10562-017-2186-2
Chung, S., Chung, J., and Chung, C. (2020). Enhanced electrochemical oxidation process with hydrogen peroxide pretreatment for removal of high strength ammonia from semiconductor wastewater. J. Water Process Eng. 37, 101425. doi:10.1016/j.jwpe.2020.101425
Dinakar, M., Tao, W. D., and Daley, D. (2020). Using hydrogen peroxide to supplement oxygen for nitrogen removal in constructed wetlands. J. Environ. Chem. Eng. 8, 104517. doi:10.1016/j.jece.2020.104517
Du, L., Zhang, G. X., Liu, X. H., Hassanpour, A., Dubois, M., Tavares, A. C., et al. (2020). Biomass-derived nonprecious metal catalysts for oxygen reduction reaction: The demand-oriented engineering of active sites and structures. Carbon Energy 2, 561–581. doi:10.1002/cey2.73
Duan, M. B., Jiang, L. B., Zeng, G. M., Wang, D. B., Tang, W. W., Liang, J., et al. (2020). Bimetallic nanoparticles/metal-organic frameworks: Synthesis, applications and challenges. Appl. Mater. Today 19, 100564. doi:10.1016/j.apmt.2020.100564
Edwards, J. K., and Hutchings, G. J. (2008). Palladium and gold-palladium catalysts for the direct synthesis of hydrogen peroxide. Angew. Chem. Int. Ed. Engl. 47, 9192–9198. doi:10.1002/anie.200802818
Fang, Y., Yang, Y., Yang, Z. G., Li, H. P., and Roesky, H. W. (2021). Advances in design of metal-organic frameworks activating persulfate for water decontamination. J. Organomet. Chem. 954, 122070. doi:10.1016/j.jorganchem.2021.122070
Feng, C. Y., Tang, L., Deng, Y. C., Wang, J. J., Luo, J., Liu, Y. N., et al. (2020). Synthesis of leaf-vein-like g-C(3)N(4)with tunable band structures and charge transfer properties for selective photocatalytic H(2)O(2)Evolution. Adv. Funct. Mater. 30, 2001922. doi:10.1002/adfm.202001922
Feng, L. W., Li, B. D., Xiao, Y. Q., Li, L. J., Zhang, Y. Q., Zhao, Q. N., et al. (2021). Au modified Bi2O3-TiO2 hybrid for photocatalytic synthesis of hydrogen peroxide. Catal. Commun. 155, 106315. doi:10.1016/j.catcom.2021.106315
Fukuzumi, S., Lee, Y. M., and Nam, W. (2018). Solar-Driven production of hydrogen peroxide from water and dioxygen. Chemistry 24, 5016–5031. doi:10.1002/chem.201704512
Gao, G. H., Tian, Y. N., Gong, X. X., Pan, Z. Y., Yang, K. Y., and Zong, B. N. (2020). Advances in the production technology of hydrogen peroxide. Chin. J. Catal. 41, 1039–1047. doi:10.1016/S1872-2067(20)63562-8
Goclon, J., and Winkler, K. (2018). Computational insight into the mechanism of O2 to H2O2 reduction on amino-groups-containing g-C3N4. Appl. Surf. Sci. 462, 134–141. doi:10.1016/j.apsusc.2018.08.070
Guarino, V. A., Oldham, W. M., Loscalzo, J., and Zhang, Y. Y. (2019). Reaction rate of pyruvate and hydrogen peroxide: Assessing antioxidant capacity of pyruvate under biological conditions. Sci. Rep. 9, 19568. doi:10.1038/s41598-019-55951-9
Guo, F., Zhang, M., Yi, S., Li, X., Xin, R., Yang, M., et al. (2022). Metal-coordinated porous polydopamine nanospheres derived Fe3N-FeCo encapsulated N-doped carbon as a highly efficient electrocatalyst for oxygen reduction reaction. Nano Res. Energy 1, e9120027. doi:10.26599/NRE.2022.9120027
Guo, Y., Li, H. R., Ma, W., Shi, W. X., Zhu, Y. F., and Choi, W. Y. (2020). Photocatalytic activity enhanced via surface hybridization. Carbon Energy 2, 308–349. doi:10.1002/cey2.66
Haider, Z., Cho, H. I., Moon, G. H., and Kim, H. I. (2019). Minireview: Selective production of hydrogen peroxide as a clean oxidant over structurally tailored carbon nitride photocatalysts. Catal. Today 335, 55–64. doi:10.1016/j.cattod.2018.11.067
Halder, R., and Lawal, A. (2007). Experimental studies on hydrogenation of anthraquinone derivative in a microreactor. Catal. Today 125, 48–55. doi:10.1016/j.cattod.2007.03.055
Han, Y., He, Z. Y., Wang, S. L., Li, W., and Zhang, J. L. (2015). Performance of facet-controlled Pd nanocrystals in 2-ethylanthraquinone hydrogenation. Catal. Sci. Technol. 5, 2630–2639. doi:10.1039/c5cy00050e
Hirakawa, H., Shiota, S., Shiraishi, Y., Sakamoto, H., Ichikawa, S., and Hirai, T. (2016). Au nanoparticles supported on BiVO4: Effective inorganic photocatalysts for H2O2 production from water and O2 under visible light. ACS Catal. 6, 4976–4982. doi:10.1021/acscatal.6b01187
Hu, S., Sun, X., Zhao, Y., Li, W., Wang, H., and Wu, G. (2020). The effective photocatalytic water splitting to simultaneously produce H2 and H2O2 over Pt loaded K-g-C3N4 catalyst. J. Taiwan Inst. Chem. Eng. 107, 129–138. doi:10.1016/j.jtice.2019.12.007
Ignaczak, A., Santos, E., and Schmickler, W. (2019). Oxygen reduction reaction on gold in alkaline solutions - the inner or outer sphere mechanisms in the light of recent achievements. Curr. Opin. Electrochem. 14, 180–185. doi:10.1016/j.coelec.2018.07.011
Isaka, Y., Kawase, Y., Kuwahara, Y., Mori, K., and Yamashita, H. (2019). Two-phase system utilizing hydrophobic metal-organic frameworks (MOFs) for photocatalytic synthesis of hydrogen peroxide. Angew. Chem. Int. Ed. Engl. 58, 5402–5406. doi:10.1002/anie.201901961
Jeon, T. Y., Yu, S. H., Yoo, S. J., Park, H. Y., and Kim, S. K. (2020). Electrochemical determination of the degree of atomic surface roughness in Pt–Ni alloy nanocatalysts for oxygen reduction reaction. Carbon Energy 3, 375–383. doi:10.1002/cey2.82
Jia, X. W., Sun, F., Fei, Y., Jin, M. P., Zhang, F., Xu, W., et al. (2018). Explosion characteristics of mixtures containing hydrogen peroxide and working solution in the anthraquinone route to hydrogen peroxide. Process Saf. Environ. Prot. 119, 218–222. doi:10.1016/j.psep.2018.08.007
Jirkovsky, J. S., Halasa, M., and Schiffrin, D. J. (2010). Kinetics of electrocatalytic reduction of oxygen and hydrogen peroxide on dispersed gold nanoparticles. Phys. Chem. Chem. Phys. 12, 8042–8052. doi:10.1039/c002416c
Jirkovsky, J. S., Panas, I., Ahlberg, E., Halasa, M., Romani, S., and Schiffrin, D. J. (2011). Single atom hot-spots at Au-Pd nanoalloys for electrocatalytic H2O2 production. J. Am. Chem. Soc. 133, 19432–19441. doi:10.1021/ja206477z
Kim, H. I., Choi, Y., Hu, S., Choi, W., and Kim, J. H. (2018b). Photocatalytic hydrogen peroxide production by anthraquinone-augmented polymeric carbon nitride. Appl. Catal. B-Environmental 229, 121–129. doi:10.1016/j.apcatb.2018.01.060
Kim, H. I., Kwon, O. S., Kim, S., Choi, W., and Kim, J. H. (2016). Harnessing low energy photons (635 nm) for the production of H2O2 using upconversion nanohybrid photocatalysts. Energy & Environ. Sci. 9, 1063–1073. doi:10.1039/c5ee03115j
Kim, J., Kim, H. E., and Lee, H. (2018a). Single-atom catalysts of precious metals for electrochemical reactions. ChemSusChem 11, 104–113. doi:10.1002/cssc.201701306
Kim, S., Moon, G. H., Kim, H., Mun, Y., Zhang, P., Lee, J., et al. (2018c). Selective charge transfer to dioxygen on KPF6-modified carbon nitride for photocatalytic synthesis of H2O2 under visible light. J. Catal. 357, 51–58. doi:10.1016/j.jcat.2017.10.002
Kofuji, Y., Ohkita, S., Shiraishi, Y., Sakamoto, H., Tanaka, S., Ichikawa, S., et al. (2016). Graphitic carbon nitride doped with biphenyl diimide: Efficient photocatalyst for hydrogen peroxide production from water and molecular oxygen by sunlight. Acs Catal. 6, 7021–7029. doi:10.1021/acscatal.6b02367
Kormann, C., Bahnemann, D. W., and Hoffmann, M. R. (1988). Photocatalytic production of hydrogen peroxides and organic peroxides in aqueous suspensions of titanium dioxide, zinc oxide, and desert sand. Environ. Sci. Technol. 22, 798–806. doi:10.1021/es00172a009
Kozlova, L. S., Novikov, V. T., Garaeva, G. R., Gol'din, M. M., and Kolesnikov, V. A. (2015). Electrodes modified with carbon materials in electrosynthesis of the dissolved hydrogen peroxide solutions and their medical properties. Prot. Metals Phys. Chem. Surfaces 51, 985–989. doi:10.1134/S2070205115060131
Li, L., Li, B., Feng, L., Zhang, X., Zhang, Y., Zhao, Q., et al. (2021a). Au modified F-TiO2 for efficient photocatalytic synthesis of hydrogen peroxide. Molecules 26, 3844. doi:10.3390/molecules26133844
Li, S. N., Dong, G. H., Hailili, R., Yang, L. P., Li, Y. X., Wang, F., et al. (2016). Effective photocatalytic H2O2 production under visible light irradiation at g-C3N4 modulated by carbon vacancies. Appl. Catal. B-Environmental 190, 26–35. doi:10.1016/j.apcatb.2016.03.004
Li, X., Wang, X., Xiao, G., and Zhu, Y. (2021b). Identifying active sites of boron, nitrogen co-doped carbon materials for the oxygen reduction reaction to hydrogen peroxide. J. Colloid Interface Sci. 602, 799–809. doi:10.1016/j.jcis.2021.06.068
Li, X. Z., Chen, C. C., and Zhao, J. C. (2001). Mechanism of photodecomposition of H2O2 on TiO2 surfaces under visible light irradiation. Langmuir 17, 4118–4122. doi:10.1021/la010035s
Liu, B., Bie, C., Zhang, Y., Wang, L., Li, Y., and Yu, J. (2021). Hierarchically porous ZnO/g-C3N4 S-scheme heterojunction photocatalyst for efficient H2O2 production. Langmuir 37, 14114–14124. doi:10.1021/acs.langmuir.1c02360
Liu, Y. M., Roy, S., Sarkar, S., Xu, J. Q., Zhao, Y. F., and Zhang, J. J. (2021). A review of carbon dots and their composite materials for electrochemical energy technologies. Carbon Energy 3, 795–826. doi:10.1002/cey2.134
Ma, R. Y., Wang, L., Wang, H., Liu, Z. Y., Xing, M. Y., Zhu, L. F., et al. (2019). Solid acids accelerate the photocatalytic hydrogen peroxide synthesis over a hybrid catalyst of titania nanotube with carbon dot. Appl. Catal. B-Environmental 244, 594–603. doi:10.1016/j.apcatb.2018.11.087
Maurino, V., Minero, C., Mariella, G., and Pelizzetti, E. (2005). Sustained production of H2O2 on irradiated TiO2-fluoride systems. Chem. Commun. (Camb), 2627–2629. doi:10.1039/b418789j
Meng, X. G., Zong, P. X., Wang, L., Yang, F., Hou, W. S., Zhang, S. T., et al. (2020). Au-nanoparticle-supported ZnO as highly efficient photocatalyst for H2O2 production. Catal. Commun. 134, 105860. doi:10.1016/j.catcom.2019.105860
Momeni, M. M., and Akbarnia, M. (2021). Photoelectrochemical, photocatalytic and electrochemical hydrogen peroxide production using Fe/S-codoped TiO2 nanotubes as new visible-light-absorbing photocatalysts. Appl. Phys. A 127, 449. doi:10.1007/s00339-021-04574-x
Moon, G.-H., Fujitsuka, M., Kim, S., Majima, T., Wang, X., and Choi, W. (2017). Eco-friendly photochemical production of H2O2 through O2 reduction over carbon nitride frameworks incorporated with multiple heteroelements. ACS Catal. 7, 2886–2895. doi:10.1021/acscatal.6b03334
Moon, G.-H., Kim, W., Bokare, A. D., Sung, N.-E., and Choi, W. (2014). Solar production of H2O2 on reduced graphene oxide–TiO2 hybrid photocatalysts consisting of earth-abundant elements only. Energy Environ. Sci. 7, 4023–4028. doi:10.1039/c4ee02757d
Moreno, C. M. (2011). Hydrogen peroxide production driven by UV-B in planktonic microorganisms: A photocatalytic factor in sea warming and ice melting in regions with ozone depletion? Biogeochemistry 107, 1–8. doi:10.1007/s10533-010-9566-7
Noh, J. H., Yoo, S. H., Son, H., Fish, K. E., Douterelo, I., and Maeng, S. K. (2020). Effects of phosphate and hydrogen peroxide on the performance of a biological activated carbon filter for enhanced biofiltration. J. Hazard Mater 388, 121778. doi:10.1016/j.jhazmat.2019.121778
Peng, Y. L., Wang, L. Z., Liu, Y. D., Chen, H. J., Lei, J. Y., and Zhang, J. L. (2017). Visible-light-Driven photocatalytic H2O2 production on g-C3N4 loaded with CoP as a noble metal free cocatalyst. Eur. J. Inorg. Chem. 2017, 4797–4802. doi:10.1002/ejic.201700930
Qu, X., Hu, S., Bai, J., Li, P., Lu, G., and Kang, X. (2018). Synthesis of band gap-tunable alkali metal modified graphitic carbon nitride with outstanding photocatalytic H2O2 production ability via molten salt method. J. Mater. Sci. Technol. 34, 1932–1938. doi:10.1016/j.jmst.2018.04.019
Shiraishi, Y., Kanazawa, S., Kofuji, Y., Sakamoto, H., Ichikawa, S., Tanaka, S., et al. (2014a). Sunlight-driven hydrogen peroxide production from water and molecular oxygen by metal-free photocatalysts. Angew. Chem. Int. Ed. Engl. 53, 13454–13459. doi:10.1002/anie.201407938
Shiraishi, Y., Kanazawa, S., Sugano, Y., Tsukamoto, D., Sakamoto, H., Ichikawa, S., et al. (2014b). Highly selective production of hydrogen peroxide on graphitic carbon nitride (g-C3N4) photocatalyst activated by visible light. Acs Catal. 4, 774–780. doi:10.1021/cs401208c
Shiraishi, Y., Kanazawa, S., Tsukamoto, D., Shiro, A., Sugano, Y., and Hirai, T. (2013). Selective hydrogen peroxide formation by titanium dioxide photocatalysis with benzylic alcohols and molecular oxygen in water. Acs Catal. 3, 2222–2227. doi:10.1021/cs400511q
Shiraishi, Y., Takii, T., Hagi, T., Mori, S., Kofuji, Y., Kitagawa, Y., et al. (2019). Resorcinol-formaldehyde resins as metal-free semiconductor photocatalysts for solar-to-hydrogen peroxide energy conversion. Nat. Mater 18, 985–993. doi:10.1038/s41563-019-0398-0
Shu, C., Tan, Q., Deng, C., Du, W., Gan, Z., Liu, Y., et al. (2021). Hierarchically mesoporous carbon spheres coated with a single atomic Fe–N–C layer for balancing activity and mass transfer in fuel cells. Carbon Energy 4, 1–11. doi:10.1002/cey2.136
Song, H. Y., Wei, L. S., Chen, C. X., Wen, C. C., and Han, F. Q. (2019). Photocatalytic production of H2O2 and its in situ utilization over atomic-scale Au modified MoS2 nanosheets. J. Catal. 376, 198–208. doi:10.1016/j.jcat.2019.06.015
Sterenchuk, T. P., Belykh, L. B., Skripov, N. I., Sanzhieva, S. B., Gvozdovskaya, K. L., and Schmidt, F. K. (2018). The effect of particle size and the modifier on the properties of palladium catalysts in the synthesis of hydrogen peroxide by the anthraquinone method. Kinet. Catal. 59, 585–592. doi:10.1134/S0023158418050166
Sun, Z., Sheng, L., Gong, H., Song, L., Jiang, X., Wang, S., et al. (2020). Electrocatalytic synthesis of hydrogen peroxide over Au/TiO2 and electrochemical trace of OOH* intermediate. Chem. Asian J. 15, 4280–4285. doi:10.1002/asia.202001089
Teng, Z. Y., Cai, W., Liu, S. X., Wang, C. Y., Zhang, Q. T., Su, C. L., et al. (2020). Bandgap engineering of polymetric carbon nitride copolymerized by 2, 5, 8-triamino-tri-s-triazine (melem) and barbituric acid for efficient nonsacrificial photocatalytic H2O2 production. Appl. Catal. B-Environmental 271, 118917. doi:10.1016/j.apcatb.2020.118917
Teranishi, M., Kunimoto, T., Naya, S., Kobayashi, H., and Tada, H. (2020). Visible-light-Driven hydrogen peroxide synthesis by a hybrid photocatalyst consisting of bismuth vanadate and bis(hexafluoroacetylacetonato)copper(II) complex. J. Phys. Chem. C 124, 3715–3721. doi:10.1021/acs.jpcc.9b11568
Teranishi, M., Naya, S., and Tada, H. (2010). In situ liquid phase synthesis of hydrogen peroxide from molecular oxygen using gold nanoparticle-loaded titanium(IV) dioxide photocatalyst. J. Am. Chem. Soc. 132, 7850–7851. doi:10.1021/ja102651g
Teranishi, M., Naya, S., and Tada, H. (2016). Temperature- and pH-dependence of hydrogen peroxide formation from molecular oxygen by gold nanoparticle-loaded titanium(IV) oxide photocatalyst. J. Phys. Chem. C 120, 1083–1088. doi:10.1021/acs.jpcc.5b10626
Tian, J., Wu, T. J., Wang, D., Pei, Y., Qiao, M. H., and Zong, B. N. (2019). One-pot synthesis of potassium and phosphorus-doped carbon nitride catalyst derived from urea for highly efficient visible light-driven hydrogen peroxide production. Catal. Today 330, 171–178. doi:10.1016/j.cattod.2018.07.039
Tsukamoto, D., Shiro, A., Shiraishi, Y., Sugano, Y., Ichikawa, S., Tanaka, S., et al. (2012). Photocatalytic H2O2 production from ethanol/O2 system using TiO2 loaded with Au–Ag bimetallic alloy nanoparticles. ACS Catal. 2, 599–603. doi:10.1021/cs2006873
Viswanathan, V., Hansen, H. A., Rossmeisl, J., and Norskov, J. K. (2012). Unifying the 2e(-) and 4e(-) Reduction of Oxygen on Metal Surfaces. J. Phys. Chem. Lett. 3, 2948–2951. doi:10.1021/jz301476w
Wang, H., Guan, Y., Hu, S., Pei, Y., Ma, W., and Fan, Z. (2019b). Hydrothermal synthesis of band gap-tunable oxygen-doped g-C3N4 with outstanding “two-channel” photocatalytic H2O2 production ability assisted by dissolution–precipitation process. Nano 14, 1950023. doi:10.1142/s1793292019500231
Wang, L. C., Cao, S., Guo, K., Wu, Z. J., Ma, Z., and Piao, L. Y. (2019a). Simultaneous hydrogen and peroxide production by photocatalytic water splitting. Chin. J. Catal., 40, 470–475. doi:10.1016/S1872-2067(19)63274-2
Wang, Y., Shi, L., Zhu, J., Li, B., and Jin, Y. (2020a). Visual and sensitive detection of telomerase activity via hydrogen peroxide test strip. Biosens. Bioelectron. 156, 112132. doi:10.1016/j.bios.2020.112132
Wang, Z., Huang, J., Mao, J., Guo, Q., Chen, Z., and Lai, Y. (2020b). Metal–organic frameworks and their derivatives with graphene composites: Preparation and applications in electrocatalysis and photocatalysis. J. Mater. Chem. A 8, 2934–2961. doi:10.1039/c9ta12776c
Wei, Z., Liu, M. L., Zhang, Z. J., Yao, W. Q., Tan, H. W., and Zhu, Y. F. (2018). Efficient visible-light-driven selective oxygen reduction to hydrogen peroxide by oxygen-enriched graphitic carbon nitride polymers. Energy & Environ. Sci. 11, 2581–2589. doi:10.1039/c8ee01316k
Willis, D. E., Taheri, M. M., Kizilkaya, O., Leite, T. R., Zhang, L., Ofoegbuna, T., et al. (2020). Critical coupling of visible light extends hot-electron lifetimes for H2O2 synthesis. ACS Appl. Mater Interfaces 12, 22778–22788. doi:10.1021/acsami.0c00825
Wu, G., Hu, S., Han, Z., Liu, C., and Li, Q. (2017). The effect of Ni(i)–N active sites on the photocatalytic H2O2 production ability over nickel doped graphitic carbon nitride nanofibers. New J. Chem. 41, 15289–15297. doi:10.1039/c7nj03298f
Xu, J., Chen, Z., Zhang, H., Lin, G., Lin, H., Wang, X., et al. (2017). Cd3(C3N3S3)2 coordination polymer/graphene nanoarchitectures for enhanced photocatalytic H2O2 production under visible light. Sci. Bull. 62, 610–618. doi:10.1016/j.scib.2017.04.013
Yan, X., Jia, Y., Wang, K., Jin, Z., Dong, C. L., Huang, Y. C., et al. (2020). Controllable synthesis of Fe–N4 species for acidic oxygen reduction. Carbon Energy 2, 452–460. doi:10.1002/cey2.47
Yang, H. (2021). A short review on heterojunction photocatalysts: Carrier transfer behavior and photocatalytic mechanisms. Mater. Res. Bull. 142, 111406. doi:10.1016/j.materresbull.2021.111406
Yang, L. P., Dong, G. H., Jacobs, D. L., Wang, Y. H., Zang, L., and Wang, C. Y. (2017). Two-channel photocatalytic production of H2O2 over g-C3N4 nanosheets modified with perylene imides. J. Catal. 352, 274–281. doi:10.1016/j.jcat.2017.05.010
Yang, S., Verdaguer-Casadevall, A., Arnarson, L., Silvio, L., Colic, V., Frydendal, R., et al. (2018). Toward the decentralized electrochemical production of H2O2: A focus on the catalysis. Acs Catal. 8, 4064–4081. doi:10.1021/acscatal.8b00217
Ye, Y. X., Wen, C., Pan, J. H., Wang, J. W., Tong, Y. J., Wei, S. B., et al. (2021). Visible-light driven efficient overall H2O2 production on modified graphitic carbon nitride under ambient conditions. Appl. Catal. B-Environmental 285, 119726. doi:10.1016/j.apcatb.2020.119726
Younis, S. A., Kwon, E. E., Qasim, M., Kim, K. H., Kim, T., Kukkar, D., et al. (2020). Metal-organic framework as a photocatalyst: Progress in modulation strategies and environmental/energy applications. Prog. Energy Combust. Sci. 81, 100870. doi:10.1016/j.pecs.2020.100870
Zeng, X. K., Liu, Y., Kang, Y., Li, Q. Y., Xia, Y., Zhu, Y. L., et al. (2020). Simultaneously tuning charge separation and oxygen reduction pathway on graphitic carbon nitride by polyethylenimine for boosted photocatalytic hydrogen peroxide production. Acs Catal. 10, 3697–3706. doi:10.1021/acscatal.9b05247
Zeng, X. K., Wang, Z. Y., Wang, G., Gengenbach, T. R., Mccarthy, D. T., Deletic, A., et al. (2017). Highly dispersed TiO2 nanocrystals and WO3 nanorods on reduced graphene oxide: Z-Scheme photocatalysis system for accelerated photocatalytic water disinfection. Appl. Catal. B-Environmental 218, 163–173. doi:10.1016/j.apcatb.2017.06.055
Zhang, C., Bai, J., Ma, L., Lv, Y., Wang, F., Zhang, X., et al. (2018). Synthesis of halogen doped graphite carbon nitride nanorods with outstanding photocatalytic H2O2 production ability via saturated NH4X (X = Cl, Br) solution-hydrothermal post-treatment. Diam. Relat. Mater. 87, 215–222. doi:10.1016/j.diamond.2018.06.013
Zhang, J., Yang, H., Gao, J., Xi, S., Cai, W., Zhang, J., et al. (2020a). Design of hierarchical, three-dimensional free-standing single-atom electrode for H2O2 production in acidic media. Carbon Energy 2, 276–282. doi:10.1002/cey2.33
Zhang, M. M., Lai, C., Li, B. S., Xu, F. H., Huang, D. L., Liu, S. Y., et al. (2020b). Unravelling the role of dual quantum dots cocatalyst in 0D/2D heterojunction photocatalyst for promoting photocatalytic organic pollutant degradation. Chem. Eng. J. 396, 125343. doi:10.1016/j.cej.2020.125343
Zhang, W., Liu, Z., Chen, P., Zhou, G., Liu, Z., and Xu, Y. (2021). Preparation of supported perovskite catalyst to purify membrane concentrate of coal chemical wastewater in UV-catalytic wet hydrogen peroxide oxidation system. Int. J. Environ. Res. Public Health 18, 4906. doi:10.3390/ijerph18094906
Zhao, Z. H., Fan, J. M., Chang, H. H., Asakura, Y., and Yin, S. (2017). Recent progress on mixed-anion type visible-light induced photocatalysts. Sci. China-Technological Sci. 60, 1447–1457. doi:10.1007/s11431-016-9022-9
Zheng, L. H., Su, H. R., Zhang, J. Z., Walekar, L. S., Molamahmood, H. V., Zhou, B. X., et al. (2018). Highly selective photocatalytic production of H2O2 on sulfur and nitrogen for co-doped graphene quantum dots tuned TiO2. Appl. Catal. B-Environmental 239, 475–484. doi:10.1016/j.apcatb.2018.08.031
Zinola, C. F., Triaca, W. E., and Arvia, A. J. (1995). Kinetics and mechanism of the oxygen electroreduction reaction on faceted platinum-electrodes in trifluoromethanesulfonic acid-solutions. J. Appl. Electrochem., 25, 740–754. doi:10.1007/Bf00648629
Zuo, G. F., Li, B. D., Guo, Z. L., Wang, L., Yang, F., Hou, W. S., et al. (2019b). Efficient photocatalytic hydrogen peroxide production over TiO2 passivated by SnO2. Catalysts 9, 623. doi:10.3390/catal9070623
Zuo, G. F., Liu, S. S., Wang, L., Song, H., Zong, P. X., Hou, W. S., et al. (2019a). Finely dispersed Au nanoparticles on graphitic carbon nitride as highly active photocatalyst for hydrogen peroxide production. Catal. Commun. 123, 69–72. doi:10.1016/j.catcom.2019.02.011
Keywords: photocatalysis, oxygen reduction reaction, H2O2 synthesis, cocatalyst, surface modification, ion doping
Citation: Wen H, Huang S, Meng X, Xian X, Zhao J and Roy VAL (2022) Recent progress in the design of photocatalytic H2O2 synthesis system. Front. Chem. 10:1098209. doi: 10.3389/fchem.2022.1098209
Received: 14 November 2022; Accepted: 12 December 2022;
Published: 22 December 2022.
Edited by:
Guigao Liu, Nanjing University of Science and Technology, ChinaReviewed by:
Ning Zhang, Central South University, ChinaHaiying Jiang, Northwest University, China
Shengyao Wang, Huazhong Agricultural University, China
Copyright © 2022 Wen, Huang, Meng, Xian, Zhao and Roy. This is an open-access article distributed under the terms of the Creative Commons Attribution License (CC BY). The use, distribution or reproduction in other forums is permitted, provided the original author(s) and the copyright owner(s) are credited and that the original publication in this journal is cited, in accordance with accepted academic practice. No use, distribution or reproduction is permitted which does not comply with these terms.
*Correspondence: Xianguang Meng, bWVuZ3hnX21hdGVyY2hlbUAxNjMuY29t; Xiaole Xian, eGlhbnhpYW9sZTUyMEAxMjYuY29t; Jingjing Zhao, emhhb2ppbmdqaW5nQG5jc3QuZWR1LmNu