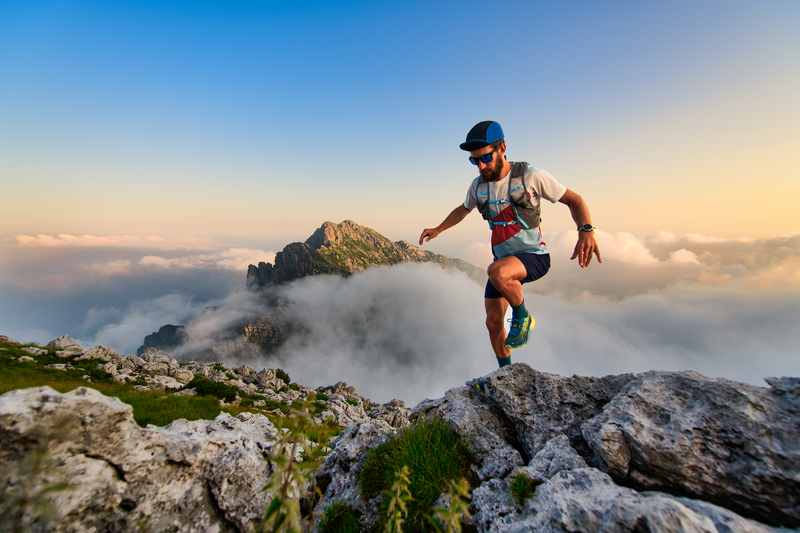
95% of researchers rate our articles as excellent or good
Learn more about the work of our research integrity team to safeguard the quality of each article we publish.
Find out more
REVIEW article
Front. Chem. , 04 January 2023
Sec. Nanoscience
Volume 10 - 2022 | https://doi.org/10.3389/fchem.2022.1095598
This article is part of the Research Topic Recent Advances in the Design of Novel Polymer Nanoagents for Cancer Theranostics View all 10 articles
Chemotherapy is the mainstay of cancer treatment today. Chemotherapeutic drugs are non-selective and can harm both cancer and healthy cells, causing a variety of adverse effects such as lack of specificity, cytotoxicity, short half-life, poor solubility, multidrug resistance, and acquiring cancer stem-like characteristics. There is a paradigm shift in drug delivery systems (DDS) with the advent of smarter ways of targeted cancer treatment. Smart Drug Delivery Systems (SDDSs) are stimuli responsive and can be modified in chemical structure in response to light, pH, redox, magnetic fields, and enzyme degradation can be future of translational medicine. Therefore, SDDSs have the potential to be used as a viable cancer treatment alternative to traditional chemotherapy. This review focuses mostly on stimuli responsive drug delivery, inorganic nanocarriers (Carbon nanotubes, gold nanoparticles, Meso-porous silica nanoparticles, quantum dots etc.), organic nanocarriers (Dendrimers, liposomes, micelles), antibody-drug conjugates (ADC) and small molecule drug conjugates (SMDC) based SDDSs for targeted cancer therapy and strategies of targeted drug delivery systems in cancer cells.
Cancer has emerged as a leading health concern of the 21st century, with over 10 million new patients diagnosed each year (Sung et al., 2021). In 2020, more than 19 million people worldwide were diagnosed with cancer, with nearly 10 million dying as a result (Mao et al., 2022). By 2040, the number of new cases and deaths is expected to be around 28 million and 16 million, respectively (Khazaei et al., 2021; Sung et al., 2021). Currently, surgical resection, radiation therapy (RT), and chemotherapy are the three major treatment modalities of cancer treatment. The comparative usefulness of various procedures are determined on the basis and type of cancer and stage of development. Despite recent advances in treatment strategies and targeted treatment, the survival rate has not improved significantly. As a result, innovative cancer treatment approaches are required. Chemotherapy has been one of the most effective treatments for both localised and metastatic tumours for more than 50 years. The issue of systemic side effects from chemotherapy has yet to be addressed. Conventional drug delivery systems frequently have systemic adverse effects due to non-specific biological distribution and uncontrolled drug release features. Exploration of innovative drug delivery technology can have commercial as well as therapeutic value for health products, is needed to have significant expansion (Liu et al., 2016). Moreover, many drugs are difficult to administer using traditional drug delivery techniques due to a lack of therapeutic effectiveness and a variety of challenges such as limited bioavailability, sensitive toxicity, insufficient specificity, and so on (Majumder and Minko, 2021). Additionally, challenges to be consider and overcome, include the attack of enzymes, the poor permeability of some tissues, and the difficulty of access to the target once arriving at the destination cells, among others (Alvarez-Lorenzo and Concheiro, 2014). There is a need to investigate new innovative ways of drug delivery that can minimize side effects. A drug delivery system (DDS) is a method or process that releases the drug at a pre-selected site in a controlled manner to achieve therapeutic effect. Drug delivery systems can in principle provide enhanced efficacy and/or reduced toxicity for a therapeutic agents. An ideal DDS in cancer achieves two goals: tumor-specific delivery and tumor-specific drug release from delivery systems (Nkepang et al., 2014). Smart Drug Delivery Systems (SDDSs) were developed to circumvent these limitations, allowing payloads to be delivered to target areas in a spatially controlled way. SDDS have many other applications and can be developed into smart systems, encasing therapeutic and imaging agents as well as bearing stealth property. SDDSs can also be used to develop diagnostics tools, PET scanning, MRI-CAs for efficient and early diagnosis of cancer (Wu and Wang, 2016).
Targeted treatments aim to block specific biologic transduction pathways or cancer proteins that are involved in tumour growth and progression, i.e., molecular targets (receptors, kinase cascades, growth factors, or molecules related to angiogenesis and apoptosis) that are found overexpressed or mutated in cancer (Chabner et al., 2005; Hanahan and Weinberg, 2011). The primary objective of these revolutionary therapies is to either block the signals that lead cancer cells to grow and divide uncontrollably, induce apoptosis in cancer cells, stimulate the immune system, or target the delivery of chemotherapy agents specifically to cancer cells, minimising the death of normal cells and avoiding the negative side effects (Perez and Fernandez-Medarde, 2015).
SDDSs can preferentially accumulate and bind to the disease target, allowing for controlled release. It is common knowledge that drugs should be released at target areas in a regulated way to maximise therapeutic effectiveness while minimising negative effects. The loaded medicines can act “smart” by inheriting from the controlled release (Liu et al., 2016).
SDDSs are designed to take advantage of the different conditions (e.g., temperature, pH, and enzyme concentration) that occur in pathological tissues rather than in normal tissues in a “smart” way, enabling them to trigger drug release in the targeted tissue, overcome intermediate barriers, and increase bioavailability, blood circulation time, and overall therapeutic efficacy (AlSawaftah et al., 2021). A better understanding of tumour biology, combined with the increased availability of versatile materials such as polymers, lipids, inorganic carriers, polymeric hydrogels, and bio-macromolecular scaffolds, has led to the development of systems that can deliver chemotherapeutics to tumour sites with improved therapeutic efficacy in recent years (Senapati et al., 2018).
Drug delivery efficiency refers to the safe delivery of a drug to target locations without significant off-target effects (Sanadgol and Wackerlig, 2020). SDDSs are efficient tools to ensure the release of the therapeutic agent at the target and in the right dosage for the needed duration in order to optimise its efficacy by accumulating at the site of action and achieve the therapeutic effective concentration level within the therapeutic window while minimising adverse effects on healthy tissues. This delivery method must be biocompatible and biodegradable in order to penetrate the tissue and cells without causing specific toxicity, immunogenicity, or accumulation in organs other than the tumour. SDDSs have the potential to deliver medicines to precise and targeted locations. The most reported carriers mainly are Liposomes, micelles, dendrimers, meso-porous silica nanoparticles (MSNs), and gold nanoparticles, carbon nanotubes (CNTs), quantum dots (QDs), vitamins (Folic acid (B9) (Rana and Bhatnagar, 2021) and Biotin (B7) (Saha et al., 2013) and monoclonal antibodies (Kimiz-Gebologlu et al., 2018).
In this review article, we highlight the recent development of various SDDSs used in cancer therapeutics to increase the therapeutic index of chemotherapeutic drugs. We highlighted the components and classification of SDDSs, example of target nanocarriers, antibody based smart drug delivery systems, small molecule based smart drug delivery systems. In the context of the current oncological developments, the contribution of fundamental research to clinical practices with respect to SDDSs is explored.
SDDSs have the exciting potential to vastly improve the efficiency and precision of treatment across a wide range of disorders. Smart drug delivery is a means of administering treatment to a patient in a targeted and controlled release manner. SDDSs can efficiently lower dosage frequency while maintaining drug concentrations in certain organs or tissues for a longer period of time when compared to conventional DDSs. In this way, SDDSs offer a wealth of possibilities for lowering drug concentration fluctuations, reducing drug toxicity, and enhancing therapeutic efficacy.
Most anticancer drugs are given at the maximum tolerated dose, cancer patients frequently suffer from severe cytotoxic side effects, limiting their treatment options. SDDSs allow for lower drug doses while maintaining effective intracellular concentrations, therefore expanding the therapeutic window of anticancer drugs. SDDSs have several advantages, including improved specific localization, patient compliance, reduced toxic side effects, and controlled biodistribution (Naziris et al., 2016).
Successful drug delivery requires that drugs should be released at desired target sites in a controlled manner to maximise therapeutic efficacy while minimising side effects. SDDSs, are made up of the following components: carriers/targeting ligand, linker and cytotoxic drug payload. A smart drug delivery system (SDDS) using liposomes as smart carrier (Figure 1), consists of (Liu et al.) Smart Carriers/Targeting Ligands that transport anti-cancer drugs to the targeted cancer site, (ii) targeting mechanisms that locate the cancerous site, and (iii) stimulus techniques that release the payload drugs at the pre-located cancer cell site. The following sections go over the various SDDSs, as well as their targeting mechanisms and stimulus techniques.
The drug target is a crucial part of SDDSs. Commonly explored drug targets in the body: i) Receptors on cell membranes which enable drug carriers to engage specifically with cells, boosting drug absorption via receptor-mediated endocytosis. For example, folate receptors (FRs), which are differentially overexpressed in epithelial cancer cells, are used to deliver tumor-specific drugs in cancers such as breast, ovarian, brain, and lung cancers (Rana and Bhatnagar, 2021). G protein coupled receptors (GPCRs), Integrins, sigma receptors, Epidermal growth factor receptor, Sigma receptors (SRs) these over expressed receptors are frequently used in preclinical cancer models for selective drug delivery via receptor–ligand pairs (Rana and Bhatnagar, 2021). Follicle-stimulating hormone receptors, C-type lectin receptors, biotin receptors, and neuropilin receptors are some of the less common receptors that have lately been utilised (Kim et al., 2018). Other targets for tumor-selective accumulation of drug carriers include those expressed on tumour vasculature endothelial cells. The dependence of tumour growth on angiogenesis is a potential target for the development of therapies to prevent the production of new tumor-feeding blood vessels to reduce tumour progression (Xu J. et al., 2017). Anti-angiogenesis strategies are successful in reducing tumour development, with endothelial cells in tumour blood arteries being the primary targets. Cancer cells are denied of nutrition and oxygen, resulting in the tumor death (Teleanu et al., 2019). Targeting ligands are coupled to drug-loaded nanocarriers. These ligands find their corresponding target on the cancer cell surface, which is overexpressed. A wide variety of synthetic and natural chemicals of various chemical classes have been utilised to target nano systems against cancer cells. Antibodies (Ab) and other proteins (such as transferrin), Aptamers, tiny molecules like folic acid, and peptides are among the most utilised. It is important to identify optimum targets to maximise the efficacy of active targeting. Identifying receptors expressed at greater levels on target cancer cells than on normal cells is the justification for picking optimal targets (Gui et al., 2017).
ii) The Cell Membrane Lipid Components: When synthetic phospholipid analogues interact with biological membranes, they change the lipid content, membrane permeability, and fluidity. As a result, signal transduction pathways are disrupted, resulting in apoptosis (Torres et al., 2021). iii) Cell Surface Antigens or Proteins: The diseased cells either produce novel proteins or show differential (under/over) expression of proteins seen in healthy cells. Against such proteins, monoclonal antibodies are employed. The tumor-specific antigen that may be used to target drugs is one that is expressed exclusively and uniformly by all tumour cells (Khanna, 2012).
Sugars, folic acid, peptides, monoclonal-antibodies and specifically designed antibodies are examples of ligands that bind with specific receptors found on certain cell types with some degree of exclusivity. Nucleic acids like aptamers, tiny compounds like vitamins, and sugars like galactose, mannose, and other sugars have also been described as cellular targeting components (Figure 2) (Khanna, 2012).
Special carrier systems are required for cancer-targeted drug delivery applications. A SDDSs carrier is a special molecule, particle, composite, or system that can hold the drug, either through encapsulation or using a spacer. The TL is one of the most significant components for the successful delivery of drug payload in a SDDS. They segregate, transport, and hold drug payloads while delivering them to a specific targeted site. SDDSs require different carrier systems depending on the type of targeting mechanism. SDDSs carriers are specially designed vectors capable of encapsulating and/or bonding with a spacer moiety to keep the drug inside or on them (Figure 3). The medication delivery vehicle utilised must be non-toxic and non-immunogenic, stable, biocompatible, biodegradable, readily eliminated from the body, and unrecognizable by the host’s defence mechanisms. Other characteristics of drug carriers include high loading/encapsulation quantity with zero premature release of drug molecules, cell type or tissue specificity and site directing ability, and appropriate regulated release rate of drug molecules with an effective local concentration (Vallet-Regi et al., 2001). SDDSs based on carriers/TL provide benefits such as a larger surface-to-volume ratio, more reactive activity centres, more adsorption capacity, and other characteristics such as morphological preferences. The mechanism of control and drug secretion by these carriers at the target locations is very distinct and special. The reason is that the SDDS cleaves at first upon exposure to a particular stimulus, leading to continuous release for a long time afterwards (Rai et al., 2022).
SDDSs for targeted chemotherapy usually consist of the carrier, a cleavable linker, and the chemotherapeutic agent (Rana and Bhatnagar, 2021). The chemotherapeutic agent is chosen to be inactive in its conjugated form, which makes the SDDS a prodrug that is activated only in the tumor tissues.
This allows the chemotherapeutic agent to exert its desired toxic activity on the cancer cells in a fast and effective manner (Figure 4). Drug delivery systems having the ability to attach targeted moieties can be given locally or systemically. The drug payload might be delivered either outside or within the target cells. Larger drug-delivery systems can provide high local drug concentrations, whereas smaller drug-delivery systems can be directly endocytosed. The precise architecture of the SDDSs allows drug payloads to be delivered to particular tissues in systemic administration. The main focus of SDDSs platform that the drug does not easily extravasate during blood circulation, but rather only releases at the sites where the drug carriers concentrate via an active or passive targeting approach (Liu et al., 2016).
Early detection, location of the original tumour and metastases, killing cancer cells as effectively as possible while limiting harm to the patient (i.e., maximising therapeutic index), and high accumulation in tumour lesion are all important factors in cancer treatment (Kue et al., 2016). Drug targeting can be an effective strategy to address these challenges and overcome some of the drawbacks of non-targeted treatments. To deliver therapeutic payloads to tumour locations, there are two main mechanisms of drug targeting (Figure 5) (Torchilin, 2010).
Passive targeting relies on the use of large, generally polymeric molecules as carriers to increase permeability and retention. Targeting moieties such as ligands and antibodies are used in active targeting. These approaches differ from mechanistic or direct targeting options, which use monoclonal antibodies (mAbs) or small-molecule drugs to bind to surface proteins or interfere with elevated metabolic processes in cancer (Kue et al., 2016).
Active targeting entails the identification of cancer cells, which leads to increased drug accumulation and cellular internalisation (Figure 6) (Kim et al., 2018). In active drug targeting, antibodies, antibody fragments, and peptides are linked to drugs and delivery systems to function as homing devices, allowing them to bind to receptor structures expressed at the target region. In terms of receptors on the cell surface and antigen expression, cancer cells vs healthy cells can be distinguished (e.g., Folate Receptors, transferrin’s and Prostate-Specific Membrane Antigen (PSMA)). Trans-membrane communication is facilitated by cell surface receptors, which are proteins anchored in the cell membrane. Active targeting refers to the employment of externally coupled targeting moieties to improve carrier distribution. Because a quickly developing tumour needs a wide range of minerals and vitamins, tumour cells overexpress several tumor-specific receptors. Nanoparticles are tethered with ligands such antibodies, peptides and folic acid that serve as targets that bind to those receptors, which may aid internalisation following engagement, to provide efficient tumor-specific drug delivery. G-protein-coupled receptors (GPCRs), integrins, folate receptors, transferrin receptors, epidermal growth factor receptor (EGFR), fibroblast growth factors (FGFRs), and sigma receptors are all used to target medicines to tumour tissues and microenvironments (Rana and Bhatnagar, 2021).
Antibodies have long been recognised to detect malignancies, particularly receptors or surface antigens with a high level of expression on cancer cells. In 1975, the first tumour antigen-targeting monoclonal antibody (mAb) was produced and since then, several mAbs have been FDA-approved for cancer therapy (Baah et al., 2021). Long-term administration of mAb-conjugated drug carriers is thought to create immunological memory against antibodies, although targeted treatment using mAb-conjugated drug carriers is regarded a key possibly curative approach (Kim et al., 2018). Antibody fragments or chimeric antibodies have the potential to significantly lower immunogenicity when compared to full antibodies (Singh et al., 2018). Cetuximab, a recombinant chimeric mAb with a murine variable region and a human constant region that has been successfully used to treat cancer by targeting the epidermal growth factor receptor (Singh et al., 2018). Dual targeting antibodies, which have two epitope binding sites and may respond with single or dual targets, are an emerging approach for improving tumour targeting capabilities (Kim et al., 2018). Trastuzumab, a humanised mAb for the treatment of HER2-positive breast cancer, was developed in 1998 (Piccart-Gebhart et al., 2005). Bevacizumab, a tumour angiogenesis inhibitor that binds to vascular endothelial growth factor, was authorised in 2004 for the treatment of colorectal cancer (VEGF) (Ferrara et al., 2004). Recent research has attempted to encapsulate chemotherapeutic medicines in nanoparticles and then functionalize the particle surface with mAbs to preserve targeted effectiveness. The nanoparticles’ absorption and cytotoxic efficacy are improved by conjugated antibodies (Sanchez-Moreno et al., 2018).
When metabolic activities are enhanced, transferrin receptors are overexpressed on cell surfaces (Shen et al., 2018). The primary route of cellular iron absorption via clathrin-coated pits, with subsequent traffic to endosomal compartments, has been shown to involve membrane transferrin receptor-mediated endocytosis of the complex of transferrin-bound iron and transferrin receptor (Tortorella and Karagiannis, 2014). Anti-tumor medicines, proteins, and therapeutic genes have all been effectively delivered through this absorption route (Sanchez-Moreno et al., 2018). Due to high iron needs, transferrin receptors have been found to be increased in malignant cancer cells, including those of bladder, brain, breast, and lung cancers, as well as lymphoma (Kim et al., 2018).
Aptamers are three-dimensional DNA or RNA sequences that are short and single-stranded. Aptamers are nucleic acid molecules that fold into complex 3D shapes that bind to specific targets, much like antibodies (Dunn et al., 2017). They’re gaining a lot of attention in clinical trials for a variety of reasons, including their prolonged storage life, narrow batch-to-batch differences, low immunogenicity, and the ability to make chemical modifications for improved stability, serum half-life extension, and targeted delivery (Ni et al., 2021). Aptamers are more stable ligands in-vivo than antibodies, as they are produced chemically via in-vitro selection, a simple and inexpensive process and the time required to generate aptamers is comparatively short. Unlike antibodies, aptamers do not require any specific biological systems for their production (Thiviyanathan and Gorenstein, 2012). According to Zhang et al., cell-based SELEX has a lot of potential since cancer cells may be targeted specifically without knowing the proteins expressed on their surfaces; hence, different aptamers can be produced to target different kinds of cancer (Zhang et al., 2020). Aptamers, have limitations, on the other hand as their affinity is lower than the one of antibodies. The most significant success of aptamers thus far has been the development of FDA-approved aptamers that can bind to VEGF, a protein involved in angiogenesis (Kaiser, 2006). The coupling of aptamers to drug-delivery nanoparticles resulted in better targeting, more effective treatments, and more selective diagnostics (Sanchez-Moreno et al., 2018).
Folate is a B9 vitamin that is water soluble and interacts with folate receptor to aid cellular uptake. The folate receptor has the advantage of having low expression in normal tissues, but it is strongly expressed by numerous malignancies, particularly cancers that afflict women, such as cervical, breast, and ovarian cancer (Rana and Bhatnagar, 2021). Folic acid binds 20 times more to tumour cells than it does to normal epithelial cells or fibroblasts. Folate conjugation has been a popular approach for targeting drug delivery systems due to these appealing features (Rana and Bhatnagar, 2021).
CTPs (cell targeting peptides) are peptides that are short and have been chemically synthesised from peptide libraries and utilised as targeting ligands (Vives et al., 2008). CTPs are less than 10 amino acids in length and are more stable than traditional antibodies (Dissanayake et al., 2017). The amino acid sequences of CTPs identify targets, and the best sequences for interacting with specific cancer cell surface receptors are important for target identification. The most well-studied CTP is the Arginylglycylaspartic acid (RGD) peptide, which has a high affinity for integrin receptors overexpressed on the surfaces of 21 different types of cancer cells (Sanchez-Moreno et al., 2018).
Passive targeting refers to the accumulation of a drug or drug-carrier system at a specific location, which can be caused by physicochemical and pharmacological variables (Eckmann et al., 2014). There are few universally applicable methods for targeting tumours and tumour cells due to the phenotypic diversity of malignant cells and tumours (or their organelles). The most important approach is since many cancerous cells and vascularized solid tumours, as well as some vascularized metastatic tumour nodules, have an enhanced permeability and retention (EPR) effect that can be used for antitumor drug “passive targeting” (Maeda et al., 2000). Because many solid tumours have a leaky vasculature and absent or limited lymphatic drainage, high molecular weight molecules (such as polymers) and small particles with a diameter of ∼20–500 nm accumulate within the tumour tissue (Ulbrich et al., 2016). This form of targeting is based on the pathophysiology of the disease and the characteristics of tumour tissues, which may encourage drug accumulation in target tissues, reducing non-specificity (Rabanel et al., 2012). The vasculature of tumours is thought to differ from that of surrounding tissue. In comparison to typical well-organized arteries, tumour angiogenesis has featured that aid drug retention, such as high vascular density and permeability, defective vascular architecture, and poor lymph drainage from tumour tissue interstitial spaces. The Enhanced permeability and retention effect (EPR) effect is used in passive targeting to detect cancer spots (Sanchez-Moreno et al., 2018). The accumulation rate of drug-loaded nanocarriers in a tumour is much higher than in normal tissue because to the leaky endothelium of the tumour vasculature (Hossen et al., 2019). The concentration of anti-cancer drugs in the tumour might be raised several times when compared to healthy bodily tissue using this EPR effect. The passive targeting of gelatin (typeB) –based NPs was extremely effective in the delivery of genes at tumour locations, according to Kommareddy et al. (Kommareddy and Samiji, 2007). Another study utilised gelatin (type B) for the creation of NP-based DDSs that included plasmid DNA (pDNA) (Kaul and Amiji, 2002). Encapsulating DNA with PEGylated gelatine NPs improved the efficiency of targeting pDNA-expressed green fluorescent proteins and -galactosidase in vitro as well as in vivo. PEGylated-gelatin NPs have also been utilised to focus on DNA moieties in lung carcinomas, suppressing tumour development and angiogenesis in breast cancer cells (Das et al., 2020; Mi, 2020).
The active drug can be released at the location in released under strict restraint systems in response to specific physical, chemical, or biological processes, some of which are triggered internally and some of them are induced externally (Sershen and West, 2002). Stimulus-based drug delivery techniques have showed a lot of promise in terms of successfully targeting active drug moieties. The first time thermo-sensitive liposomes were utilised for medication delivery was in 1978 (Mazzotta et al., 2018). Over the years, scientists have created and widely used stimuli-responsive biomaterials for regulated drug administration, culminating in the development of the area of stimuli-responsive polymers (Mi, 2020). As a result, they may be divided into two types of responsive DDS (Figure 7).
1. Exogeneous stimuli-responsive SDDSs (Open-loop system): Externally controlled systems, or pulsatile systems, are also known as open-loop systems. Magnets, temperature, ultrasound, electric effects, and in these systems, external triggers were employed to deliver the drug (Wen et al., 2018).
2. Endogenous stimuli-responsive SDDSs (Close-loop system): These are also known as self-regulating or responsive medication delivery systems. pH, enzyme-responsive drug delivery systems, and other internal triggers like redox-responsive drug delivery systems, etc., controls the drug release from a closed loop control system (Wen et al., 2018). Drug release needs structural changes across the carrier or in specific layers or channels due to the fact that stimulation, according to SDDS (Mi, 2020). There are two types of stimuli: exogenous and endogenous. The utilisation of endogenous cues such as pH, glutathione (GSH), and certain enzymes allows for non-invasive, spatiotemporally regulated medication delivery (Mousavi et al., 2020). Different stimuli-based energy sources (light, ultrasonic, magnetic) that efficiently trigger drug release from nano cargos for effective delivery to specific locations (Table 1).
pH is one of the most commonly used triggers for drug release because of the significant pH difference seen at the cellular level between the cytosol (7.4), the Golgi apparatus (6.40), the endosome (5.5–6.0), and the lysosome (4.5–5.5) of cancer cells and in the tumour microenvironment (Mi, 2020). In general, the pH of cytoplasm, blood, and normal tissues is around 7.0 to 7.4, while endosomal/lysosomal organelles have a pH of 6 to 4, and the tumour microenvironment has a pH of 6.5–6.8 (Mi, 2020). The use of polymers with weak acids (e.g., carboxylic acid) or bases (e.g., primary and tertiary amines) groups is used to create pH-responsive systems that produce rapid changes in ionisation at the appropriate pH. The pH responsiveness of the polymer may be readily tweaked by changing the type of the co-monomers employed to make it (Darvin et al., 2019). A pH-responsive medication delivery system may be created by hydrazone bonding an anticancer agent to carriers or targeting ligands. A medication delivery system like this reacts to acidity inside tumour cells and releases drugs in a regulated manner. Following this technique, Du et al. developed PCC-Hyd-DOXDA, a custom-made dual pH-triggered polymer drug attached system. PCC-Hyd-DOX-DA has been found to be easily absorbed by MDA-MB-231 tumour cells at pH 6.8, whereas absorption at pH 7.4 is negligible (Du et al., 2011). The polyacidic pH-responsive system includes polyacrylic acid (PAAc) and polymethacrylic acid (PMAAc) (Chen et al., 2016a). The invention of a pH-triggered auto-fluorescent polymeric nanoplatform for the delivery of non-fluorescent aromatic nitrogen mustard chlorambucil (CBL) to cancer tumours was reported by Saha et al. (Saha et al., 2019). In another study, Zhang and others incorporated doxorubicin and dextran with a hydrazone linker, targeting hepatocytes with folate (Zhang et al., 2015). While pH is widely utilised in smart medicine administration, it should be combined with other stimuli such as temperature or redox to achieve extremely exact and precise release at the target locations. The use of acidic pH as a tumour microenvironment trigger has certain drawbacks. To begin with, the acid pH in perivascular areas is often remote from the blood flow, resulting in a lack of reaction of nanoparticles. In addition, pH changes in healthy tissues and malignant tissues are frequently similar (Pan et al., 2012; Cheng R. et al., 2013). For regulated release of doxorubicin, Nikravan et al. created a pH sensitive cross-linked nanoparticle system generated from various molar ratios of poly (acrylic acid) (PAA) and ethylene glycol dimethacrylate. With increasing cross-linking degrees, the pH-responsive behaviour of this nanocarrier was less effective. At pH levels of 1.2, 5.3, and 7.4, the release of the model drug doxorubicin was investigated (Dhanasekaran, 2015).
The redox-sensitive drug delivery system has sparked a lot of attention in the field of therapeutic strategies, because of its close ties to a variety of diseases, and it is being investigated a lot (Mura et al., 2013). Additionally, the redox-sensitive delivery system has the benefit of drug release within the cancer cell. Redox hemostasis is a crucial process for cell survival that involves glycolysis, glutathione synthesis, fatty acid oxidation, and glutaminolysis (Panieri and Santoro, 2016). However, in tumour cells, dysregulated redox hemostasis resulted in a shift in redox balance and an increase in ROS levels. An increase in ROS levels was caused by mitochondrial dysfunction, overexpression of NADPH oxidases, and changes in antioxidant enzymes (Arcucci et al., 2016). The redox potential in microenvironments tends to vary depending on the tissue, which may be exploited to develop redox-responsive delivery systems. The reducing environment of tumour cells is largely determined by NADPH/NADP+ and glutathione (GSH, GSH/GSSG), both of which have different reduction potentials and capacities (Wu et al., 2004). GSH levels differ between normal and cancerous cells. It ranges from 2 to 20 μM in blood and normal extracellular matrices, whereas it ranges from 2 to 10 mM in cancer cells which is 100- to 500- fold higher than the normal ranges (Liu et al., 2016). To produce redox-responsive carriers, the disulfide bond has been proven to be the major redox-sensitive linker (Liu et al., 2017). GSH levels in intracellular compartments (cytosol, mitochondria, and nucleus) are two to three orders of magnitude greater (2–10 mM) than in external fluids (2–20 mM). As a result, GSH is a well-known intracellular molecule that may be used to induce drug release within cells (Indermun et al., 2018). Many studies on the redox responsiveness of disulfide bonds are currently in progress, and diselenide bonds are also getting a lot of attention as well. Diselenide redox-sensitive bonds delivery systems are similarly sensitive to reduction and have characteristics similar to disulfide connections. Diselenide bonds can be used to create a more sensitive redox-responsive delivery system in tumour therapy because their bond energies are lower than S–S bonds (Se–Se 172 kJ/mol; C–Se 244 kJ/mol; S–S 268 kJ/mol) (Guo et al., 2018). Gang Cheng et al. synthesised the polycationic carrier OEI800-SeSex by adding the active ester containing diselenide bonds to the branched oligoethyleneimine 800 Da (OEI800) (Cheng et al., 2012). The ability of SDDSs to respond to reactive oxygen or nitrogen species (ROS or RNS) is still barely explored (Alvarez-Lorenzo and Concheiro, 2014). The main contributors to the intra- and extracellular redox potential associated with stress conditions, signalling cascades, diabetes, hypertension, atherosclerosis, or cancer are ROS such as hydrogen peroxide, superoxide, or OH radicals (Lallana and Tirelli, 2013). Oxidation-responsive SDDSs are a subset of redox-sensitive drug delivery systems that rely on reactive oxygen species (Torres et al.), primarily H2O2 and OH radicals (Darvin et al., 2019). A redox-sensitive polymeric nanoparticle for tumor-targeted medication delivery was described by Cho et al. The paclitaxel-incorporated nanoparticle was prepared using a redox-responsive biodegradable polymer that was capable of delivering paclitaxel in response to a reduction process (Das et al., 2020).
Due of its distinct benefits, such as substrate, specificity and excellent selectivity under moderate circumstances, enzymes employed as triggers in the construction of SDDSs have been a growing topic in recent years (Liu et al., 2016). Many enzymes have been put to work to enhance medication transport to cancer cells, including lipase, protease, trypsin, glycosidase, phospholipase, oxidoreductase, and others (De La Rica et al., 2012). The drugs will be released at the target locations by site-specific enzymatic cleavage by smart carriers/ligands bearing drug payload linked/conjugated to them via encapsulation or covalent bonding. The drug-release mechanism is triggered by several enzymes (Darvin et al., 2019). Proteases which degrade protein and peptides, a fantastic alternative for releasing medicines from liposomes (Hossen et al., 2019). Radhakrishnan et al. developed hollow nanocarriers triggered by the trypsin/hyaluronidase enzyme to deliver anticancer agents intracellularly (Radhakrishnan et al., 2014).
The phospholipase A2 (PLA2) enzyme is used to release medicines or expose target ligands from SDDSs that use liposomes or small unilamellar vesicles (SUVs) (Sanchez-Moreno et al., 2018). With the presence of Cathepsin B, an intracellular cysteine protease that was particularly overexpressed in tumour locations, the H-Phe-Lys-OH peptide could be broken. Hollow nanocarriers activated by the trypsin/hyaluronidase enzyme to deliver anticancer drugs intracellularly. MMPs (matrix metalloproteases) are a zinc-dependent family of endopeptidases that are well-known for their role in cancer prognosis (Liu et al., 2017) and have been extensively studied for drug delivery and imaging applications (Kessenbrock et al., 2010). Zhu et al. Developed MMP2-sensitive; PEG lipid conjugated liposomes with anti-nucleosome monoclonal antibodies modified on their surface to improve cancer targeting (Zhu et al., 2012). In another study, Chen et al. manufactured multifunctional poly (ethylene glycol)- blocked-poly (l-lysine) Biotin 6-maleimido-caproic acid (Biotin-PEG-b-PLL (Mal)-peptide) polymeric micelles enclosing doxorubicin to improve cancer cell uptake by endocytosis (Chen WH. et al., 2015). Despite its utility, enzyme responsive SDDSs lacks precise control over the system’s initial response time.
Light-responsive SDDSs have received much interest as a way to take advantage of either daily and seasonal exposure to natural solar irradiation or artificial sources of electromagnetic radiation with very specific wavelengths between 2500nm and 380 nm (Alvarez-Lorenzo and Concheiro, 2014). These photo-responsive drug delivery systems offer many advantages over other stimuli-responsive formulations for drug delivery because photochemical processes do not require additional reagents or catalysts, and the majority of by-products, if any, are harmless (Pan et al., 2021). Photosensitive carriers light-responsive smart drug delivery devices have an on/off drug release mechanism in response to irradiation stimulation. A photosensitive biomaterial is generally conjugated or encapsulated to a therapeutic agent in such SDDSs. The photosensitive material absorbs light (photons), which causes a conformational change in these smart-carriers, dramatically altering their structure and allowing the encapsulated/conjugated agent to be released at the desired site in a spatio-temporal controlled manner (Sanchis et al., 2019; Pan et al., 2021). UV and visible light can cause medication release from formulations that are applied to the skin or circulate through blood vessels near the body’s surface (e.g., eye structures). Drug release is usually initiated by reversible or irreversible photo-induced structural changes in smart-carriers. Photo-cleavable bonds can be used to conjugate medicines for light sensitive release (Alvarez-Lorenzo and Concheiro, 2014). For example, doxorubicin-encapsulated poly (lactic-co-glycolic acid) (PLGA)matrix particles with a gold over-layer, NIR-triggered release was observed. When cancer cells were exposed to NIR light, doxorubicin was released abruptly, resulting in high cancer cell toxicity and tissue ablation (Zhu et al., 2012). Specifically, carbon nanotubes and graphene nanoparticles (GNPs) are excellent candidates for light-triggered stimuli, in particular, the near-infrared (Prasanna et al.) range (Hossen et al., 2019). In order to destroy cancer cells, metallic nanocarriers are capable of absorbing light and convert it to heat (Alvarez-Lorenzo and Concheiro, 2014).
Azobenzene and its derivative-based nanocarriers are frequently utilised to regulate drug delivery at its target by using ultraviolet–visible light and/or visible light to facilitate structural change and drug release (Yan et al., 2012). By encapsulating doxorubicin and ammonium bicarbonate inside nanocarriers, Chen et al. created a bubble-generating thermo-responsive liposomal system. Ammonium bicarbonate decomposes at high temperatures, releasing carbon dioxide bubbles that generate permeable holes in the lipid bilayer of liposomal nanocarriers, allowing the loaded medication doxorubicin to be released quickly (Chen et al., 2013).
Ultrasound is a type of high-frequency sound wave that may have an impact on carriers used for controlled drug release at diseased sites (i.e., tumors). The ultrasound intensity could be adjusted for various applications. At low ultrasound frequencies (less than 20 kHz), it could be used for imaging, as well as disrupting smart-carriers to release cargos or increasing the permeability of cancer cell membranes at high ultrasound frequencies (greater than 20 kHz) (Mi, 2020). Ultrasound has become quite popular as a stimulus in clinical investigations because to its various benefits, including intrinsic tissue penetration, improved spatiotemporal control, and increased safety. Ultrasound has recently been popular in clinics as a diagnostic and therapeutic technique (Mi, 2020). The invention of nanocarriers with ultrasonic sensitivities for ultrasonography has expanded ultrasound procedures to become a unique and successful tool for capturing drug carriers and triggering drug release at the target locations by adjusting the ultrasound frequency, duty cycles, and exposure duration (Liu et al., 2016). Kruskal et al. used a nanocarrier-DOX-encapsulated delivery technique, followed by ultrasonic tumour irradiation, to accomplish tumour targeting, resulting in the drug’s systemic distribution. Wang et al. created amphiphile segments with ultrasound-sensitive oxyl-alkylhydroxylamine(-oa) linkages. To improve medication transport to hepatocellular carcinoma cells, hydrophobic DOX was encased between the hydrophobic amphiphile portion (Mi, 2020). Jung et al. created dual-functional Gd(III)-DOTA-modified sono-sensitive liposomes for doxorubicin administration and magnetic resonance imaging acquisition (Jung et al., 2012). In the realm of cancer treatment, ultrasonic therapy has been utilised in combination with micelles. Husseini et al. (Bulbake et al., 2017) examined the release of doxorubicin from Pluronic P105 micelles at various ultrasonic frequencies.
Magnetic-responsive drug delivery systems offer a non-invasive method of controlling the carriers’ spatiotemporal proximity to their targets. The use of magnetic particles for the delivery of anti-cancer drugs or antibodies to organs or tissues altered by disease has become an active and appealing field of research since the pioneering idea proposed by Freeman et al. (Freeman et al., 1960) that fine iron particles could be transported through the vascular system and concentrated at a particular point in the body with the aid of a magnetic field (Estelrich et al., 2015). This aids the device in releasing payloads under programmed external magnetic field exposure. MNPs (magnetic nanoparticles) have an abundance of active sites for bio molecule conjugation, allowing for accurate design and engineering to achieve their intended smart functions by applying a localised external magnetic field, such as long-term circulation in the bloodstream, target specificity to lesion tissues, and therapeutic delivery (Darvin et al., 2019). The most widely used core/shell magnetic nanoparticle has a wide range of magnetic properties. The drug is combined with a pharmaceutically stable ferromagnetic carrier in this complex. There are a number of ways to create magnetic-responsive systems, such using nanoparticles, or magneto-liposomes (Madaan et al., 2014). Jiang et al. created magnetically tunable BSA (Fe3O4/BSA) particles coated with negatively charged iron oxide nanoparticles. The release of these particles from bone marrow mesenchymal stem cells, where they were internalised with the help of an external magnetic field, was delayed (Freeman et al., 1960). Li et al. created a magneto-thermally responsive nanocarrier/doxorubicin (MTRN/Dox) using Mn-Zn containing ferrite magnetic nanoparticles (MZF-MNPs) to form a thermosensitive copolymer coating with absorbed chemotherapeutic combined with the magnetothermal effect of MZF-MNPs to allow controlled drug release at the tumour site under an alternating magnetic field (AMF) (Li et al., 2018). When compared to free Dox and MTRN/Dox treatment without the use of an AMF, the authors found that magnetic targeting of MTRN/Dox increased accumulation in tumour tissues and that AMF treatment was required for MTRN/Dox increased cytotoxicity. The MTRN/Dox with combined magnetic targeting and AMF treatment showed the greatest tumour volume reduction compared to the MTRN/Dox with only magnetic targeting or AMF treatments after injection into nude mice bearing tumours, indicating that it has potential as a liver cancer therapy. Fang, Xiuqi et al. developed a highly controllable process of Carbon Encapsulated Magnetic Nanoparticles (CEMNs) synthesis in arc discharge plasma. With an external magnetic field, CEMNs have been made more controllable with respect to both their size distribution and purity and with an external magnetic field, CEMNs have been made more controllable with respect to both their size distribution and purity. For the purpose of assessing the potential for CEMNs to be used in biomedicine, the human breast cancer cell line MDA-MB-231 was used to determine the cytotoxicity of CEMNs. Based on this finding, it is concluded that specific CEMN dosages can be utilized in biomedical settings such as MRI, cell migration control, hyperthermia, and medication administration (Fang et al., 2018).
Nanotechnology is a cutting-edge, innovative, and promising method of delivering a drug payload to tumour tissue. Nanoparticles (NPs), which range in size from 1 to 100 nm, can reveal both physical and chemical properties; are more likely to be accumulated in solid tumors by passively extravasation from the hyperpermeable tumor blood vasculature (Cabral et al., 2011). Nanoparticle delivery systems are broadly evaluated preclinically with other nanoparticle-constructed formulations and technologies that have been used so far in the clinic setup (Peer et al., 2007; Shi et al., 2010; Wicki et al., 2015; Navya et al., 2018; Murugan et al., 2021). There are two types of therapeutic and diagnostic nanoparticles: [a] inorganic nanoparticles (such as gold, silica, and iron oxide) and [b] organic nanoparticles (e.g., polymeric, liposomes, and micelles) (Figure 8) (Murugan et al., 2021). Conventional nanocarriers are unable to transport and release drugs in the desired concentration at the targeted site when stimulated externally or internally. They must be improvised or functionalized to make them smart (Lee et al., 2015). The following qualities should be present in smart nanocarriers. To begin, smart nanocarriers should avoid the immune system’s cleaning process. Second, they should only be gathered at the targeted site. Third, upon external or internal stimulation, the intelligent nanocarrier should release the cargo at the correct focusing on the targeting site (Hossen et al., 2019). Finally, they must supply chemotherapeutics as well as other things for example, genetic materials and imaging agents, and other similar compounds (Peer et al., 2007; Lee et al., 2015; Liu et al., 2016). Depending on the type and application of conventional nanocarriers, there are a few methods for transforming them to smart nanocarriers (Bhatia, 2016; Sur et al., 2019; Sirisha, 2020). First, nanocarriers must overcome a number of biological obstacles, including cleaning, making their way to the desired targeted site through the reticuloendothelial system (RES). The RES quickly removes the nanocarrier from circulation and it is then stored in the liver, spleen, or bone marrow as anti-cancer drug payload carrying nanocarriers (Nie, 2010). Second, nanocarriers may be functionalized to distinguish cancer cells from normal cells with pinpoint accuracy. Some proteins are overexpressed on surface-level of cancer cells (Kubler and Albrecht, 2018; Antignani et al., 2020). The smart primary targets are overexpressed proteins (Perez and Fernandez-Medarde, 2015). Nanocarriers are equipped with ligands that match the overexpressed proteins. Smart nanocarriers use ligands to detect cancer cells that have overly expressed receptor proteins of their surface (Sabir et al., 2021). Third, delivering the drug to the target cancerous cells does not imply that the operation is finished. The next major issue will be releasing the drug from the smart carrier while it is being stimulated. The surface of nanocarriers can be grafted with a variety of chemical groups to make them sensitive to the stimuli system (Alvarez-Lorenzo and Concheiro, 2014). Fourth, changes are made to allow anti-cancer drugs to be delivered when combined with another material such as genetic materials (Xu et al., 2014), imaging agents (Das et al., 2020), or even more anti-cancer therapies (Bose et al., 2018).
Smart NPs materials utilized in SDDSs can be classified into Organic and inorganic NPs based on number of organic and inorganic materials have been used to fabricate them with their own distinctive architecture and attached functionalities, and they have been evaluated for effective drug delivery to tumors (Srinivasan et al., 2015). Liposomes, dendrimers, micelles, are example of organic nanoparticles and carbon nanotubes, meso-porous silica NPs (MSNs), gold/silver NPs and Quantum Dots are example of inorganic nanoparticles.
Liposomes are tiny, artificially created vesicles that are completely enclosed by phospholipid bilayer membranes of varying sizes (20–10,000 nm) (Prasanna et al., 2018). Gregoriadis et al. were the first to employ liposomes as an example drug delivery device in 1971 (Gregoriadis et al., 1971). The large unilamellar liposomes (LUV) may then be produced by extrusion of multilamellar vesicles via polycarbonate filters, thanks to the invention of novel preparation technique (Prasanna et al., 2018). Liposomes have been widely used as advanced DDSs in numerous clinical trials, especially when the diameter of the liposome was reduced to less than 100 nm (Torchilin, 2012; Akbarzadeh et al., 2013).
The physico-chemical nature of lipids allows drug molecules to be encapsulated or intercalated into phospholipid bilayers, extending the medication’s location. Liposomes have been extensively studied for the delivery of imaging and therapeutic agents in a sustained and controlled manner for cancer diagnosis and treatment, with high diagnostic and therapeutic efficiency and minimal side effects (Koren et al., 2012).
Traditional liposomes have a number of flaws, including instability, insufficient drug loading, faster drug release, and shorter blood circulation times; therefore, they are not smart (Bozzuto and Molinari, 2015). The conventional liposomes need to be Traditional liposomes have been functionalized, making them ideal for use as SDDSs in order to makes them smart for utilized as SDDSs. Liposomes, like other nanocarriers, must overcome the challenge posed by the RES. Liposomes are helped to escape the RES by PEGylation. PEGylated liposomes have a longer blood circulation time as a result (Allen and Cullis, 2013). Smart nanocarriers can distinguish between cancerous and healthy cells. To actively target the cancer site, monoclonal antibodies, antibody fragments, proteins, peptides, vitamins, carbohydrates, and glycoproteins are usually attached/conjugated on the liposome (Sapra and Allen, 2003; Ruoslahti, 2012; Sawant and Torchilin, 2012; Noble et al., 2014). Smart liposomes drug delivery systems are responsive to various external and internal stimulation, including pH change, enzyme transformation, redox reaction, light, ultrasound and microwaves (Jin et al., 2016; Lee and Thompson, 2017). As a smart drug carrier system, ThermoDox, temperature-sensitive DOX liposomes developed by the company Celsion may be the closest formulation to the clinic so far. The doxorubicin may be liberated from ThermoDox at 41.5°C by taking advantage of the dipalmitoylphosphatidylcholine (DPPC) lipid crystallisation melting point (Chen et al., 2014). A novel range of cationic liposome-based systems has also been developed by integrating different cationic lipids for targeted delivery of anionic therapies such as small interfering RNA (siRNA), antisense oligonucleotides, and aptamers etc (Yingchoncharoen et al., 2016). For example, Peddada et al. created a complex nanocarrier by combining a cationic DOTAP (1,2-dioleoyl-3-trimethylammonium-propane) liposome, an anionic copolymer, and an antisense oligonucleotide with a poly (propyl-acrylic acid) (PPAA) polymer backbone. In human ovarian cancer A2780 cells, this complex nanocarrier with grafted poly (alkylene oxides) (g-PAO) increased antisense gene silencing activity. The authors also observed increased antisense oligonucleotide delivery in ovarian tumour xenografts, demonstrating that the DOTAP/PPAA-g-PAO nanocarrier system can be used for antisense oligonucleotide delivery for gene silencing (Peddada et al., 2014). Kang et al. created a dual-targeted liposomal system that used the Pep-1 peptide as a cell penetrating peptide and folic acid as an affinity ligand for the folate receptor (FR). The authors created this dual ligand (Pep-1 and folate)-modified liposome by using a short (PEG-2000) and long (PEG-3400) polymer linker to attach both ligands to the liposomal surface. In FR-positive HeLa and FR-negative HaCaT cells, cellular uptake of various fluorescent tagged liposomes was investigated. In FR positive cells, cellular uptake was higher than in FR negative cells, indicating that this multifunctional liposomal system is suitable for FR-selective drug targeting (Kang et al., 2015).
Polymer micelles are thermodynamically stable colloidal solutions formed by self-assembly of amphiphilic block copolymers (O'Reilly et al., 2006). Polymeric micelles are created using block copolymers, which are composed of two or more polymer chains with distinct hydrophilic characteristics. In an aqueous environment, these copolymers spontaneously combine into a core-shell structure. The core is made up of hydrophobic blocks, which may carry any hydrophobic medication, while the shell of hydrophilic blocks (Hibino et al., 2021). To develop therapeutic carriers, a variety of polymeric molecules have been investigated. Polymer-protein conjugates, drug-polymer conjugates, and supramolecular drug delivery systems are just a few examples. Only a few polymers have been accepted into clinical practise out of the many that have been proposed (Sanchez-Moreno et al., 2018). Biodegradable polymers, in particular, are highly preferred due to their high bioavailability, better encapsulation, controlled release, and low toxicity. Wang et al. demonstrated that paclitaxel-loaded micelles bound specifically to an MCF-7 cell-specific phage and found the cytotoxicity of the targeted paclitaxel-loaded phage micelles was significantly higher than that of the free drug or non-targeted micelle formulations against target MCF-7 cells, but not against non-target C166 cells (Wang et al., 2010). Ke et al. created micelles containing both thioridazine (which has been proven to kill cancer stem cells) and doxorubicin, presenting a promising method for breast cancer treatment that targets cancer as well as the cancer stem cells (Ke et al., 2014). Site-specific drug delivery smart nanocarriers are sought in the field of cancer therapy, with different molecules located in the external part of the nanoparticles that favour receptor-mediated cell-internalization (Wang et al., 2014). Different types of ligands, for example, folic acid and peptides, carbohydrates, antibodies, aptamers are utilised to adorn the micelle surface in order to aggressively target cancer cells (Sutton et al., 2007). The core of the micelle can be functionalized to release the anti-cancer medication at the correct concentration. pH gradients, temperature fluctuations, ultrasound, enzymes, and oxidation are among stimuli utilised in micelle based SDDSs (Sutton et al., 2007; Hossen et al., 2019). Co-delivery strategies in cancer treatment are very important for synergetic effects using multifunctional micelles. Seo et al. described a temperature-responsive micelle-based co-delivery system capable of carrying genes and anti-cancer drugs (Seo et al., 2015).
Dendrimers are synthetic polymers with a high degree of branching made composed of an initiator core and several layers of active terminal groups. Each layer is referred to as a generation (the core is referred to as generation zero), and it is made up of repeating units (Fischer et al., 2010). Dendrimers are great candidates for developing smart nanocarriers for biological applications due to their distinct chemical structure and ability to incorporate a large number of functional groups at spatially precise locations (Nanjwade et al., 2009). Dendrimers are versatile due to their branched structure. Furthermore, all of the surface’s active groups face outward, resulting in a higher drug encapsulation rate. Several kinds of dendrimers have been reported, including poly (propylene-imine) (PPI or POPAM), polylysine dendrimer, dendritic hydrocarbon, carbon oxygen-based dendrimer, porphyrin-based dendrimer, ionic dendrimer, silicon-based dendrimers, phosphorus-based dendrimer, and Newkome dendrimer (Hossen et al., 2019).
Traditional dendrimers are cleared rapidly by the immune system and have a low uptake by cancer cells. The alternative to these limitations is to modify the dendrimer. Chemical modification, copolymerization with a linear polymer, and hybridization with other smart nanocarriers have all been suggested as ways to get around these limitations (Bugno et al., 2015). Peptides, proteins, carbohydrates, aptamers, antibodies, and other substances can be used to modify the surface of dendritic structures to actively target the cancer site (Sirisha, 2020). The surface of the dendrimer may also be changed to respond to various stimuli, such as light, heat, and pH shift protein, and enzyme transformation (Rajasekhar Reddy et al., 2012; Wang et al., 2016). The cationic character of PAMAM, among other dendrimers, makes it ideal for the transport of genetic elements. The production of PAMAM has an impact on delivery efficiency. PAMAM-based nucleic acid delivery was initially reported by Haensler and Szoka in 1993 (Madaan et al., 2014). The use of a dendritic contrast agent for tumour imaging has shown to be highly effective (Hossen et al., 2019). Researchers Zhang and Shi found a multifunctional system that may be used to target cancer treatment using G5-PAMAM dendrimers coated with folic acid and doxorubicin (Zhang et al., 2018). Kaminskas et al. investigated the use of a PEGylated polylysine dendrimer conjugated to doxorubicin to promote controlled and prolonged doxorubicin exposure of lung-resident cancers. After 2 weeks of treatment, they found a 95% reduction in lung tumour burden in rats (Kaminskas et al., 2014).
Smart polymers are extremely efficient polymers that adapt to their surroundings. Natural, semi-synthetic, or synthetic polymers are used to make polymeric NPs (Brighenti et al., 2020). Polymeric nanosystems are formed by the polymerization of numerous monomer units, and under specific conditions, they may be structured and self-assemble with a nanometric size (10–100 nm) (Joglekar and Trewyn, 2013). Drugs can be entrapped, encapsulated, or bonded to polymeric NPs in the form of a nanosphere, a nano-capsule, or a drug conjugate, depending on the production technique (Prabhu et al., 2015). Polymeric capsules may be created by conjugating targeting ligands, which boost selectivity for cancer cells and improve intracellular drug delivery while decreasing various side effects and medication toxicity (Prabhu et al., 2015). Monoclonal antibodies (mAbs) or antibody fragments, aptamers, peptides, and small compounds, such as folic acid, are widely used as targeting ligands for polymeric capsule (Avramovic et al., 2020). These ligands specifically bind to antigens or receptors overexpressed on cancer cells (Rana and Bhatnagar, 2021). The efficacy of polymeric carriers modified with targeting ligands is determined by ligand properties such as density and receptor binding affinities, which can improve receptor internalisation and drug biodistribution. A drug is chemically bonded to the polymer via a linker/spacer in drug-conjugates. When the drug is released at the target site, the bond drug-linker/spacer is a common breakage point. FA-PEG-b-PCL-hyd-DOX, a multifunctional polymeric-drug conjugate containing a di-block PEG-PCL copolymer linked to DOX through a labile hydrazone bond and adorned with folic acid (FA), was developed by Guo et al. (Guo et al., 2016)]. Hu et al. created a nanoplatform with paclitaxel (PTX) encapsulated in a triblock PCL-PEG-PCL copolymer that confirmed sustained drug release and a lower cytotoxic effect when compared to free PTX injection (Hu et al., 2017). Guo et al. demonstrated the ability of the hydrophobic polymer PLGA to encapsulate the low-solubility medicine PTX in a poly (lactic-co-glycolic acid)-poly (ethylene glycol) (PLGA-PEG) nanoplatform, with longer circulation time and improved cancer inhibition confirmed when this SDDS was decorated with DNA aptamers in C6 glioma cells (Guo et al., 2011). In another study, Wang et al. found that methoxy PEG-PLGA NP co-loaded with hydrophilic DOX and hydrophobic PCT inhibited cancer development more effectively than polymeric micelles loaded with only one medication (either DOX or PCT), with the best anticancer effectiveness at a 2:1 concentration ratio (Yingchoncharoen et al., 2016). Duong et al. also developed a PEG-PLGA copolymer system for the delivery of DOX and PCT, which includes the targeting ligand folate and the TAT peptide, and which improves the cellular interaction between PEG-PLGA micelles in the kB cell line of a human oral cavity carcinoma (Duong, 2013). In essence, folate improves the drug carriers’ targeting ability, whereas TAT peptide is a cell-penetrating peptide (CPP) used to modify the carrier surface. In PEG-PLGA micelles, different concentration ratios of DOX and PCT were used, and a concentration ratio of 1:0.2 was found to be more effective than a concentration ratio of 1:1 (Duong, 2013). Jin et al. recently developed a promising smart delivery system based on the cationic deblock poly (ethyleneimine)-poly (lactic acid) (PEI-PLA) copolymer, which was designed to deliver the drug PTX and siRNA in a synergistic strategy in chemo or gene therapy for non-small cell lung cancer (Jin et al., 2018). This PTX NPs formulation enhances the drug’s effect by inhibiting target proteins involved in cancer cell metabolism and proliferation via siRNA. With high drug loading, a longer half-life in the circulation, lower toxicity, and an antiproliferative effect of PTX on A549 cells, this co-delivery system is a promising SDDS (Jin et al., 2018).
CNTs have attracted incredible interest in the biomedical field due both to their promising properties (such as high surface area, needle-like structure, considerable strength, flexible interaction with drug cargo, high drug loading capacity, outstanding optical and electrical features, high stability, biocompatibility, and ability to release therapeutic agents at targeted sites) and negative properties (most notably, lack of biodegradability and toxicity) (Alshehri et al., 2016; Costa et al., 2016; Singh et al., 2016; Azqhandi et al., 2017). CNTs are one-dimensional carbon allotropes with a nanostructure with a length-to-diameter ratio greater than one million that are made by rolling a thick sheet of graphene into a smooth cylinder with a diameter on the order of a nanometre (nm) (Rahamathulla et al., 2021). CNT can be fabricated in a number of ways, including rolling up a single layer of graphene sheet (single-walled CNT; SWCNT) or rolling up many layers to form concentric cylinders (multiwalled CNT; MWNT) (Rahamathulla et al., 2021). Traditional CNTs have difficulties dissolving in both aqueous and organic solvents, which makes it difficult to disperse homogeneously as compared with other nanoparticles. To make conventional CNTs smart, they must be functionalized chemically or physically (Li Z. et al., 2017). Several biological applications, including as proteins, nucleic acids, and drug transporters, have been successfully explored using CNTs that have been functional (Anzar et al., 2020). PEGylation is a critical step in increasing solubility, avoiding RES, and reducing toxicity (Kenchegowda et al., 2021). The polymer poly (N-isopropyl acrylamide) (PNIPAM) is temperature sensitive. PNIPAM could be used to modify CNTs for temperature stimulus because of their low critical stimulus temperature (LCST) (Schmaljohann, 2006). For enzyme-responsive drug release, a disulfide cross-linker based on methacrylate cysteine is used. An ionizable polymer with a pKa value of 3–10 is an ideal candidate for pH responsiveness. Weak acids and bases show a change in the ionization state upon pH variation (Schmaljohann, 2006). Researchers developed a PEGylated CNT complex loaded with paclitaxel for the treatment of breast cancer in an early study. When compared to free paclitaxel alone, the CNT-paclitaxel complex showed better treatment efficacy in a 4T1 murine breast cancer model (Liu et al., 2008). Jain et al. reported that chemical modification of CNTs by carbohydrate d-galactose can generate a novel cascade of chemical functionalization of MWCNTs (Jain et al., 2009). Galactosylated MWCNTs are utilised to deliver active ligands (like galactose) to tumour sites as a targeted drug (Jain et al., 2009). SWCNTs are more efficient in drug distribution than MWCNTs because their walls are more defined and MWCNTs have more structural flaws. CNTs have been studied as nanocarriers for medication delivery as well as biomolecules including DNA, siRNA, and others. Functionalized carbon nanotubes can be utilised as early cancer detection techniques (Hossen et al., 2019). Cheng et al. recently developed a PLGA-functionalized CNT system for delivering the proapoptotic protein caspase-3 (CP3) to bone cancer cells with reduced toxicity (Cheng Q. et al., 2013). This nanocomplex showed efficient transfection of CP3 in cells and suppressed their proliferation. In a CNT-PLGA system, transcription factors were well delivered with a good transfection rate, and the payload release profile could be modified by adjusting the PLGA polymer molecular weight and ratio (Cheng Q. et al., 2013). For the treatment of cancer, Mehra et al. created a multiwall PEG-CTN complex loaded with doxorubicin (DOX) (Mehra and NKJain, 2015). On the surface of this DOX/ES-PEG-MWCNT system, both folic acid (FA) and estrone (ES) were attached as targeting molecules. They observed a long survival of Balb/c mice with MCF-7 tumors treated with DOX/ES-PEGMWCNT nano formulation (Mehra and NKJain, 2015).
Mesoporous materials, which have pore sizes ranging from 2 to 50 nm, high surface areas, adjustable pore sizes and internal architectures, and a plethora of modifiable sites, are frequently utilised in catalyzer, sensor, and molecular sieve research (Shi et al., 2020). In the recent decades, mesoporous materials have shown significant potential for SDDS. Due to their drug loading capacity, desirable biocompatibility, and practical feasibility, MSNs have attracted the attention of researchers (Farjadian et al., 2019). The versatility of MSNs is due to their tuneable particle size (50–300 nm), tuneable pore size (2–6 nm), high surface area, and biocompatibility (Hossen et al., 2019). Tuneable particle size is an essential criterion to be a smart nanocarrier, and tuneable pore size allows drugs of different molecular shapes to be loaded. The high surface areas of the pores and external surface are suitable for grafting different functional groups on MSNs (Yingchoncharoen et al., 2016; Hossen et al., 2019). Typical MSNs have low circulation half-lives due to hemolysis of red blood cells, non-specific binding to human serum proteins, and phagocytosis of human THP-1 mono-cytic leukemia macrophages. PEGylation can help to reduce the negative impact of these variables (He et al., 2010). MSNs are used as stimulus-sensitive drug delivery systems, and the surface pores are also blocked to build gatekeeper-based delivery systems, thanks to their adaptability. For targeted administration of doxorubicin, Cheng et al. developed and synthesised a pH responsive multifunctional MSN system made up of poly dopamine, poly (ethylene glycol), and folic acid (Cheng et al., 2017). The findings revealed significant anticancer activity and release of the encapsulated drug payload from MSNPDA-PEG-FA nanosystems in acidic pH (Cheng et al., 2017). Yang et al. created disulfide-bridged ‘degradable dendritic mesoporous organo-silica nanoparticles (DDMONs) to deliver therapeutic proteins to cancer cells (Yang et al., 2016). In B16F0 cancer cells, this DDMONs system demonstrated a greater rate of glutathione (GSH)-responsive degradation and release of the therapeutic protein, but in normal HEK293t cells, the nanoparticle degradation was modest (Yang et al., 2016). Targeted MSNs therapies work by interfering directly with specific molecules involved in cancer growth and progression or indirectly by activating the immune system to detect and destroy cancer cells to prevent cancer from spreading (Colilla et al., 2010). For example, many anticancer medicines require “zero release” before reaching the target site. Efficient distribution of doxorubicin (DOX) utilising MSNs coated with a PEG copolymer employing 50 nm MSNs, which can reach a size of 110 nm when coated with the copolymer (Gary-Bobo et al., 2012; Bharti et al., 2015).
As GNPs (gold nanoparticles) have a high drug loading capacity, biocompatibility, and stability, they can be used as nano-carriers to transport drugs. In order to create GNPs with the desired morphology, the seeded growth technique is used (Dhanasekaran, 2015). The size of GNPs can be controlled by adjusting the seed to chloroauric salt ratio and the pace at which reducing agents are added, and the form of GNPs may be controlled by using surfactant intelligently to tailor the end facets (Wang et al., 2020). Medicines are connected to the surface of GO in spherical or rod-shaped GNPs; in hollow-structured GNPs, drugs are enclosed in the hollow cave (Shi et al., 2020). Non-covalent and covalent interactions are involved in the conjugation of GNPs and medicines (Wang et al., 2020). Among the non-covalent interactions are electrostatic and hydrophobic interactions, which are weak forces linking drug payload molecules to GNPs (Shi et al., 2020). The gold–thiolate bond (Au–S) is primarily responsible for covalent connections, and thiol-containing molecules are connected on the surface of GNPs in this fashion (Xue et al., 2021). For example, thiol-linked drugs or genes are linked on the surface of GNPs to release drug delivery; thiol-linked targeting groups are also decorated on the surface to improve targeting efficacy; and polymers with stimuli responsibility are functionalized on GNPs via Au–S link or electrostatic attraction, endowing the system with TME responsibility (Cobley et al., 2011). The surface plasmon resonance (SPR) phenomena in GNPs is particularly fascinating, which allows them to change light into heat and disperse that heat to kill cancer cells (Sztandera et al., 2019). Ideally, SDDS should be chemically stable in biological media, biocompatible, and targetable. Traditional GNPs are unstable in blood and are more likely to be absorbed by RES. In order to overcome these limitations, gold nanocarriers must be PEGylated. PEGylated GNPs exhibit enhanced solubility and stability under physiological conditions (Qian et al., 2011). GNPs can be modified by ligands or tumor-specific recognition molecules to deliver targeted drugs such as transferrin, folic acid, epidermal growth factor (EGF), or any number of monoclonal antibodies can be conjugated to the surface of GNPs (Vines et al., 2019). Drugs can be released from GNPs through either (1) external stimulation (laser, ultrasound, X-ray, light) or (2) internal stimulation (pH, redox condition, matrix metalloproteinase) (Tian et al., 2016; Yao et al., 2016). Trastuzumab (anti-EGF receptor monoclonal antibodies) was conjugated with citrate-coated GNPs to target EGF receptors in human SK-BR-3 breast cancer cells, resulting in downstream expression of EGF receptors and a 2-fold increase in trastuzumab cytotoxicity, even at low GNP concentrations (Jiang et al., 2008). Another study used GNPs to treat pancreatic cancer with gemcitabine and cetuximab. The cancer site could be identified using GNPs conjugated with fluorescently labelled heparin (Bansal et al., 2020).
QDs are semiconductor nanoparticles with excellent photoluminescence properties, optical properties, and electronic properties that make them suitable for image guided drug delivery. (Mi, 2020). This smart carrier could be used to visualise the tumour and can be functionalized with the targeting ligands for tissue specific therapeutic delivery application for the drug is delivered to the desired location. Various targeted QDs have been studied for diagnosis and therapeutic delivery applications over the years (Badıllı et al., 2020). Chen et al., for example, developed a quantum dot-based FRET system for image-guided drug delivery in the nucleus (Chen H. et al., 2015). In this study, graphene quantum dots (GQDs) were prepared and decorated with TAT peptide to facilitate nuclear localization. The quantum dot-based FRET system enabled real-time monitoring of therapeutic delivery as well as image-based tracking of release (Chen H. et al., 2015). Recent research has shown that conjugating metal based QDs with lipid nanocarriers reduces their cytotoxicity and improves their safety (Olerile et al., 2017). Iannazzo et al. recently demonstrated the potential of graphene QD-based targeted drug delivery. To exploit the biotin receptor overexpressed on tumour cells, they covalently conjugated QDs to the tumour targeting ligand biotin. This system utilizes the pH stimuli to release the drug payload at desired targeted site (Iannazzo et al., 2017). The inherent florescence of QDs makes them ideal for cancer imaging. Ovarian cancer has been diagnosed using a folic acid complex (Zhao, 2016). A DNA aptamer was added up to the top of the created QDs to target mutant MUC1 mucin, which is overexpressed in ovarian cancer. Doxorubicin was attached to the surface of the QD by a pH labile hydrazine linker, which hydrolyses at the acidic pH of the tumour microenvironment, allowing for regulated drug release (Dutta et al., 2021).
In the past 3 decades, monoclonal antibodies have evolved from scientific tools into powerful therapeutics. A monoclonal antibody (mAb) is covalently attached to a cytotoxic drug payload via a chemical linker in an antibody–drug conjugate (ADC). It combines the advantages of highly specific targeting and a highly potent killing effect to achieve accurate and efficient cancer cell elimination, and it has become one of the hotspots for anticancer drug research and development (Fu et al., 2022). The first ADC drug, Mylotarg® (gemtuzumab ozogamicin), was approved by the US Food and Drug Administration (FDA) in 2000 for adults with acute myeloid leukaemia (AML), signalling the start of the ADC era of cancer targeted therapy (Norsworthy et al., 2018).
By December 2021, 14 ADC drugs had been approved worldwide for both haematological malignancies and solid tumours. Furthermore, there are currently over 100 ADC candidates in various stages of clinical trials (Fu et al., 2022). Figure 9 depicts the general mechanism of action for an ADC. Following administration, the ADC’s mAb component recognises and binds to the target tumour cells’ cell surface antigens. After antigen binding, the ADC–antigen complex is internalised by the cancer cell through endocytosis (Ritchie et al., 2013). In the case of non-cleavable linkers, the internalised complex is broken down via proteolysis within lysosomes, releasing the cytotoxic payload inside the cell, whereas the mechanism of payload release for ADCs with cleavable linkers varies depending on the specific linker used (Sanadgol and Wackerlig, 2020). The liberated payload binds to its target in all cases, causing cell death through apoptosis (Tong et al., 2021).
Just like ADCs, Small Molecule Drug Conjugates (SMDCs) are another class of SDDSs. An SMDC comprises of a targeting ligand, a releasable bond, a hydrophilic spacer, and a therapeutic drug payload (Rana and Bhatnagar, 2021). SMDCs which use biomarker-targeted small molecule compounds as the targeting moieties, in contrast to ADCs, provide a new, less established approach to targeted delivery. However, SMDCs have several advantages, including 1) a non-immunogenic nature, 2) a much more manageable synthesis, and 3) lower molecular weights, all of which contribute to a high potential for cell penetration in solid tumours (Zhuang et al., 2019). SMDCs have been successfully targeted against Folate Receptor, Prostate Specific Membrane Antigen, Somatostatin Receptor, and Carbonic Anhydrase IX (Ghiasikhou et al., 2019). More recently, other receptors (such as biotin receptor, bombesin receptor, Eph receptor) have gained attention as they can be potentially targeted with small molecules (Rana and Bhatnagar, 2021). Among them, the folate receptor (Iannazzo et al.) has received most of the attention and several compounds primarily developed by Endocyte have entered clinical trials (Zhuang et al., 2019). The first compounds that used folate as a targeting moiety were used for imaging. Etarfolatide was one of the first products in its class to make it to the clinic (Patel et al., 2021). A SMDC most commonly used as a delivery vehicle for folic acid is vintafolide, a conjugate containing desacetylvinblastin hydrazide (DAVLBH). Developed at Endocyte and later licensed to Merck in a $1 billion deal, this drug reached phase III clinical trials for platinum-resistant ovarian cancer. However, the results of the clinical trials, reported shortly after the European Medicines Agency (EMA) had recommended the drug approval, halted its development (Vlahov et al., 2017; Reddy et al., 2018). An innovative compound in the clinic, the peptide based SMDC 177Lu-DOTATATE, has been approved by the USFDA and EMA for the treatment of gastroenteropancreatic neuroendocrine tumors (Curtis et al., 2014). Several recent preclinical studies demonstrated the striking potency of various chemotherapeutic agents such as PEN-866, EC145, AEZS-108, NGR-TNF (Asn-Gly-Arg-TNFa), and EC0225 in xenograft models of solid tumours including breast, pancreatic, and small cell lung cancer in xenograft models of solid tumours including breast, pancreatic, and small cell lung cancer (SCLC) (Patel et al., 2021). In theory, SMDCs can deliver cytotoxic agents to target cells that overexpress specific receptors such as FR, PSMA, and others by targeting ligand to the receptors and allowing it to be internalised via receptor-mediated endocytosis (Leamon and Jackman, 2008). Once the SMDC–receptor complex is internalised, it travels from the endosome to the lysosome, where the cytotoxic drug is released from the SMDCs via deconjugation (cleaving the linker) in intracellular compartments, resulting in cell death (Patel et al., 2021). SMDCs targeting cancer endocytosis, heat shock protein 90 (HSP90), BCR/ABL fusion protein, PSMA, GLUT1, LRP1, aminopeptidase N (APN), and somatostatin receptor are all in clinical trials (SSTR). All of them are currently undergoing clinical trials in various stages (Table 2). The SMDC approach, on the other hand, has been widely used in the fields of radiotherapy and cancer diagnosis. The efficacy of ligand-targeted compounds used for cancer imaging has been demonstrated in clinical trials by the identification and localization of tumours (Srinivasarao et al., 2015; Srinivasarao and Low, 2017; Banerji et al., 2018; Patel et al., 2021; Rana and Bhatnagar, 2021).
TABLE 2. Some medicines for cancer treatment based on SDDSs that are in clinical trials or already commercialized.
An aptamer is a simple, small, single-stranded deoxyribonucleic acid (DNA) or ribonucleic acid (RNA) that folds into a three-dimensional conformation just like an antibody for binding to target molecules (Nimjee et al., 2017). Aptamers can typically bind to various molecules, such as overexpressed receptors, for diagnostic and therapeutic purposes using an in vitro iterative selection method known as SELEX (Systematic Evolution of Ligands by Exponential Enrichment) (Maghsoudi et al., 2019). Aptamers are more beneficial, less toxic, and easier to modify and synthesise in the lab than antibodies. Furthermore, aptamers were chosen as a new family of cancer therapeutics because of their numerous advantages over recent cancer therapies such as monoclonal antibodies. Their promising affinity for specific tumour cell lines, higher robustness than antibodies, fast in vitro selection, low immunogenicity, and better penetration into solid tumour tissue are just a few of these advantages (Hori et al., 2018). Antisoma developed AS1411, a 26-nt guanosine-rich G-quadruplex DNA oligonucleotide that was the first aptamer to enter clinical trials for cancer treatment. AS1411 was discovered in a screen for antiproliferative DNA oligonucleotides, not by SELEX (Hori et al., 2018). Aptamer–drug conjugates are particularly useful in the treatment of chemotherapeutic agents with systemic side effects. Doxorubicin (Dox) has been used as a model agent for cell-specific aptamer conjugation. Dox is a traditional chemotherapeutic agent that induces cancer cell death by intercalating into DNA. Dox can non-covalently conjugate to aptamers via intercalation into their GC-rich regions for delivery into specific cells, according to some studies (Bagalkot et al., 2006; Hu et al., 2012; Subramanian et al., 2012). Several other groups have reported novel types of aptamer–Dox conjugates in the recent years. Wen et al. isolated a CD38-targeting DNA aptamer and used CG-cargo to non-covalently conjugate Dox to it in a CG-repeat structure (Wen et al., 2016). The aptamer-Dox conjugate was formed with a 1:5 M ratio of aptamer to Dox using the CG-repeat structure. It specifically released Dox in tumour cells when systemically administered to multiple myeloma-bearing mice, inhibiting tumour growth and improving mouse survival rates (Wen et al., 2016). Trinh et al. developed AS1411-Dox, a drug-DNA adduct, by crosslinking Dox and AS1411 with formaldehyde overnight at 10°. AS1411-Dox inhibited tumour growth in hepatocellular carcinoma-bearing mice without causing severe toxicity in non-tumor tissues when given systemically (Trinh et al., 2015). Covalent conjugation to aptamers has also been utilized to target other chemotherapy agents to cancer cells. For example, Zhao et al. developed a cell-specific aptamer—methotrexate (MTX) conjugate to specifically inhibit AML (Zhao et al., 2015). In the first step, they isolated a DNA aptamer that targets CD117, an antigen that is highly expressed on AML cells. MTX was covalently conjugated with DNA aptamers with G-quadruplex structures using N-hydroxysuccinimide (NHS). The CD117 aptamer-MTX conjugate specifically inhibited the cell growth in AML (Zhao et al., 2015).
It is inevitable that every opportunity will come with some challenges. There is no exception to this rule with SDDSs. In order for SDDSs to succeed, they must overcome the toxicity of nanocarriers in the human body, the cost-effectiveness of the system, the heterogeneity and diversity of cancers, and the lack of specific regulatory guidelines (Shi et al., 2017).
To kill cancer cells, smart carriers needs to transport and release anti-cancer drugs at the targeted sites. In nanocarrier delivery, the biggest challenge is the toxicity of nanocarriers, which will need to be studied further in the future, as well as the limitation between their use in small animals and their clinical effectiveness. Depending on the chemical composition, size, shape, specific surface area, surface charge, as well as the presence or absence of a shell around the nanocarrier, conventional nanocarriers can accumulate in different vital organs including the lungs, spleen, kidneys, liver, and heart. Similarly, in case of ADCs, the high cost of production, limited penetration into solid tumor masses, and premature drug release the main concerns (Lo et al., 2022).
The challenges associated with ligands include selection of an appropriate ligand, developing conjugation strategies, and characterizing the release of the drug from the ligand (selection of a linker). A carrier-based challenge involves carrier selection and carrier physicochemical and pharmacokinetic characterization. SDDSs formulation requires additional steps in chemical synthesis and purification. Furthermore, there are additional quality control and regulatory steps, increased costs, and longer timeframes. The majority of these carriers have been designed and tested in small animal models, with excellent therapeutic results; however, the translation of animal results into human success has been limited. In order to fully comprehend the advantages and disadvantages of these vehicles, more clinical data is needed.
Another challenge that limits application of SDDSs is functional group complementarity as well as release of drug in active form in cellular melieu. pH-responsive delivery can be accomplished by the controlled protonation of the functional groups in the linker of SDDS and pH-responsive bond cleavage. Similarly, pH-induced bond cleavage can release drugs directly or by breaking up the carrier’s topological structure. Chemical bonds that can be cleaved by pH-responsive materials include hydrazine, oxime, amide, imine, ketal or acetal, orthoester, and phenyl vinyl ether. When drugs are linked to the carrier by these bonds, their cleavage in an acidic environment leads to their release. For the redox-responsive system, commonly used linkers include disulfide and diselenide linkages which will be broken with significant increases in the level of surrounding reducing agents such as GSH. On similar lines, it is necessary for enzyme-responsive SDDSs to tolerate specific conditions of pH and presence of other ions in cellular mileiu that may interfere with enzyme activity. Besides in the DDS the substrate should mimic and also be complementary to the binding pocket of target enzyme for the targeted enzyme to act, the actions of the targeted enzyme must alter the properties of the linkers used in SDDS as well. It is also worth mentioning that the long-term effects of associated with toxicity due to accumulation of nanocarriers or other SDDDs in patients is a necessity that requires investigation. All the above are formidable challenges for medicinal chemists; however, the potential of SDDSs in translational medicine cannot be denied.
In the future, SDDS will combine diagnosis and targeted therapy into one, centralized treatment system. A novel theranostic strategy has the potential to facilitate highly selective, effective, and relatively sensitive treatments of cancer and other chronic diseases, leading to personalized chemotherapy with improved outcomes for patients. All smart drug delivery systems all share the same goal: to benefit patients. Future research on smart DDSs for controlled drug delivery should concentrate on clinical translation so that more stimulus-sensitive nanomedicine may be employed in clinical settings.
The pharmaceutical and biotechnology industries are undergoing a significant transformation. Although the last decade has seen significant advances in drug delivery yet challenges remain. Smart drug delivery systems have the potential to overcome the limitations of traditional drug delivery methods. The development of smart drug delivery systems holds a lot of promise for pathology-specific medication design and delivery techniques that are tailored as per therapeutic needs.
Smart drug delivery systems incorporate several benefits, which includes i) a long shelf life and is not readily degraded or cleared by the reticuloendothelial system (RES) during blood circulation, ii) efficient intracellular drug delivery at the tumour targeted region or location that meets iii) drug pharmacodynamics of kinetics and spatial control, and iv) tolerability.
Another class of SDDSs include stimuli-responsive nanocarriers that can be used to deliver diagnostic and therapeutic substances to specific locations. Many improvements in stimulus sensitive delivery systems have been made in the last few years. In this review, literature studies of internal stimuli such as pH, redox, and enzyme demonstrate a superior property of controlling and adjusting the location and time of drug release without the use of any other external remote apparatus, leading in increased therapeutic drug internalisation in target cells and external stimuli such as light, ultrasound, and magnetic fields can also be utilised to initiate or increase drug release at disease sites. Smart Nanocarriers, a marvel of modern technology, are critical in the delivery of anti-cancer drugs. Because of their outstanding characteristics for cancer therapy, organic and inorganic based smart nanomaterials have recovered a lot of interest.
Changes will be made in clinical trials to allow for the specific targeting of cancer cells, which will enhance cancer patients’ quality of life by minimising the side effects of chemotherapeutic drugs and improving overall survival. Liposomes, nano-suspension, polymer nanoparticles, nanocapsule, micelles, doxil, and other nanocarriers have been authorised in clinical trials (Mi, 2020).
SDDS has a bright future and offers many opportunities for improving quality of life and patient compliance, and it could become the future of Translational Medicine.
AR wrote the manuscript with support from MA. All the diagrams were drawn by AR. AR, MA, and PS collected references. SB and BCD supervised the whole work and edited the manuscript. All the authors approved this manuscript.
Author SB gratefully acknowledges Indian Council of Medical Research (ICMR), Government of India for Project Grant (5/13/15/2015/NCD-III). Author AR gratefully acknowledges Indian Council of Medical Research (ICMR), Government of India for Senior Research fellowship Grant (F.No. 3/2/2/99/2022/NCD-III). Author PS gratefully acknowledges University Grants Commission (UGC), Government of India for Junior Research fellowship Grant. All authors gratefully acknowledge the infrastructure support provided by Amity Institute of Biotechnology, Amity University Noida.
The authors declare that the research was conducted in the absence of any commercial or financial relationships that could be construed as a potential conflict of interest.
All claims expressed in this article are solely those of the authors and do not necessarily represent those of their affiliated organizations, or those of the publisher, the editors and the reviewers. Any product that may be evaluated in this article, or claim that may be made by its manufacturer, is not guaranteed or endorsed by the publisher.
Ak, G., Yilmaz, H., Güneş, A., and Hamarat Sanlier, S. (2018). In vitro and in vivo evaluation of folate receptor-targeted a novel magnetic drug delivery system for ovarian cancer therapy. Artif. Cells Nanomed. Biotechnol. 46, 926–937. doi:10.1080/21691401.2018.1439838
Akbarzadeh, A., Rezaei-Sadabady, R., Davaran, S., Joo, S. W., Zarghami, N., Hanifehpour, Y., et al. (2013). Liposome: Classification, preparation, and applications. Nanoscale Res. Lett. 8, 102. doi:10.1186/1556-276x-8-102
Al Faraj, A, Shaik, Apshaik , , and Asjijon, (2015). Magnetic single-walled carbon nanotubes as efficient drug delivery nanocarriers in breast cancer murine model: Noninvasive monitoring using diffusion-weighted magnetic resonance imaging as sensitive imaging biomarker. Int. J. Nanomedicine 10, 157–168. doi:10.2147/IJN.S75074
Allen, T. M., and Cullis, P. R. (2013). Liposomal drug delivery systems: From concept to clinical applications. Adv. Drug Deliv. Rev. 65, 36–48. doi:10.1016/j.addr.2012.09.037
Alsawaftah, N., and Pitt, W. G. H. (2021). Dual-targeting and stimuli-triggered liposomal drug delivery in cancer treatment. ACS Pharmacol. Transl. Sci. 4, 1028–1049. doi:10.1021/acsptsci.1c00066
Alshehri, R., Ilyas, A. M., Hasan, A., Arnaout, A., Ahmed, F., and Memic, A. (2016). Carbon nanotubes in biomedical applications: Factors, mechanisms, and remedies of toxicity. J. Med. Chem. 59, 8149–8167. doi:10.1021/acs.jmedchem.5b01770
Alvarez-Lorenzo, C., and Concheiro, A. (2014). Smart drug delivery systems: From fundamentals to the clinic. Chem. Commun. 50, 7743–7765. doi:10.1039/c4cc01429d
Antignani, A., Ho, E. C. H., Bilotta, M. T., Qiu, R., Sarnvosky, R., and FitzGerald, D. J. (2020). Targeting receptors on cancer cells with protein toxins. Biomolecules 10, 1331. doi:10.3390/biom10091331
Anzar, N., Hasan, R., Tyagi, M., Yadav, N., and Narang, J. (2020). Carbon nanotube - a review on Synthesis, Properties and plethora of applications in the field of biomedical science. Sens. Int. 1, 100003. doi:10.1016/j.sintl.2020.100003
Arcucci, A., Ruocco, M. R., Granato, G., Sacco, A. M., and Montagnani, S. (2016). Cancer: An oxidative crosstalk between solid tumor cells and cancer associated fibroblasts. Biomed. Res. Int. 2016, 1–7. doi:10.1155/2016/4502846
Avramovic, N., Mandic, B., Savic-Radojevic, A., and Simic, T. (2020). Polymeric nanocarriers of drug delivery systems in cancer therapy. Pharmaceutics 12, 298. doi:10.3390/pharmaceutics12040298
Azqhandi, M. H. A, Farahani, B. V., and Dehghani, N. (2017). Encapsulation of methotrexate and cyclophosphamide in interpolymer complexes formed between poly acrylic acid and poly ethylene glycol on multi-walled carbon nanotubes as drug delivery systems. Mater. Sci. Eng. C 79, 841–847. doi:10.1016/j.msec.2017.05.089
Baah, S., and Mrahman, K. M. (2021). Antibody-drug conjugates-A tutorial review. Molecules 26, 2943. doi:10.3390/molecules26102943
Badıllı, U., Mollarasouli, F., Bakirhan, N. K., Ozkan, Y., and Ozkan, S. A. (2020). Role of quantum dots in pharmaceutical and biomedical analysis, and its application in drug delivery. TrAC Trends Anal. Chem. 131, 116013. doi:10.1016/j.trac.2020.116013
Bagalkot, V., Farokhzad, O. C., Langer, R., and Jon, S. (2006). An aptamer-doxorubicin physical conjugate as a novel targeted drug-delivery platform. Angew. Chem. Int. Ed. 45, 8149–8152. doi:10.1002/anie.200602251
Banerji, U., Cook, N., and Evans, T. J. (2018). A Cancer Research UK phase I/IIa trial of BT1718 (a first in class Bicycle Drug Conjugate) given intravenously in patients with advanced solid tumours. Am. Soc. Clin. Oncol., 34, 3487.
Bansal, D., Yadav, K., Pandey, V., Ganeshpurkar, A., Agnihotri, A., and Dubey, N. (2016). Lactobionic acid coupled liposomes: An innovative strategy for targeting hepatocellular carcinoma. Drug Deliv. (Lond). 23, 140–146. doi:10.3109/10717544.2014.907373
Bansal, S. A., Kumar, V., Karimi, J., and Singh, A. P. (2020). Role of gold nanoparticles in advanced biomedical applications. Nanoscale Adv. 2, 3764–3787. doi:10.1039/d0na00472c
Banu, H., Sethi, D. K., Edgar, A., Sheriff, A., Rayees, N., Renuka, N., et al. (2015). Doxorubicin loaded polymeric gold nanoparticles targeted to human folate receptor upon laser photothermal therapy potentiates chemotherapy in breast cancer cell lines. J. Photochem. Photobiol. B Biol. 149, 116–128. doi:10.1016/j.jphotobiol.2015.05.008
Bao, W., Ma, H., Wang, N., and He, Z. (2019). pH-sensitive carbon quantum dots− doxorubicin nanoparticles for tumor cellular targeted drug delivery. Polym. Adv. Technol. 30, 2664–2673. doi:10.1002/pat.4696
Beguin, E., Gray, M. D., Logan, K. A., Nesbitt, H., Sheng, Y., Kamila, S., et al. (2020). Magnetic microbubble mediated chemo-sonodynamic therapy using a combined magnetic-acoustic device. J. Control. Release 317, 23–33. doi:10.1016/j.jconrel.2019.11.013
Bharti, C., Nagaich, U., and Pal, A. K. (2015). Mesoporous silica nanoparticles in target drug delivery system: A review. Int. J. Pharm. Investig. 5, 124–133. doi:10.4103/2230-973x.160844
Bhatia, S. (2016). Nanoparticles types, classification, characterization, fabrication methods and drug delivery applications, Nat. Polym. drug Deliv. Syst., 48, 19907. Springer.
Bose, R. J. C., Uday Kumar, S., Zeng, Y., Afjei, R., Robinson, E., Lau, K., et al. (2018). Tumor cell-derived extracellular vesicle-coated nanocarriers: An efficient theranostic platform for the cancer-specific delivery of anti-miR-21 and imaging agents. ACS Nano 12, 10817–10832. doi:10.1021/acsnano.8b02587
Bozzuto, G., and Molinari, A. (2015). Liposomes as nanomedical devices. Int. J. Nanomedicine 10, 975–999. doi:10.2147/ijn.s68861
Brighenti, R., and Li, Y. V. (2020). Smart polymers for advanced applications: A mechanical perspective review. Front. Mat. 7, 196. doi:10.3389/fmats.2020.00196
Bugno, J., Hsu, H. J., and Hong, S. (2015). Tweaking dendrimers and dendritic nanoparticles for controlled nano-bio interactions: Potential nanocarriers for improved cancer targeting. J. Drug Target. 23, 642–650. doi:10.3109/1061186x.2015.1052077
Bulbake, U., Doppalapudi, S., Kommineni, N., and Khan, W. (2017). Liposomal formulations in clinical use: An updated review. Pharmaceutics 9, 12. doi:10.3390/pharmaceutics9020012
Cabral, H., Matsumoto, Y., Mizuno, K., Chen, Q., Murakami, M., Kimura, M., et al. (2011). Accumulation of sub-100 nm polymeric micelles in poorly permeable tumours depends on size. Nat. Nanotechnol. 6, 815–823. doi:10.1038/nnano.2011.166
Cazzamalli, S., Dal Corso, A., Widmayer, F., and Neri, D. (2018). Chemically defined antibody–and small molecule–drug conjugates for in vivo tumor targeting applications: A comparative analysis. J. Am. Chem. Soc. 140, 1617–1621. doi:10.1021/jacs.7b13361
Chabner, B.A., and Roberts, T.G. (2005). Timeline: Chemotherapy and the war on cancer. Nat. Rev. Cancer 5, 65–72. doi:10.1038/nrc1529
Chen, H., and Liu, D. G. (2016a). Endogenous stimuli-responsive nanocarriers for drug delivery. Chem. Lett. 45, 242–249. doi:10.1246/cl.151176
Chen, H., Wang, Z., Zong, S., Chen, P., Zhu, D., Wu, L., et al. (2015a). A graphene quantum dot-based FRET system for nuclear-targeted and real-time monitoring of drug delivery. Nanoscale 7, 15477–15486. doi:10.1039/c5nr03454j
Chen, K. J., Chaung, E. Y., Wey, S. P., Lin, K. J., Cheng, F., Lin, C. C., et al. (2014). Hyperthermia-mediated local drug delivery by a bubble-generating liposomal system for tumor-specific chemotherapy. ACS Nano 8, 5105–5115. doi:10.1021/nn501162x
Chen, K. J., Liang, H. F., Chen, H. L., Wang, Y., Cheng, P. Y., Liu, H. L., et al. (2013). A thermoresponsive bubble-generating liposomal system for triggering localized extracellular drug delivery. ACS Nano 7, 438–446. doi:10.1021/nn304474j
Chen, L., Zhou, X., Nie, W., Zhang, Q., Wang, W., Zhang, Y., et al. (2016b). Multifunctional redox-responsive mesoporous silica nanoparticles for efficient targeting drug delivery and magnetic resonance imaging. ACS Appl. Mat. Interfaces 8, 33829–33841. doi:10.1021/acsami.6b11802
Chen, M., Hu, J., Wang, L., Li, Y., Zhu, C., Chen, C., et al. (2020a). Targeted and redox-responsive drug delivery systems based on carbonic anhydrase IX-decorated mesoporous silica nanoparticles for cancer therapy. Sci. Rep. 10, 14447–14512. doi:10.1038/s41598-020-71071-1
Chen, S. H., Liu, T. I., Chuang, C. L., Chen, H. H., Chiang, W. H., and Chiu, H. C. (2020b). Alendronate/folic acid-decorated polymeric nanoparticles for hierarchically targetable chemotherapy against bone metastatic breast cancer. J. Mat. Chem. B 8, 3789–3800. doi:10.1039/d0tb00046a
Chen, W. H., Luo, G. F., Lei, Q., Jia, H. Z., Hong, S., Wang, Q. R., et al. (2015b). MMP-2 responsive polymeric micelles for cancer-targeted intracellular drug delivery. Chem. Commun. 51, 465–468. doi:10.1039/c4cc07563c
Chen, X., Sun, H., Hu, J., Han, X., Liu, H., and Hu, Y. (2017). Transferrin gated mesoporous silica nanoparticles for redox-responsive and targeted drug delivery. Colloids Surfaces B Biointerfaces 152, 77–84. doi:10.1016/j.colsurfb.2017.01.010
Cheng, G., He, Y., Xie, L., Nie, Y., He, B., Zhang, Z., et al. (2012). Development of a reduction-sensitive diselenide-conjugated oligoethylenimine nanoparticulate system as a gene carrier. Int. J. Nanomedicine 7, 3991–4006. doi:10.2147/ijn.s32961
Cheng, Q., Blais, M. O., Harris, G. M., and Jabbarzadeh, E. (2013a). PLGA-carbon nanotube conjugates for intercellular delivery of caspase-3 into osteosarcoma cells. PLoS One 8, e81947. doi:10.1371/journal.pone.0081947
Cheng, R., Meng, F., Deng, C., Klok, H. A., and Zhong, Z. (2013b). Dual and multi-stimuli responsive polymeric nanoparticles for programmed site-specific drug delivery. Biomaterials 34, 3647–3657. doi:10.1016/j.biomaterials.2013.01.084
Cheng, W., Nie, J., Xu, L., Liang, C., Peng, Y., Liu, G., et al. (2017). pH-sensitive delivery vehicle based on folic acid-conjugated polydopamine-modified mesoporous silica nanoparticles for targeted cancer therapy. ACS Appl. Mat. Interfaces 9, 18462–18473. doi:10.1021/acsami.7b02457
Chowdhuri, A. R., Singh, T., Ghosh, S. K., and Sahu, S. K. (2016). Carbon dots embedded magnetic nanoparticles@ chitosan@ metal organic framework as a nanoprobe for pH sensitive targeted anticancer drug delivery. ACS Appl. Mat. Interfaces 8, 16573–16583. doi:10.1021/acsami.6b03988
Cobley, C. M., Chen, J., Cho, E. C., Wang, L. V., and Xia, Y. (2011). Gold nanostructures: A class of multifunctional materials for biomedical applications. Chem. Soc. Rev. 40, 44–56. doi:10.1039/b821763g
Colilla, M., Izquierdo-Barba, I., and Vallet-Regí, M. (2010). Phosphorus-containing SBA-15 materials as bisphosphonate carriers for osteoporosis treatment. Microporous Mesoporous Mat. 135, 51–59. doi:10.1016/j.micromeso.2010.06.010
Costa, P. M., Bourgognon, M., Wang, J. T., and Al-Jamal, K. T. (2016). Functionalised carbon nanotubes: From intracellular uptake and cell-related toxicity to systemic brain delivery. J. Control. Release 241, 200–219. doi:10.1016/j.jconrel.2016.09.033
Curtis, K. K., Sarantopoulos, J., Northfelt, D. W., Weiss, G. J., Barnhart, K. M., Whisnant, J. K., et al. (2014). Novel LHRH-receptor-targeted cytolytic peptide, EP-100: first-in-human phase I study in patients with advanced LHRH-receptor-expressing solid tumors. Cancer Chemother. Pharmacol. 73, 931–941. doi:10.1007/s00280-014-2424-x
Darvin, P., and Chandrasekharan, A. K. (2019). Introduction to smart drug delivery systems, Patients, 1–9.
Das, S. S., Bharadwaj, P., Bilal, M., Barani, M., Rahdar, A., Taboada, P., et al. (2020). Stimuli-Responsive Polymeric Nanocarriers for Drug Delivery, Imaging, and Theragnosis, 12. Polymers (Basel).
De La Rica, R, Aili, D., and Stevens, M. M. (2012). Enzyme-responsive nanoparticles for drug release and diagnostics. Adv. Drug Deliv. Rev. 64, 967–978. doi:10.1016/j.addr.2012.01.002
Dhanasekaran, S. (2015). SMART drug based targeted delivery: A new paradigm for nanomedicine strategies. J Int. J. Immunother.
Dijoseph, J. F., Armellino, D. C., Boghaert, E. R., Khandke, K., Dougher, M. M., Sridharan, L., et al. (2004). Antibody-targeted chemotherapy with CMC-544: A CD22-targeted immunoconjugate of calicheamicin for the treatment of B-lymphoid malignancies. Blood 103, 1807–1814. doi:10.1182/blood-2003-07-2466
Dilnawaz, F., Singh, A., Mohanty, C., and Sahoo, S. K. (2010). Dual drug loaded superparamagnetic iron oxide nanoparticles for targeted cancer therapy. Biomaterials 31, 3694–3706. doi:10.1016/j.biomaterials.2010.01.057
Dissanayake, S., Denny, W. A., Gamage, S., and Sarojini, V. (2017). Recent developments in anticancer drug delivery using cell penetrating and tumor targeting peptides. J. Control. Release 250, 62–76. doi:10.1016/j.jconrel.2017.02.006
Du, C., Deng, D., Shan, L., Wan, S., Cao, J., Tian, J., et al. (2013). A pH-sensitive doxorubicin prodrug based on folate-conjugated BSA for tumor-targeted drug delivery. Biomaterials 34, 3087–3097. doi:10.1016/j.biomaterials.2013.01.041
Du, J. Z., Du, X. J., Mao, C. Q., and Wang, J. (2011). Tailor-made dual pH-sensitive polymer-doxorubicin nanoparticles for efficient anticancer drug delivery. J. Am. Chem. Soc. 133, 17560–17563. doi:10.1021/ja207150n
Dunn, M. R., Jimenez, R. M., and Chaput, , J. C. J. N. R. C. (2017). Analysis aptamer Discov. Technol. 1, 1–16.
Duong, H. H., and Yung, L. Y. (2013). Synergistic co-delivery of doxorubicin and paclitaxel using multi-functional micelles for cancer treatment. Int. J. Pharm. X. 454, 486–495. doi:10.1016/j.ijpharm.2013.06.017
Dutta, B, Barick, K., and Chassan, P. A. (2021). Recent advances in active targeting of nanomaterials for anticancer drug delivery. Adv. Colloid Interface Sci. 296, 102509. doi:10.1016/j.cis.2021.102509
Eckmann, D. M., Composto, R. J., Tsourkas, A., and Muzykantov, V. R. (2014). Nanogel carrier design for targeted drug delivery. J. Mat. Chem. B 2, 8085–8097. doi:10.1039/c4tb01141d
Estelrich, J., Escribano, E., Queralt, J., and Busquets, M. (2015). Iron oxide nanoparticles for magnetically-guided and magnetically-responsive drug delivery. Int. J. Mol. Sci. 16, 8070–8101. doi:10.3390/ijms16048070
Fan, N. C., Cheng, F. Y., Ho, JaA., and Yeh, C. S. (2012). Photocontrolled targeted drug delivery: Photocaged biologically active folic acid as a light-responsive tumor-targeting molecule. Angew. Chem. Int. Ed. 51, 8806–8810. doi:10.1002/anie.201203339
Fan, R., Chen, C., Hou, H., Chuan, D., Mu, M., Liu, Z., et al. (2021). Tumor acidity and near-infrared light responsive dual drug delivery polydopamine, Based Nanoparticles Chemo-Photothermal Ther., 31, 2009733.
Fang, X., Cheng, X., Zhang, Y., Zhang, L. G., and Keidar, M. (2018). Single-step synthesis of carbon encapsulated magnetic nanoparticles in arc plasma and potential biomedical applications. J. Colloid Interface Sci. 509, 414–421. doi:10.1016/j.jcis.2017.09.015
Farjadian, F., Roointan, A., Mohammadi-Samani, S., and Hosseini, M. (2019). Mesoporous silica nanoparticles: Synthesis, pharmaceutical applications, biodistribution, and biosafety assessment. Chem. Eng. J. 359, 684–705. doi:10.1016/j.cej.2018.11.156
Ferrara, N., Hillan, K. J., Gerber, H. P., and Novotny, W. (2004). Discovery and development of bevacizumab, an anti-VEGF antibody for treating cancer. Nat. Rev. Drug Discov. 3, 391–400. doi:10.1038/nrd1381
Fischer, M., Appelhans, D., Schwarz, S., Klajnert, B., Bryszewska, M., Voit, B., et al. (2010). Influence of surface functionality of poly(propylene imine) dendrimers on protease resistance and propagation of the scrapie prion protein. Biomacromolecules 11, 1314–1325. doi:10.1021/bm100101s
Fu, Z., Li, S., Han, S., Shi, C., and Zhang, Y. (2022). Antibody drug conjugate: The "biological missile" for targeted cancer therapy. Signal Transduct. Target. Ther. 7, 93. doi:10.1038/s41392-022-00947-7
Gao, J., Nesbitt, H., Logan, K., Burnett, K., White, B., Jack, I. G., et al. (2020). An ultrasound responsive microbubble-liposome conjugate for targeted irinotecan-oxaliplatin treatment of pancreatic cancer. Eur. J. Pharm. Biopharm. 157, 233–240. doi:10.1016/j.ejpb.2020.10.012
Gao, W., Ye, G., Duan, X., Yang, X., and Yang, V. C. (2017). Transferrin receptor-targeted pH-sensitive micellar system for diminution of drug resistance and targetable delivery in multidrug-resistant breast cancer. Int. J. Nanomedicine 12, 1047–1064. doi:10.2147/ijn.s115215
Gary-Bobo, M., Hocine, O., Brevet, D., Maynadier, M., Raehm, L., Richeter, S., et al. (2012). Cancer therapy improvement with mesoporous silica nanoparticles combining targeting, drug delivery and PDT. Int. J. Pharm. X. 423, 509–515. doi:10.1016/j.ijpharm.2011.11.045
Ghiasikhou, S., Cazzamalli, S., Scheuermann, J., Neri, D., and Zenobi, R. (2019). Automated and enhanced extraction of a small molecule-drug conjugate using an enzyme-inhibitor interaction based SPME tool followed by direct analysis by ESI-MS. Anal. Bioanal. Chem. 411, 7387–7398. doi:10.1007/s00216-019-02165-7
Gregoriadis, G., Leathwood, P. D., and Dryman, B. E. (1971). Enzyme entrapment in liposomes. FEBS Lett. 14, 95–99. doi:10.1016/0014-5793(71)80109-6
Gui, C., Zhao, E., Kwok, R. T. K., Leung, A. C. S., Lam, J. W. Y., Jiang, M., et al. (2017). AIE-Active theranostic system: Selective staining and killing of cancer cells. Chem. Sci. 8, 1822–1830. doi:10.1039/c6sc04947h
Guo, J., Gao, X., Su, L., Xia, H., Gu, G., Pang, Z., et al. (2011). Aptamer-functionalized PEG-PLGA nanoparticles for enhanced anti-glioma drug delivery. Biomaterials 32, 8010–8020. doi:10.1016/j.biomaterials.2011.07.004
Guo, L., Shi, D., Shang, M., Sun, X., Meng, D., Liu, X., et al. (2022). Utilizing RNA nanotechnology to construct negatively charged and ultrasound-responsive nanodroplets for targeted delivery of siRNA. Drug Deliv. (Lond). 29, 316–327. doi:10.1080/10717544.2022.2026532
Guo, X., Cheng, Y., Zhao, X., Luo, Y., Chen, J., and Yuan, W. E. (2018). Advances in redox-responsive drug delivery systems of tumor microenvironment. J. Nanobiotechnology 16, 74. doi:10.1186/s12951-018-0398-2
Guo, X., Wang, L., Wei, X., and Zhou, S. (2016). Polymer-based drug delivery systems for cancer treatment. J. Polym. Sci. Part A Polym. Chem. 54, 3525–3550. doi:10.1002/pola.28252
Han, H., Valdepérez, D., Jin, Q., Yang, B., Li, Z., Wu, Y., et al. (2017). Dual enzymatic reaction-assisted gemcitabine delivery systems for programmed pancreatic cancer therapy. ACS Nano 11, 1281–1291. doi:10.1021/acsnano.6b05541
Hanahan, D. Weinberg, (2011). Hallmarks of cancer: The next generation. Cell. 144, 646–674. doi:10.1016/j.cell.2011.02.013
He, Q., Zhang, J., Shi, J., Zhu, Z., Zhang, L., Bu, W., et al. (2010). The effect of PEGylation of mesoporous silica nanoparticles on nonspecific binding of serum proteins and cellular responses. Biomaterials 31, 1085–1092. doi:10.1016/j.biomaterials.2009.10.046
Hibino, M., Tanaka, K., Ouchi, M., and Terashima, T. (2021). Amphiphilic random-block copolymer micelles in water: Precise and dynamic self-assembly controlled by random copolymer association. Macromolecules 55, 178–189. doi:10.1021/acs.macromol.1c02186
Hori, S. I., Herrera, A., Rossi, J. J., and Zhou, J. (2018). Current advances in aptamers for cancer diagnosis and therapy, Basel Cancers, 10.
Hossen, S., Hossain, M. K., Basher, M. K., Mia, M., Rahman, M., and Uddin, M. J. (2019). Smart nanocarrier-based drug delivery systems for cancer therapy and toxicity studies: A review. J. Adv. Res. 15, 1–18. doi:10.1016/j.jare.2018.06.005
Hu, J., Fu, S., Peng, Q., Han, Y., Xie, J., Zan, N., et al. (2017). Paclitaxel-loaded polymeric nanoparticles combined with chronomodulated chemotherapy on lung cancer: In vitro and in vivo evaluation. Int. J. Pharm. X. 516, 313–322. doi:10.1016/j.ijpharm.2016.11.047
Hu, Y., Duan, J., Zhan, Q., Wang, F., Lu, X., and Yang, X. D. (2012). Novel MUC1 aptamer selectively delivers cytotoxic agent to cancer cells in vitro. PLoS One 7, e31970. doi:10.1371/journal.pone.0031970
Iacobazzi, R. M., Porcelli, L., Lopedota, A. A., Laquintana, V., Lopalco, A., Cutrignelli, A., et al. (2017). Targeting human liver cancer cells with lactobionic acid-G (4)-PAMAM-FITC sorafenib loaded dendrimers. Int. J. Pharm. X. 528, 485–497. doi:10.1016/j.ijpharm.2017.06.049
Iannazzo, D., Pistone, A., Salamo, M., Galvagno, S., Romeo, R., Giofre, S. V., et al. (2017). Graphene quantum dots for cancer targeted drug delivery. Int. J. Pharm. X. 518, 185–192. doi:10.1016/j.ijpharm.2016.12.060
Indermun, S., Govender, M., Kumar, P., Choonara, Y. E., and Pillay, V. (2018). Stimuli-responsive polymers as smart drug delivery systems: Classifications based on carrier type and triggered-release mechanism, Stimuli Responsive Polym. Nanocarriers Drug Deliv. Appl., Volume 1. Elsevier.
Jablonowski, L. J., Conover, D., Teraphongphom, N. T., and Wheatley, M. A. (2018). Manipulating multifaceted microbubble shell composition to target both TRAIL-sensitive and resistant cells. J. Biomed. Mat. Res. A 106, 1903–1915. doi:10.1002/jbm.a.36389
Jain, A. K., Dubey, V., Mehra, N. K., Lodhi, N., Nahar, M., Mishra, D. K., et al. (2009). Carbohydrate-conjugated multiwalled carbon nanotubes: Development and characterization. Nanomedicine Nanotechnol. Biol. Med. 5, 432–442. doi:10.1016/j.nano.2009.03.001
Jiang, W., Kim, B. Y., Rutka, J. T., and Chan, W. C. W. (2008). Nanoparticle-mediated cellular response is size-dependent. Nat. Nanotechnol. 3, 145–150. doi:10.1038/nnano.2008.30
Jin, M., Jin, G., Kang, L., Chen, L., Gao, Z., and Huang, W. (2018). Smart polymeric nanoparticles with pH-responsive and PEG-detachable properties for co-delivering paclitaxel and survivin siRNA to enhance antitumor outcomes. Int. J. Nanomedicine 13, 2405–2426. doi:10.2147/ijn.s161426
Jin, X., Zhang, J., Jin, X., Liu, L., and Tian, X. (2020). Folate receptor targeting and cathepsin B-sensitive drug delivery system for selective cancer cell death and imaging. ACS Med. Chem. Lett. 11, 1514–1520. doi:10.1021/acsmedchemlett.0c00031
Jin, Y., Liang, X., An, Y., and Dai, Z. (2016). Microwave-triggered smart drug release from liposomes Co-encapsulating doxorubicin and salt for local combined hyperthermia and chemotherapy of cancer. Bioconjug. Chem. 27, 2931–2942. doi:10.1021/acs.bioconjchem.6b00603
Joglekar, M., and Trewyn, B. G. (2013). Polymer-based stimuli-responsive nanosystems for biomedical applications. Biotechnol. J. 8, 931–945. doi:10.1002/biot.201300073
Jung, S. H., Na, K., Lee, S. A., Cho, S. H., Seong, H., and Shin, B. C. (2012). Gd(III)-DOTA-modified sonosensitive liposomes for ultrasound-triggered release and MR imaging. Nanoscale Res. Lett. 7, 462. doi:10.1186/1556-276x-7-462
Kaiser, P. K. (2006). Antivascular endothelial growth factor agents and their development: Therapeutic implications in ocular diseases. Am. J. Ophthalmol. 142, 660–668. doi:10.1016/j.ajo.2006.05.061
Kaminskas, L. M., Mcleod, V. M., Ryan, G. M., Kelly, B. D., Haynes, J. M., Williamson, M., et al. (2014). Pulmonary administration of a doxorubicin-conjugated dendrimer enhances drug exposure to lung metastases and improves cancer therapy. J. Control. Release 183, 18–26. doi:10.1016/j.jconrel.2014.03.012
Kang, M. H., Yoo, H. J., Kwon, Y. H., Yoon, H. Y., Lee, S. G., Kim, S. R., et al. (2015). Design of multifunctional liposomal nanocarriers for folate receptor-specific intracellular drug delivery. Mol. Pharm. 12, 4200–4213. doi:10.1021/acs.molpharmaceut.5b00399
Kaul, G., and Amiji, M. (2002). Long-circulating poly(ethylene glycol)-modified gelatin nanoparticles for intracellular delivery. Pharm. Res. 19, 1061–1067. doi:10.1023/a:1016486910719
Ke, W., Zha, Z., Mukerabigwi, J. F., Chen, W., Wang, Y., He, C., et al. (2017). Matrix metalloproteinase-responsive multifunctional peptide-linked amphiphilic block copolymers for intelligent systemic anticancer drug delivery. Bioconjug. Chem. 28, 2190–2198. doi:10.1021/acs.bioconjchem.7b00330
Ke, X. Y., Lin Ng, V. W., Gao, S. J., Tong, Y. W., Hedrick, J. L., and Yang, Y. Y. (2014). Co-delivery of thioridazine and doxorubicin using polymeric micelles for targeting both cancer cells and cancer stem cells. Biomaterials 35, 1096–1108. doi:10.1016/j.biomaterials.2013.10.049
Kenchegowda, M., Rahamathulla, M., Hani, U., Begum, M. Y., Guruswamy, S., Osmani, R. A. M., et al. (2021). Smart nanocarriers as an emerging platform for cancer therapy: A review. Molecules 27, 146. doi:10.3390/molecules27010146
Kessenbrock, K., and Plaks, V. W. (2010). Matrix metalloproteinases: Regulators of the tumor microenvironment. Cell. 141, 52–67. doi:10.1016/j.cell.2010.03.015
Khazaei, Z., Namayandeh, S. M., Beiranvand, R., Naemi, H., Bechashk, S. M., and Goodarzi, E. (2021). Worldwide incidence and mortality of ovarian cancer and human development index (HDI): GLOBOCAN sources and methods 2018. J. Prev. Med. Hyg. 62, E174–E184. doi:10.15167/2421-4248/jpmh2021.62.1.1606
Kim, D-H., Vitol, E. A., Liu, J., Balasubramanian, S., Gosztola, D. J., Cohen, E. E., et al. (2013). Stimuli-responsive magnetic nanomicelles as multifunctional heat and cargo delivery vehicles. Langmuir 29, 7425–7432. doi:10.1021/la3044158
Kim, M. W., Kwon, S. H., Choi, J. H., and Lee, A. (2018). A promising biocompatible platform: Lipid-based and bio-inspired smart drug delivery systems for cancer therapy. Int. J. Mol. Sci. 19, 3859. doi:10.3390/ijms19123859
Kimiz-Gebologlu, I., and Sbiray-Avci, C. (2018). Monoclonal antibodies in cancer immunotherapy. Mol. Biol. Rep. 45, 2935–2940. doi:10.1007/s11033-018-4427-x
Kommareddy, S., and Samiji, M. (2007). Poly(ethylene glycol)-modified thiolated gelatin nanoparticles for glutathione-responsive intracellular DNA delivery. Nanomedicine Nanotechnol. Biol. Med. 3, 32–42. doi:10.1016/j.nano.2006.11.005
Koren, E., Apte, A., Jani, A., and Torchilin, V. P. (2012). Multifunctional PEGylated 2C5-immunoliposomes containing pH-sensitive bonds and TAT peptide for enhanced tumor cell internalization and cytotoxicity. J. Control. Release 160, 264–273. doi:10.1016/j.jconrel.2011.12.002
Kubler, E., and Albrecht, H. (2018). Large set data mining reveals overexpressed GPCRs in prostate and breast cancer: Potential for active targeting with engineered anti-cancer nanomedicines. Oncotarget 9, 24882–24897. doi:10.18632/oncotarget.25427
Kue, C. S., Kamkaew, A., Burgess, K., Kiew, L. V., Chung, L. Y., and Lee, H. B. (2016). Small molecules for active targeting in cancer. Med. Res. Rev. 36, 494–575. doi:10.1002/med.21387
Lale, S. V., Rg, A., Aravind, A., Kumar, D. S., and Koul, V. (2014). AS1411 aptamer and folic acid functionalized pH-responsive ATRP fabricated pPEGMA–PCL–pPEGMA polymeric nanoparticles for targeted drug delivery in cancer therapy. Biomacromolecules 15, 1737–1752. doi:10.1021/bm5001263
Lallana, E., and Tirelli, N. J. M. C. P. (2013). Oxidation-responsive polymers: Which groups to use, how to make them, what to expect from them (biomedical applications). Macromol. Chem. Phys. 214, 143–158. doi:10.1002/macp.201200502
Leamon, C. P., Reddy, J. A., Vlahov, I. R., Kleindl, P. J., Vetzel, M., and Westrick, E. (2006). Synthesis and biological evaluation of EC140: A novel folate-targeted vinca alkaloid conjugate. Bioconjug. Chem. 17, 1226–1232. doi:10.1021/bc060145g
Leamon, C. P., and Jackman, A. L. (2008). Exploitation of the folate receptor in the management of cancer and inflammatory disease. Vitam. Horm. 79, 203–233. doi:10.1016/S0083-6729(08)00407-X
Lee, B. K., Yun, Y. H., and Park, K. (2015). Smart nanoparticles for drug delivery: Boundaries and opportunities. Chem. Eng. Sci. 125, 158–164. doi:10.1016/j.ces.2014.06.042
Lee, Y., and Thompson, D. H. (2017). Stimuli-responsive liposomes for drug delivery. Wiley Interdiscip. Rev. Nanomed Nanobiotechnol, 9.
Li, M., Bu, W., Ren, J., Li, J., Deng, L., Gao, M., et al. (2018). Enhanced synergism of thermo-chemotherapy for liver cancer with magnetothermally responsive nanocarriers. Theranostics 8, 693–709. doi:10.7150/thno.21297
Li Volsi, A., Scialabba, C., Vetri, V., Cavallaro, G., Licciardi, M., and Giammona, G. (2017). Near-infrared light responsive folate targeted gold nanorods for combined photothermal-chemotherapy of osteosarcoma. ACS Appl. Mat. Interfaces 9, 14453–14469. doi:10.1021/acsami.7b03711
Li, Y., Jiang, C., Zhang, D., Wang, Y., Ren, X., Ai, K., et al. (2017a). Targeted polydopamine nanoparticles enable photoacoustic imaging guided chemo-photothermal synergistic therapy of tumor. Acta Biomater. 47, 124–134. doi:10.1016/j.actbio.2016.10.010
Li, Z., De Barros, A. L. B., Soares, D., and Alisaraie, L. (2017b). Functionalized single-walled carbon nanotubes: Cellular uptake, biodistribution and applications in drug delivery. Int. J. Pharm. X. 524, 41–54. doi:10.1016/j.ijpharm.2017.03.017
Li, Z-Y., Hu, J-J., Xu, Q., Chen, S., Jia, H. Z., Sun, Y. X., et al. (2015). A redox-responsive drug delivery system based on RGD containing peptide-capped mesoporous silica nanoparticles. J. Mat. Chem. B 3, 39–44. doi:10.1039/c4tb01533a
Lin, X., Cao, Y., Li, J., Zheng, D., Lan, S., Xue, Y., et al. (2019). Folic acid-modified Prussian blue/polydopamine nanoparticles as an MRI agent for use in targeted chemo/photothermal therapy. Biomater. Sci. 7, 2996–3006. doi:10.1039/c9bm00276f
Liu, D., Yang, F., Xiong, F., and Gu, N. (2016). The smart drug delivery system and its clinical potential. Theranostics 6, 1306–1323. doi:10.7150/thno.14858
Liu, L., Wei, Y., Zhai, S., Chen, Q., and Xing, D. (2015). Dihydroartemisinin and transferrin dual-dressed nano-graphene oxide for a pH-triggered chemotherapy, Drug, 62, 35–46.
Liu, M., Du, H., Zhang, W., and Zhai, G. (2017). Internal stimuli-responsive nanocarriers for drug delivery: Design strategies and applications. Mater. Sci. Eng. C 71, 1267–1280. doi:10.1016/j.msec.2016.11.030
Liu, Z., Chen, K., Davis, C., Sherlock, S., Cao, Q., Chen, X., et al. (2008). Drug delivery with carbon nanotubes for in vivo cancer treatment. Cancer Res. 68, 6652–6660. doi:10.1158/0008-5472.can-08-1468
Lo, C. F., Chiu, T. Y., Liu, Y. T., Pan, P. Y., Liu, K. L., Hsu, C. Y., et al. (2022). Targeting the phosphatidylserine-immune checkpoint with a small-molecule maytansinoid conjugate. J. Med. Chem. 65, 12802–12824. doi:10.1021/acs.jmedchem.2c00631
Lu, Y-J., Wei, K-C., Ma, C-C. M., Yang, S. Y., and Chen, J. P. (2012). Dual targeted delivery of doxorubicin to cancer cells using folate-conjugated magnetic multi-walled carbon nanotubes. Colloids Surfaces B Biointerfaces 89, 1–9. doi:10.1016/j.colsurfb.2011.08.001
Luo, Z., Jin, K., Pang, Q., Shen, S., Yan, Z., Jiang, T., et al. (2017). On-demand drug release from dual-targeting small nanoparticles triggered by high-intensity focused ultrasound enhanced glioblastoma-targeting therapy. ACS Appl. Mat. Interfaces 9, 31612–31625. doi:10.1021/acsami.7b10866
Madaan, K., Kumar, S., Poonia, N., and Lather, V. (2014). Dendrimers in drug delivery and targeting: Drug-dendrimer interactions and toxicity issues. J. Pharm. Bioallied Sci. 6, 139–150. doi:10.4103/0975-7406.130965
Maeda, H., Wu, J., Sawa, T., Matsumura, Y., and Hori, K. (2000). Tumor vascular permeability and the EPR effect in macromolecular therapeutics: A review. J. Control. Release 65, 271–284. doi:10.1016/s0168-3659(99)00248-5
Maghsoudi, S., Shahraki, B. T., Rabiee, N., Afshari, R., Fatahi, Y., Dinarvand, R., et al. (2019). Recent advancements in aptamer-bioconjugates: Sharpening stones for breast and prostate cancers targeting. J. Drug Deliv. Sci. Technol. 53, 101146. doi:10.1016/j.jddst.2019.101146
Majumder, J., and Minko, T. (2021). Multifunctional and stimuli-responsive nanocarriers for targeted therapeutic delivery. Expert Opin. Drug Deliv. 18, 205–227. doi:10.1080/17425247.2021.1828339
Mao, J. J., Pillai, G. G., Andrade, C. J., Ligibel, J. A., Basu, P., Cohen, L., et al. (2022). Integrative oncology: Addressing the global challenges of cancer prevention and treatment. Ca. A Cancer J. Clin. 72, 144–164. doi:10.3322/caac.21706
Mazzotta, E., Tavano, L., and Muzzalupo, R. (2018). Thermo-sensitive vesicles in controlled drug delivery for chemotherapy. Pharmaceutics 10, 150. doi:10.3390/pharmaceutics10030150
Mehra, N. K., and Jain, N. K. (2015). One platform comparison of estrone and folic acid anchored surface engineered MWCNTs for doxorubicin delivery. Mol. Pharm. 12, 630–643. doi:10.1021/mp500720a
Mi, P. (2020). Stimuli-responsive nanocarriers for drug delivery, tumor imaging, therapy and theranostics. Theranostics 10, 4557–4588. doi:10.7150/thno.38069
Mousavi, T., Hadizadeh, N., Nikfar, S., and Abdollahi, M. (2020). Drug discovery strategies for modulating oxidative stress in gastrointestinal disorders. Expert Opin. Drug Discov. 15, 1309–1341. doi:10.1080/17460441.2020.1791077
Mura, S., Nicolas, J., and Couvreur, P. (2013). Stimuli-responsive nanocarriers for drug delivery. Nat. Mat. 12, 991–1003. doi:10.1038/nmat3776
Murugan, B., Sagadevan, S., Fatimah, I., Oh, W. C., Motalib Hossain, M. A., and Johan, M. R. (2021). Smart stimuli-responsive nanocarriers for the cancer therapy–nanomedicine. Nanotechnol. Rev. 10, 933–953. doi:10.1515/ntrev-2021-0067
Nanjwade, B. K., Bechra, H. M., Derkar, G. K., Manvi, F., and Nanjwade, V. K. (2009). Dendrimers: Emerging polymers for drug-delivery systems. Eur. J. Pharm. Sci. 38, 185–196. doi:10.1016/j.ejps.2009.07.008
Navya, P., Kaphle, A. D., and Hjn, (2018). Nanomedicine in sensing, delivery, imaging and tissue engineering: Advances, opportunities and challenges, Sensing, 30–56.
Naziris, N., Pippa, N., Pispas, S., and Demetzos, C. (2016). Stimuli-responsive drug delivery nanosystems: From bench to clinic. 6, 166–185. doi:10.2174/2468187306666160712232449
Ni, S., Zhuo, Z., Pan, Y., Yu, Y., Li, F., Liu, J., et al. (2021). Recent progress in aptamer discoveries and modifications for therapeutic applications. ACS Appl. Mat. Interfaces 13, 9500–9519. doi:10.1021/acsami.0c05750
Nie, S. (2010). Understanding and overcoming major barriers in cancer nanomedicine. Nanomedicine (Lond) 5, 523–528. doi:10.2217/nnm.10.23
Nimjee, S. M., White, R. R., Becker, R. C., and Sullenger, B. A. (2017). Aptamers as therapeutics. Annu. Rev. Pharmacol. Toxicol. 57, 61–79. doi:10.1146/annurev-pharmtox-010716-104558
Nkepang, G., Bio, M., Rajaputra, P., Awuah, S. G., and You, Y. (2014). Folate receptor-mediated enhanced and specific delivery of far-red light-activatable prodrugs of combretastatin A-4 to FR-positive tumor. Bioconjug. Chem. 25, 2175–2188. doi:10.1021/bc500376j
Noble, G. T., Stefanick, J. F., Ashley, J. D., Kiziltepe, T., and Bilgicer, B. (2014). Ligand-targeted liposome design: Challenges and fundamental considerations. Trends Biotechnol. 32, 32–45. doi:10.1016/j.tibtech.2013.09.007
Norsworthy, K. J., Ko, C. W., Lee, J. E., Liu, J., John, C. S., Przepiorka, D., et al. (2018). FDA approval summary: Mylotarg for treatment of patients with relapsed or refractory CD33-positive acute myeloid leukemia. Oncologist 23, 1103–1108. doi:10.1634/theoncologist.2017-0604
O'reilly, R. K., Hawker, C. J., and Wooley, K. L. (2006). Cross-linked block copolymer micelles: Functional nanostructures of great potential and versatility. Chem. Soc. Rev. 35, 1068–1083. doi:10.1039/b514858h
Olerile, L. D., Liu, Y., Zhang, B., Wang, T., Mu, S., Zhang, J., et al. (2017). Near-infrared mediated quantum dots and paclitaxel co-loaded nanostructured lipid carriers for cancer theragnostic. Colloids Surfaces B Biointerfaces 150, 121–130. doi:10.1016/j.colsurfb.2016.11.032
Palanikumar, L., Al-Hosani, S., Kalmouni, M., Nguyen, V. P., Ali, L., Pasricha, R., et al. (2020). pH-responsive high stability polymeric nanoparticles for targeted delivery of anticancer therapeutics. Commun. Biol. 3, 95–17. doi:10.1038/s42003-020-0817-4
Pan, P., Svirskis, D., Rees, S. W. P., Barker, D., Waterhouse, G. I., and Wu, Z. (2021). Photosensitive drug delivery systems for cancer therapy: Mechanisms and applications. J. Control. Release 338, 446–461. doi:10.1016/j.jconrel.2021.08.053
Pan, Y. J., Chen, Y. Y., Wang, D. R., Wei, C., Guo, J., Lu, D. R., et al. (2012). Redox/pH dual stimuli-responsive biodegradable nanohydrogels with varying responses to dithiothreitol and glutathione for controlled drug release. Biomaterials 33, 6570–6579. doi:10.1016/j.biomaterials.2012.05.062
Panieri, E., and Santoro, M. M. (2016). ROS homeostasis and metabolism: A dangerous liason in cancer cells. Cell. Death Dis. 7, e2253. doi:10.1038/cddis.2016.105
Parida, S., Maiti, C., Rajesh, Y., Dey, K. K., Pal, I., Parekh, A., et al. (2017). Gold nanorod embedded reduction responsive block copolymer micelle-triggered drug delivery combined with photothermal ablation for targeted cancer therapy. Biochimica Biophysica Acta - General Subj. 1861, 3039–3052. doi:10.1016/j.bbagen.2016.10.004
Patel, T. K., Adhikari, N., Amin, S. A., Biswas, S., Jha, T., and Ghosh, B. (2021). Small molecule drug conjugates (SMDCs): An emerging strategy for anticancer drug design and discovery. New J. Chem. 45, 5291–5321. doi:10.1039/d0nj04134c
Peddada, L. Y., Garbuzenko, O. B., Devore, D. I., Minko, T., and Roth, C. M. (2014). Delivery of antisense oligonucleotides using poly(alkylene oxide)-poly(propylacrylic acid) graft copolymers in conjunction with cationic liposomes. J. Control. Release 194, 103–112. doi:10.1016/j.jconrel.2014.08.023
Peer, D., Karp, J. M., Hong, S., Farokhzad, O. C., Margalit, R., and Langer, R. (2007). Nanocarriers as an emerging platform for cancer therapy. Nat. Nanotechnol. 2, 751–760. doi:10.1038/nnano.2007.387
Perez, H., and Fernandez-Medarde, A. (2015). Advanced targeted therapies in cancer: Drug nanocarriers, the future of chemotherapy. Eur. J. Pharm. Biopharm. 93, 52–79. doi:10.1016/j.ejpb.2015.03.018
Piccart-Gebhart, M. J., Procter, M., Leyland-Jones, B., Goldhirsch, A., Untch, M., Smith, I., et al. (2005). Trastuzumab after adjuvant chemotherapy in HER2-positive breast cancer. N. Engl. J. Med. Overseas. Ed. 353, 1659–1672. doi:10.1056/nejmoa052306
Prabhu, R. H., and Patravale, V. B. J. (2015). Polymeric nanoparticles for targeted treatment in oncology: Current insights. Int. J. Nanomedicine 10, 1001–1018. doi:10.2147/ijn.s56932
Prasanna, A., Pooja, R., Suchithra, V., Ravikumar, A., Gupta, P. K., Niranjan, V., et al. (2018). Smart drug delivery systems for cancer treatment using nanomaterials, System, 5, 21047–21054.
Purushothaman, B. K., Maheswari, P., and Usheriffa Begum, K., (2021). pH and magnetic field responsive protein-inorganic nanohybrid conjugated with biotin: A biocompatible carrier system targeting lung cancer cells. J. Appl. Polym. Sci. 138, 49949. doi:10.1002/app.49949
Qian, W., Murakami, M., Ichikawa, Y., and Che, Y. (2011). Highly efficient and controllable PEGylation of gold nanoparticles prepared by femtosecond laser ablation in water. J. Phys. Chem. C 115, 23293–23298. doi:10.1021/jp2079567
Qiu, J., Zhang, R., Li, J., Sang, Y., Tang, W., Rivera Gil, P., et al. (2015). Fluorescent graphene quantum dots as traceable, pH-sensitive drug delivery systems. Int. J. Nanomedicine 10, 6709–6724. doi:10.2147/ijn.s91864
Rabanel, J. M., Aoun, V., Elkin, I., Mokhtar, M., and Hildgen, P. (2012). Drug-loaded nanocarriers: Passive targeting and crossing of biological barriers. Curr. Med. Chem. 19, 3070–3102. doi:10.2174/092986712800784702
Radhakrishnan, K., Tripathy, J., Gnanadhas, D. P., Chakravortty, D., and Raichur, A. M. (2014). Dual enzyme responsive and targeted nanocapsules for intracellular delivery of anticancer agents. RSC Adv. 4, 45961–45968. doi:10.1039/c4ra07815b
Rahamathulla, M., Bhosale, R. R., Osmani, RaM., Mahima, K. C., Johnson, A. P., Hani, U., et al. (2021). Carbon nanotubes: Current perspectives on diverse applications in targeted drug delivery and therapies, Mater. (Basel), 14, 3452.
Rai, S., Singh, N., and Bhattacharya, S. (2022). Concepts on smart nano-based drug delivery system. Recent Pat. Nanotechnol. 16, 67–89. doi:10.2174/1872210515666210120113738
Rajasekhar Reddy, R., Raghupathi, K. R., Torres, D. A., and Thayumanavan, S. (2012). Stimuli sensitive amphiphilic dendrimers. New J. Chem. 36, 340–349. doi:10.1039/c2nj20879b
Rana, A., and Bhatnagar, S. (2021). Advancements in folate receptor targeting for anti-cancer therapy: A small molecule-drug conjugate approach. Bioorg. Chem. 112, 104946. doi:10.1016/j.bioorg.2021.104946
Ravar, F., Saadat, E., Gholami, M., Dehghankelishadi, P., Mahdavi, M., Azami, S., et al. (2016). Hyaluronic acid-coated liposomes for targeted delivery of paclitaxel, in-vitro characterization and in-vivo evaluation. J. Control. Release 229, 10–22. doi:10.1016/j.jconrel.2016.03.012
Reddy, J. A., Dorton, R., Bloomfield, A., Nelson, M., Dircksen, C., Vetzel, M., et al. (2018). Pre-clinical evaluation of EC1456, a folate-tubulysin anti-cancer therapeutic. Sci. Rep. 8, 8943. doi:10.1038/s41598-018-27320-5
Reddy, J. A., Dorton, R., Westrick, E., Dawson, A., Smith, T., Xu, L. C., et al. (2007). Preclinical evaluation of EC145, a folate-vinca alkaloid conjugate, Evaluation, 67, 4434–4442.
Ritchie, M., Tchistiakova, L., and Scott, N. (2013). Implications of receptor-mediated endocytosis and intracellular trafficking dynamics in the development of antibody drug conjugates. MAbs 5, 13–21. doi:10.4161/mabs.22854
Ruoslahti, E. (2012). Peptides as targeting elements and tissue penetration devices for nanoparticles. Adv. Mat. 24, 3747–3756. doi:10.1002/adma.201200454
Sabir, F., Qindeel, M., Zeeshan, M., Ul Ain, Q., Rahdar, A., Barani, M., et al. (2021). Onco-receptors targeting in lung cancer via application of surface-modified and hybrid nanoparticles: A cross-disciplinary review. Process. (Basel). 9, 621. doi:10.3390/pr9040621
Saha, B., Choudhury, N., Seal, S., Ruidas, B., and De, P. (2019). Aromatic nitrogen mustard-based autofluorescent amphiphilic brush copolymer as pH-responsive drug delivery vehicle. Biomacromolecules 20, 546–557. doi:10.1021/acs.biomac.8b01468
Saha, S., Majumdar, R., Hussain, A., Dighe, R. R., and Chakravarty, A. R. (2013). Biotin-conjugated tumour-targeting photocytotoxic iron(III) complexes. Phil. Trans. R. Soc. A 371, 20120190. doi:10.1098/rsta.2012.0190
Sanadgol, N., and Wackerlig, J. (2020). Developments of smart drug-delivery systems based on magnetic molecularly imprinted polymers for targeted cancer therapy: A short review. Pharmaceutics 12, 831. doi:10.3390/pharmaceutics12090831
Sanchez-Moreno, P., Ortega-Vinuesa, J. L., Peula-Garcia, J. M., Marchal, J. A., and Boulaiz, H. (2018). Smart drug-delivery systems for cancer nanotherapy. Curr. Drug Targets 19, 339–359. doi:10.2174/1389450117666160527142544
Sanchis, A., and Salvador, J. P. M. (2019). Light-induced mechanisms for nanocarrier's cargo release. Colloids Surfaces B Biointerfaces 173, 825–832. doi:10.1016/j.colsurfb.2018.10.056
Sapra, P., and Allen, T. M. (2003). Ligand-targeted liposomal anticancer drugs. Prog. Lipid Res. 42, 439–462. doi:10.1016/s0163-7827(03)00032-8
Satsangi, A., Roy, S. S., Satsangi, R. K., Tolcher, A. W., Vadlamudi, R. K., Goins, B., et al. (2015). Synthesis of a novel, sequentially active-targeted drug delivery nanoplatform for breast cancer therapy. Biomaterials 59, 88–101. doi:10.1016/j.biomaterials.2015.03.039
Sawant, R. R., and Torchilin, V. P. (2012). Challenges in development of targeted liposomal therapeutics. AAPS J. 14, 303–315. doi:10.1208/s12248-012-9330-0
Schmaljohann, D. (2006). Thermo- and pH-responsive polymers in drug delivery. Adv. Drug Deliv. Rev. 58, 1655–1670. doi:10.1016/j.addr.2006.09.020
Senapati, S., Mahanta, A. K., Kumar, S., and Maiti, P. (2018). Controlled drug delivery vehicles for cancer treatment and their performance. Signal Transduct. Target. Ther. 3, 7. doi:10.1038/s41392-017-0004-3
Seo, S. J., Lee, S. Y., Choi, S. J., and Kim, H. W. (2015). Tumor-targeting Co-delivery of drug and gene from temperature-triggered micelles. Macromol. Biosci. 15, 1198–1204. doi:10.1002/mabi.201500137
Sershen, S., and West, J. (2002). Implantable, polymeric systems for modulated drug delivery. Adv. Drug Deliv. Rev. 54, 1225–1235. doi:10.1016/s0169-409x(02)00090-x
Shen, Y., Li, X., Dong, D., Zhang, B., Xue, Y., and Shang, P. (2018). Transferrin receptor 1 in cancer: A new sight for cancer therapy. Am. J. Cancer Res. 8, 916–931.
Shi, C., Guo, X., Qu, Q., Tang, Z., Wang, Y., and Zhou, S. (2014). Actively targeted delivery of anticancer drug to tumor cells by redox-responsive star-shaped micelles. Biomaterials 35, 8711–8722. doi:10.1016/j.biomaterials.2014.06.036
Shi, J., Kantoff, P. W., Wooster, R., and Farokhzad, O. C. (2017). Cancer nanomedicine: Progress, challenges and opportunities. Nat. Rev. Cancer 17, 20–37. doi:10.1038/nrc.2016.108
Shi, J., Votruba, A. R., Farokhzad, O. C., and Langer, R. (2010). Nanotechnology in drug delivery and tissue engineering: From discovery to applications. Nano Lett. 10, 3223–3230. doi:10.1021/nl102184c
Shi, Z., Zhou, Y., Fan, T., Lin, Y., Zhang, H., and Mei, L. (2020). Inorganic nano-carriers based smart drug delivery systems for tumor therapy. Smart Mat. Med. 1, 32–47. doi:10.1016/j.smaim.2020.05.002
Shirbin, S. J., Ladewig, K., Fu, Q., Klimak, M., Zhang, X., Duan, W., et al. (2015). Cisplatin-induced formation of biocompatible and biodegradable polypeptide-based vesicles for targeted anticancer drug delivery. Biomacromolecules 16, 2463–2474. doi:10.1021/acs.biomac.5b00692
Singh, B., Lohan, S., Sandhu, P. S., Jain, A., and Mehta, S. K. (2016). Functionalized carbon nanotubes and their promising applications in therapeutics and diagnostics, Nanobiomaterials Med. Imaging, 38, 17768. Elsevier.
Singh, S., Kumar, N. K., Dwiwedi, P., Charan, J., Kaur, R., Sidhu, P., et al. (2018). Monoclonal antibodies: A review. Curr. Clin. Pharmacol. 13, 85–99. doi:10.2174/1574884712666170809124728
Sirisha, S. (2020). A review on delivery of anti-cancer drugs by smart nanocarriers: Data obtained from past one decade. Rese. Jour. Pharm. Dosag. Form. Technol. 12, 185–190. doi:10.5958/0975-4377.2020.00032.4
Srinivasan, M., Rajabi, M., and Mousa, S. A. (2015). Multifunctional nanomaterials and their applications in drug delivery and cancer therapy. Nanomater. (Basel) 5, 1690–1703. doi:10.3390/nano5041690
Srinivasarao, M., Galliford, C. V., and Low, P. S. (2015). Principles in the design of ligand-targeted cancer therapeutics and imaging agents. Nat. Rev. Drug Discov. 14, 203–219. doi:10.1038/nrd4519
Srinivasarao, M., and Low, P. S. (2017). Ligand-targeted drug delivery. Chem. Rev. 117, 12133–12164. doi:10.1021/acs.chemrev.7b00013
Subramanian, N., Raghunathan, V., Kanwar, J. R., Kanwar, R. K., Elchuri, S. V., Khetan, V., et al. (2012). Target-specific delivery of doxorubicin to retinoblastoma using epithelial cell adhesion molecule aptamer. Mol. Vis. 18, 2783–2795.
Sun, Q., Bi, H., Wang, Z., Li, C., Wang, X., Xu, J., et al. (2019). Hyaluronic acid-targeted and pH-responsive drug delivery system based on metal-organic frameworks for efficient antitumor therapy. Biomaterials 223, 119473. doi:10.1016/j.biomaterials.2019.119473
Sung, H., Ferlay, J., Siegel, R. L., Laversanne, M., Soerjomataram, I., Jemal, A., et al. (2021). Global cancer statistics 2020: GLOBOCAN estimates of incidence and mortality worldwide for 36 cancers in 185 countries. Ca. A Cancer J. Clin. 71, 209–249. doi:10.3322/caac.21660
Sur, S., Rathore, A., Dave, V., Reddy, K. R., Chouhan, R. S., and Sadhu, V. (2019). Recent developments in functionalized polymer nanoparticles for efficient drug delivery system. Nano-Structures Nano-Objects 20, 100397. doi:10.1016/j.nanoso.2019.100397
Sutton, D., Nasongkla, N., Blanco, E., and Gao, J. (2007). Functionalized micellar systems for cancer targeted drug delivery. Pharm. Res. 24, 1029–1046. doi:10.1007/s11095-006-9223-y
Sztandera, K., Gorzkiewicz, M., and Klajnert-Maculewicz, B. (2019). Gold nanoparticles in cancer treatment. Mol. Pharm. 16, 1–23. doi:10.1021/acs.molpharmaceut.8b00810
Tang, H., Guo, Y., Peng, L., Fang, H., Wang, Z., Zheng, Y., et al. (2018). In vivo targeted, responsive, and synergistic cancer nanotheranostics by magnetic resonance, Imaging-guided Synerg. high-intensity Focus. ultrasound ablation Chemother., 10, 15428–15441.
Teleanu, R. I., Chircov, C., Grumezescu, A. M., and Teleanu, D. M. (2019). Tumor angiogenesis and anti-angiogenic strategies for cancer treatment. J. Clin. Med. 9, 84. doi:10.3390/jcm9010084
Thiviyanathan, V., and Gorenstein, D. G. (2012). Aptamers and the next generation of diagnostic reagents. Prot. Clin. Appl. 6, 563–573. doi:10.1002/prca.201200042
Tian, L., Lu, L., Qiao, Y., Ravi, S., Salatan, F., and Melancon, M. (2016). Stimuli-responsive gold nanoparticles for cancer diagnosis and therapy. J. Funct. Biomater. 7, 19. doi:10.3390/jfb7020019
Tong, J. T. W., Harris, P. W. R., Brimble, M. A., and Kavianinia, I. (2021). An insight into FDA approved antibody-drug conjugates for cancer therapy. Molecules 26, 5847. doi:10.3390/molecules26195847
Torchilin, V. (2012). Liposomes in drug delivery. Fundam. Appl. Control. Release drug Deliv., 45. Springer.
Torchilin, V. P. (2010). Passive and active drug targeting: Drug delivery to tumors as an example. Handb. Exp. Pharmacol., 3–53. doi:10.1007/978-3-642-00477-3_1
Torres, M., Parets, S., Fernandez-Diaz, J., Beteta-Gobel, R., Rodriguez-Lorca, R., Roman, R., et al. (2021). Lipids in Pathophysiology and Development of the Membrane Lipid Therapy: New Bioactive Lipids, 11. Membr. (Basel).
Tortorella, S., and Karagiannis, T. C. (2014). Transferrin receptor-mediated endocytosis: A useful target for cancer therapy. J. Membr. Biol. 247, 291–307. doi:10.1007/s00232-014-9637-0
Trinh, T. L., Zhu, G., Xiao, X., Puszyk, W., Sefah, K., Wu, Q., et al. (2015). A synthetic aptamer-drug adduct for targeted liver cancer therapy. PLoS One 10, e0136673. doi:10.1371/journal.pone.0136673
Ulbrich, K., Hola, K., Subr, V., Bakandritsos, A., Tucek, J., and Zboril, R. (2016). Targeted drug delivery with polymers and magnetic nanoparticles: Covalent and noncovalent approaches, release control, and clinical studies. Chem. Rev. 116, 5338–5431. doi:10.1021/acs.chemrev.5b00589
Vallet-Regi, M., Rámila, A., Del Real, R., and Perez-Pariente, J. (2001). A new property of MCM-41: Drug delivery system. Chem. Mat. 13, 308–311. doi:10.1021/cm0011559
Vines, J. B., Yoon, J. H., Ryu, N. E., Lim, D. J., and Park, H. (2019). Gold nanoparticles for photothermal cancer therapy. Front. Chem. 7, 167. doi:10.3389/fchem.2019.00167
Vives, E., Schmidt, J., and Pelegrin, A. (2008). Cell-penetrating and cell-targeting peptides in drug delivery. Biochimica Biophysica Acta - Rev. Cancer 1786, 126–138. doi:10.1016/j.bbcan.2008.03.001
Vlahov, I. R., Qi, L., Kleindl, P. J., Santhapuram, H. K., Felten, A., Parham, G. L., et al. (2017). Latent warheads for targeted cancer therapy: Design and synthesis of pro-pyrrolobenzodiazepines and conjugates. Bioconjug. Chem. 28, 2921–2931. doi:10.1021/acs.bioconjchem.7b00476
Vlahov, I. R., Santhapuram, H. K. R., Kleindl, P. J., Howard, S. J., Stanford, K. M., and Leamon, C. P. (2006). Design and regioselective synthesis of a new generation of targeted chemotherapeutics. Part 1: EC145, a folic acid conjugate of desacetylvinblastine monohydrazide. Bioorg. Med. Chem. Lett. 16, 5093–5096. doi:10.1016/j.bmcl.2006.07.030
Wang, H., Huang, Q., Chang, H., Xiao, J., and Cheng, Y. (2016). Stimuli-responsive dendrimers in drug delivery. Biomater. Sci. 4, 375–390. doi:10.1039/c5bm00532a
Wang, J., and Jiao, Y. S. (2018). Mesoporous silica nanoparticles for dual-mode chemo-sonodynamic therapy by low-energy ultrasound. Materials 11, 2041. doi:10.3390/ma11102041
Wang, K., Yao, H., Meng, Y., Wang, Y., Yan, X., and Huang, R. (2015). Specific aptamer-conjugated mesoporous silica–carbon nanoparticles for HER2-targeted chemo-photothermal combined therapy. Acta Biomater. 16, 196–205. doi:10.1016/j.actbio.2015.01.002
Wang, T., and Petrenko, V. (2010). Paclitaxel-loaded polymeric micelles modified with MCF-7 cell-specific phage protein: Enhanced binding to target cancer cells and increased cytotoxicity. Mol. Pharm. 7, 1007–1014. doi:10.1021/mp1001125
Wang, W., and Wang, J. D. (2020). Gold nanoparticle-conjugated nanomedicine: Design, construction, and structure-efficacy relationship studies. J. Mat. Chem. B 8, 4813–4830. doi:10.1039/c9tb02924a
Wang, X., Li, S., Shi, Y., Chuan, X., Li, J., Zhong, T., et al. (2014). The development of site-specific drug delivery nanocarriers based on receptor mediation. J. Control. Release 193, 139–153. doi:10.1016/j.jconrel.2014.05.028
Wang, Z., He, Q., Zhao, W., Luo, J., and Gao, W. (2017). Tumor-homing, pH-and ultrasound-responsive polypeptide-doxorubicin nanoconjugates overcome doxorubicin resistance in cancer therapy. J. Control. Release 264, 66–75. doi:10.1016/j.jconrel.2017.08.017
Wen, J., Tao, W., Hao, S., Iyer, S. P., and Zu, Y. (2016). A unique aptamer-drug conjugate for targeted therapy of multiple myeloma. Leukemia 30, 987–991. doi:10.1038/leu.2015.216
Wen, R., Umeano, A. C., Chen, P., and Farooqi, A. A. (2018). Polymer-based drug delivery systems for cancer. Crit. Rev. Ther. Drug Carr. Syst. 35, 521–553. doi:10.1615/critrevtherdrugcarriersyst.2018021124
Wicki, A., Witzigmann, D., Balasubramanian, V., and Huwyler, J. (2015). Nanomedicine in cancer therapy: Challenges, opportunities, and clinical applications. J. Control. Release 200, 138–157. doi:10.1016/j.jconrel.2014.12.030
Wu, G., Fang, Y. Z., Yang, S., Lupton, J. R., and Turner, N. D. (2004). Glutathione metabolism and its implications for health. J. Nutr. 134, 489–492. doi:10.1093/jn/134.3.489
Wu, H. Q., and Wang, C. C. (2016). Biodegradable smart nanogels: A new platform for targeting drug delivery and biomedical diagnostics. Langmuir 32, 6211–6225. doi:10.1021/acs.langmuir.6b00842
Xin, X., Teng, C., Du, X., Lv, Y., Xiao, Q., Wu, Y., et al. (2018). Drug-delivering-drug platform-mediated potent protein therapeutics via a non-endo-lysosomal route. Theranostics 8, 3474–3489. doi:10.7150/thno.23804
Xing, Q., Li, N., Chen, D., Sha, W., Jiao, Y., Qi, X., et al. (2014). Light-responsive amphiphilic copolymer coated nanoparticles as nanocarriers and real-time monitors for controlled drug release. J. Mat. Chem. B 2, 1182–1189. doi:10.1039/c3tb21269f
Xing, Q., Li, N., Jiao, Y., Chen, D., Xu, J., Xu, Q., et al. (2015). Near-infrared light-controlled drug release and cancer therapy with polymer-caged upconversion nanoparticles. RSC Adv. 5, 5269–5276. doi:10.1039/c4ra12678e
Xu, H., and Li, Z. S. (2014). Nanocarriers in gene therapy: A review. J. Biomed. Nanotechnol. 10, 3483–3507. doi:10.1166/jbn.2014.2044
Xu, J., Shao, X., Wei, Y., Xu, F., and Wang, H. (2017a). iTRAQ proteomic analysis reveals that metabolic pathways involving energy metabolism are affected by tea tree oil in botrytis cinerea. Front. Microbiol. 8, 1989. doi:10.3389/fmicb.2017.01989
Xu, W., Qian, J., Hou, G., Suo, A., Wang, Y., Wang, J., et al. (2017b). Hyaluronic acid-functionalized gold nanorods with pH/NIR dual-responsive drug release for synergetic targeted photothermal chemotherapy of breast cancer. ACS Appl. Mat. Interfaces 9, 36533–36547. doi:10.1021/acsami.7b08700
Xu, Y., Jin, X., Zhang, J., Wang, K., Jin, X., Xu, D., et al. (2020). Antitumor activity of a novel double-targeted system for folate receptor-mediated delivery of mitomycin C. ACS Omega 5, 26864–26870. doi:10.1021/acsomega.0c04042
Xue, Y., Bai, H., Peng, B., Fang, B., Baell, J., Li, L., et al. (2021). Stimulus-cleavable chemistry in the field of controlled drug delivery. Chem. Soc. Rev. 50, 4872–4931. doi:10.1039/d0cs01061h
Yan, F., Li, L., Deng, Z., Jin, Q., Chen, J., Yang, W., et al. (2013). Paclitaxel-liposome–microbubble complexes as ultrasound-triggered therapeutic drug delivery carriers. J. Control. Release 166, 246–255. doi:10.1016/j.jconrel.2012.12.025
Yan, H., Teh, C., Sreejith, S., Zhu, L., Kwok, A., Fang, W., et al. (2012). Functional mesoporous silica nanoparticles for photothermal-controlled drug delivery in vivo. Angew. Chem. Int. Ed. 51, 8373–8377. doi:10.1002/anie.201203993
Yang, Y., Wan, J., Niu, Y., Gu, Z., Zhang, J., Yu, M., et al. (2016). Structure-dependent and glutathione-responsive biodegradable dendritic mesoporous organosilica nanoparticles for safe protein delivery. Chem. Mat. 28, 9008–9016. doi:10.1021/acs.chemmater.6b03896
Yao, C., Zhang, L., Wang, J., He, Y., Xin, J., Wang, S., et al. (2016). Gold nanoparticle mediated phototherapy for cancer, Cancer, 28, 895.
Yin, S., Huai, J., Chen, X., Yang, Y., Zhang, X., Gan, Y., et al. (2015). Intracellular delivery and antitumor effects of a redox-responsive polymeric paclitaxel conjugate based on hyaluronic acid. Acta Biomater. 26, 274–285. doi:10.1016/j.actbio.2015.08.029
Yingchoncharoen, P, Kalinowsk, D., and Richardson, D. R. (2016). Lipid-based drug delivery systems in cancer therapy: What is available and what is yet to come. Pharmacol. Rev. 68, 701–787. doi:10.1124/pr.115.012070
Zhang, C., Pan, D., Li, J., Hu, J., Bains, A., Guys, N., et al. (2017). Enzyme-responsive peptide dendrimer-gemcitabine conjugate as a controlled-release drug delivery vehicle with enhanced antitumor efficacy. Acta Biomater. 55, 153–162. doi:10.1016/j.actbio.2017.02.047
Zhang, H., Fan, T., Chen, W., Li, Y., and Wang, B. (2020). Recent advances of two-dimensional materials in smart drug delivery nano-systems. Bioact. Mat. 5, 1071–1086. doi:10.1016/j.bioactmat.2020.06.012
Zhang, J., Song, L., Zhou, S., Hu, M., Jiao, Y., Teng, Y., et al. (2019). Enhanced ultrasound imaging and anti-tumor in vivo properties of Span–polyethylene glycol with folic acid–carbon nanotube–paclitaxel multifunctional microbubbles. RSC Adv. 9, 35345–35355. doi:10.1039/c9ra06437k
Zhang, M., Zhu, J., Zheng, Y., Guo, R., Wang, S., Mignani, S., et al. (2018). Doxorubicin-conjugated PAMAM dendrimers for pH-responsive drug release and folic acid-targeted cancer therapy. Pharmaceutics 10, 162. doi:10.3390/pharmaceutics10030162
Zhang, W., Wang, F., Wang, Y., Wang, J., Yu, Y., Guo, S., et al. (2016). pH and near-infrared light dual-stimuli responsive drug delivery using DNA-conjugated gold nanorods for effective treatment of multidrug resistant cancer cells. J. Control. Release 232, 9–19. doi:10.1016/j.jconrel.2016.04.001
Zhang, Y., Wang, H., Mukerabigwi, J. F., Liu, M., Luo, S., Lei, S., et al. (2015). Self-organized nanoparticle drug delivery systems from a folate-targeted dextran–doxorubicin conjugate loaded with doxorubicin against multidrug resistance. RSC Adv. 5, 71164–71173. doi:10.1039/c5ra10341j
Zhao, M. X. Z. (2016). The research and applications of quantum dots as nano-carriers for targeted drug delivery and cancer therapy. Nanoscale Res. Lett. 11, 207. doi:10.1186/s11671-016-1394-9
Zhao, N., Pei, S. N., Qi, J., Zeng, Z., Iyer, S. P., Lin, P., et al. (2015). Oligonucleotide aptamer-drug conjugates for targeted therapy of acute myeloid leukemia. Biomaterials 67, 42–51. doi:10.1016/j.biomaterials.2015.07.025
Zhou, Y., Chen, R., Yang, H., Bao, C., Fan, J., Wang, C., et al. (2020). Light-responsive polymersomes with a charge-switch for targeted drug delivery. J. Mat. Chem. B 8, 727–735. doi:10.1039/c9tb02411e
Zhu, L., Kate, P., and Torchilin, V. P. (2012). Matrix metalloprotease 2-responsive multifunctional liposomal nanocarrier for enhanced tumor targeting. ACS Nano 6, 3491–3498. doi:10.1021/nn300524f
Zhuang, C., Guan, X., Ma, H., Cong, H., Zhang, W., and Miao, Z. (2019). Small molecule-drug conjugates: A novel strategy for cancer-targeted treatment. Eur. J. Med. Chem. 163, 883–895. doi:10.1016/j.ejmech.2018.12.035
Keywords: smart drug delivery systems (SDDSs), cancer, translational medicine, nano-therapy, active targeting, passive targeting, targeted drug delivery, targeted cancer therapy
Citation: Rana A, Adhikary M, Singh PK, Das BC and Bhatnagar S (2023) “Smart” drug delivery: A window to future of translational medicine. Front. Chem. 10:1095598. doi: 10.3389/fchem.2022.1095598
Received: 11 November 2022; Accepted: 28 November 2022;
Published: 04 January 2023.
Edited by:
Bo Shen, Northwestern University, United StatesReviewed by:
Yuerou Zhang, Brown University, United StatesCopyright © 2023 Rana, Adhikary, Singh, Das and Bhatnagar. This is an open-access article distributed under the terms of the Creative Commons Attribution License (CC BY). The use, distribution or reproduction in other forums is permitted, provided the original author(s) and the copyright owner(s) are credited and that the original publication in this journal is cited, in accordance with accepted academic practice. No use, distribution or reproduction is permitted which does not comply with these terms.
*Correspondence: Seema Bhatnagar, c2JoYXRuYWdhcjFAYW1pdHkuZWR1
Disclaimer: All claims expressed in this article are solely those of the authors and do not necessarily represent those of their affiliated organizations, or those of the publisher, the editors and the reviewers. Any product that may be evaluated in this article or claim that may be made by its manufacturer is not guaranteed or endorsed by the publisher.
Research integrity at Frontiers
Learn more about the work of our research integrity team to safeguard the quality of each article we publish.