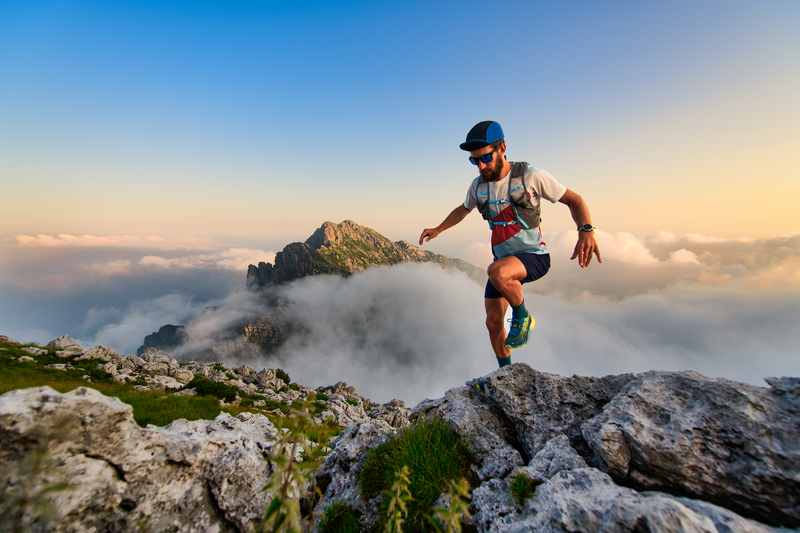
95% of researchers rate our articles as excellent or good
Learn more about the work of our research integrity team to safeguard the quality of each article we publish.
Find out more
MINI REVIEW article
Front. Chem. , 02 December 2022
Sec. Green and Sustainable Chemistry
Volume 10 - 2022 | https://doi.org/10.3389/fchem.2022.1084089
This article is part of the Research Topic Sustainable Catalytic Production of Bio-Based Heteroatom-Containing Compounds, Volume III View all 13 articles
Ionic liquids have attracted attention due to their excellent properties and potential for use as co-solvents, solvents, co-catalysts, catalysts, and as other chemical reagents. This mini-review focuses on the properties and structures of ionic liquids, the pretreatment of lignocellulosic biomass, and the development of novel ionic liquid-based solid catalysts for cellulose and hemicellulose derived HMF production.
As reported, lignocellulosic biomass is abundant (Zabed et al., 2017), has carbon–neutral properties, and is a sustainable and non-edible green feedstock (Hoang et al., 2021) that is a potential source material for the production of valuable biofuels and chemicals. The percentage of each constituent is determined by the wood/plant species, but, in general, is composed of cellulose (40%–50%), hemicellulose (25%–30%), and lignin (15%–20%), as well as small amounts of pectin, nitrogen compounds, and inorganic compounds (Kumar A. A. et al., 2020). The compound 5-hydroxymethylfurfural (HMF) is known as the “sleeping giant” of renewable intermediate chemicals, with derivatives that can be used in applications such as pesticides, medicines, and biofuel chemistry (Osatiashtiani et al., 2015; Le et al., 2022; Nasrollahzadeh et al., 2022). With current energy shortages and environmental pollution, it is critical that we seek green, sustainable, and alternative solutions.
Ethyl ammonium nitrate, which is a liquid at ambient temperature and pressure, was the first described ionic liquid (IL) in 1914 (Walden, 1914; Angell et al., 2012). ILs have been widely identified as green substitutes for organic solvents based on their near-zero vapor pressures, high thermal stability and devisable polarity, hydrophobicity, and excellent capacity as solvents through modification of cations and anions (Zhang et al., 2014; Amarasekara, 2016). To the best of our knowledge, whether ILs can be recognized as green depends on approaches to synthesis and internal physico-chemical properties.
An IL can also be regarded as a salt, depending on whether its melting point is below 100°C, as salts consist of large and asymmetrical ions that typically have lower melting points (Berthod et al., 2017). Generally, the melting temperature, Tm, decreases with increased size, anisotropy, and internal flexibility of the ions; however, Tm increases with enhanced alkyl chain interaction (Weingärtner, 2008). Viscosity is one of the most significant material properties of ILs. High values for viscosity limit applications of ILs in various areas, since it reduces rate of reaction and molecule diffusion by forming a circulation barrier (Weingärtner, 2008). Tests of the thermal stability of ILs have shown that decomposition happens slowly at nearly 200°C (Berthod et al., 2017) in a process that hinges on the unique cation and corresponding anion association. The thermal stability of the imidazolium salts, along with the growth of the number of alkyl substitutions, has been demonstrated (Ngo et al., 2000). ILs involving linear side chains are more thermally stable than comparable branched monocationic ones (Xue et al., 2016). Halide anions can also decrease thermal stability to a certain extent. Generally, cations account for viscosity, melting point, and electrochemical stability, whereas anions are responsible for hydrogen bonding and miscibility with other solvents or water (Puripat et al., 2016).
The first-generation ILs defined dialkylimidazolium and alkylpyridinium as cations and metal halide (FeCl4− and Al2Cl7−) as anions sensitive to water and air. Cations of quaternary ammonium and phosphonium containing dialkylimidazolium, alkylpyridinium, ammonium, and phosphonium, along with the classic anions tetrafluoroborate (BF4−) and hexafluorophosphate (PF6−) made up the second generation of ILs, which are not sensitive to either water or air. Unfortunately, slow hydrolysis of these anions with increased temperature leads to the production of hazardous and ecotoxic hydrogen fluoride (HF). From a green, sustainability perspective, second-generation ILs also show poor biodegradability, and are neither cost-effective nor green (Deetlefs and Seddon, 2010). Third-generation ILs are biodegradable cations and anions, and natural compounds containing choline, amino acids, or carbohydrates have been developed for IL production (Egorova et al., 2017). Widespread commercial application of ILs in various fields has been studied on account of these attributes (Figure 1).
Because of the above-mentioned characteristics, ILs have been identified as excellent solvents and catalysts for the synthesis of HMF from biomass and associated derivatives (Jiang et al., 2016; Li et al., 2018). Among IL cations, the mono-imidazole type has exhibited excellent performance. To facilitate the interaction between ILs and biomass or carbohydrates, di-/tri-cationic ILs with higher density and more hydrogen bonding were developed (Marullo et al., 2019; Rathod et al., 2019; Prasad et al., 2021).
Lignocellulosic biomass pretreatment is a significant process in the production of biofuels and value-added chemicals, the degradation of which is hindered by chemical properties, chemical structure, and microscopic complexity. Therefore, new approaches and reaction parameters are determined by attributes such as the degree of crystallization and polymerization of cellulose and the percentage of hemicellulose and lignin (Mood et al., 2013; Abraham et al., 2020) (Figure 2). Various ILs have been investigated to determine their effectiveness in lignocellulose dissolution and degradation to main compounds (Bian et al., 2014), although without the desired target HMF yield due to the multiple steps necessary to isolate HMF from raw materials.
Kahani et al. (2017) synthesized N-allyl-N-methylmorpholinium acetate ([AMMorph][Ac]) and successfully introduced it for the pretreatment of rice straw. Compared to the most efficient solvent imidazolium liquids, the morpholinium liquids are less toxic and less expensive, while providing glucose yields of 98.4 ± 1.3% using mixtures of [AMMorph][Ac]-DMSO (70:30, v/v) and N-methylmorpholine-N-oxide (NMMO), [Bmim][Ac], and NaOH at 120°C. Furthermore, using DMSO as a co-solvent could minimize IL usage and enhance pretreatment efficiency by reducing viscosity (Kahani et al., 2017).
Use of a novel integrated –SO3H functionalized IL catalyst [IL-SO3H][Cl] and nickel sulfate (NiSO4.6H2O) co-catalyst resulted in a maximum glucose conversion of 99.92%, with 21.80% HMF yield when incubated at 175°C for 1.5 h in the aqueous phase (Kumar K. et al., 2020). According to the pseudo-first-order kinetic equations, the activation energy (Ea) and pre-exponential factor (A) was confirmed to be 47.45 kJ/mol and 7.9 × 103 min−1, respectively, for conversion of glucose into HMF. The research demonstrated an effective synergistic effect of the IL catalyst and Lewis acidic co-catalyst in clean synthesis of HMF from waste biomass derived glucose, providing a promising pathway for the preparation of vital platform chemicals and renewable fuels.
The synthesis of HMF derived from cellulose involves several steps: depolymerization, hydrolysis, isomerization and, ultimately, dehydration (Tyufekchiev et al., 2018; Zhang et al., 2018a; Zhang et al., 2018b; Naz et al., 2021). For different processes, catalyst properties vary depending on the reactions.
Excessive Brønsted acid or Lewis acid can accelerate the side reactions that produce by-products. Therefore, the “tailor-made” development of satisfactory catalyst processing with the appropriate ratio of Lewis acid to Brønsted acid is crucial for increasing HMF yield. In addition, allylimidazole-type ILs have strong advantages in cellulose dissolution (Liu et al., 2012).
At the beginning of the instantiation phase, metallic ILs (i.e., Cr([PSMIM]HSO4)3 and CuCr([PSMIM]SO4)5) were designed and applied to the cellulose-HMF system (Zhou et al., 2013). Cr([PSMIM]HSO4)3 demonstrated higher catalytic performance in the production of HMF, with 53% yield ascribed to the bifunctionality and higher Brønsted acidity at 120°C.
Liu et al. (2022) prepared a series of reactions with different proportions of Brønsted acid and Lewis acid ILs for the degradation of cellulose to produce HMF. Among these, [(HSO3-P)2im]Cl⋅ZnCl2 exhibited excellent catalytic performance, with an HMF yield of 65.66% at 140°C for 3 h. This study facilitated directional optimization of the catalyst. The quantum chemical calculation method for molecular design was used to predict the catalytic effect (different ratios of Brønsted acid to Lewis acid) and investigate the catalytic mechanism. Therefore, the solvation model density (SMD) model was proposed in combination with Frontier orbital theory. In addition, cellulose degradation experiments were performed to verify the simulation results and inform discussion of the catalytic mechanism (Liu et al., 2022).
As the second most predominant component of lignocellulosic biomass, hemicellulose is composed of pentoses like xylose, arabinose, and hexose, including glucose, mannose, galactose, and the amorphous polymer xylan (Ruiz et al., 2013). There are few developments in the production of HMF derived from mannose and galactose. Researchers demonstrated that mannose is predominantly isomerized to fructose, which can be efficiently converted into HMF, while galactose primarily isomerizes to tagatose, which is the C-4 epimer of fructose and weaker than fructose in yielding HMF (van Putten et al., 2013).
When lignocellulosic biomass was employed as raw material, cellulose with higher HMF yield than hemicellulose was preferentially chosen for the synthesis of HMF, as the research was aimed at improving the conversion efficiency of cellulose (Menegazzo et al., 2018). Considering the complex components involved, the conversion conditions for HMF are difficult to control.
HMF yield close to 100% will eventually be achieved by adjusting the approach to isolation of the compound from feedstock and optimizing IL-based catalyst reaction conditions. This cost-effective, green, sustainable catalyst system, which inhibits by-products, is easily functionalized, and has no adverse environmental impact, will lead to significant advances in future industrial-scale HMF production. Density functional theory (DFT) and molecular dynamics should be applied in biomass conversion to aid in the development of reliable reaction pathways.
XL conceived the article and discussed the outline. XL wrote the manuscript. HL, DY, ZP, and ZZ made preliminary revisions to the manuscript. CL and XL coordinated the content of the manuscript and made detailed revisions.
This work was financially supported by the Technical Talent Support Program of Guizhou Education Department ([2022]087), the Guizhou Provincial Key Laboratory for Rare Animal and Economic Insects of the Mountainous Region ([2018]5102), the National Natural Science Foundation of China (22065004), and the Guizhou Science and Technology Foundation ([2020]1Y054).
The authors declare that the research was conducted in the absence of any commercial or financial relationships that could be construed as a potential conflict of interest.
The handling editor declared a past co-authorship with the authors XL and CL.
All claims expressed in this article are solely those of the authors and do not necessarily represent those of their affiliated organizations, or those of the publisher, the editors and the reviewers. Any product that may be evaluated in this article, or claim that may be made by its manufacturer, is not guaranteed or endorsed by the publisher.
Abraham, A., Mathew, A. K., Park, H., Choi, O., Sindhu, R., Parameswaran, B., et al. (2020). Pretreatment strategies for enhanced biogas production from lignocellulosic biomass. Bioresour. Technol. 301, 122725. doi:10.1016/j.biortech.2019.122725
Amarasekara, A. S. (2016). Acidic ionic liquids. Chem. Rev. 116, 6133–6183. doi:10.1021/acs.chemrev.5b00763
Angell, C. A., Ansari, Y., and Zhao, Z. (2012). Ionic liquids: Past, present and future. Faraday Discuss. 154, 9–27. doi:10.1039/C1FD00112D
Berthod, A., Ruiz-Ángel, M. J., and Carda-Broch, S. (2017). Recent advances on ionic liquid uses in separation techniques. J. Chromatogr. A 1559, 2–16. doi:10.1016/j.chroma.2017.09.044
Bian, J., Peng, F., Peng, X. P., Xiao, X., Peng, P., Xu, F., et al. (2014). Effect of [Emim]Ac pretreatment on the structure and enzymatic hydrolysis of sugarcane bagasse cellulose. Carbohydr. Polym. 100, 211–217. doi:10.1016/j.carbpol.2013.02.059
Deetlefs, M., and Seddon, K. R. (2010). Assessing the greenness of some typical laboratory ionic liquid preparations. Green Chem. 12, 17–30. doi:10.1039/B915049H
Egorova, K. S., Gordeev, E. G., and Ananikov, V. P. (2017). Biological activity of ionic liquids and their applicationin pharmaceutics and medicine. Chem. Rev. 117, 7132–7189. doi:10.1021/acs.chemrev.6b00562
Hoang, A. T., Ong, H. C., Fattah, I. M. R., Chong, C. T., Cheng, C. K., Sakthivel, R., et al. (2021). Progress on the lignocellulosic biomass pyrolysis for biofuel production toward environmental sustainability. Fuel Process. Technol. 223, 106997. doi:10.1016/j.fuproc.2021.106997
Jiang, Y., Zang, H., Han, S., Yan, B., Yu, S., and Cheng, B. (2016). Direct conversion of chitosan to 5-hydroxymethylfurfural in water using Brønsted-Lewis acidic ionic liquids as catalysts. RSC Adv. 6, 103774–103781. doi:10.1039/c6ra21289a
Kahani, S., Shafiei, M., Abdolmaleki, A., and Karimi, K. (2017). Enhancement of ethanol production by novel morpholinium ionic liquids. J. Clean. Prod. 168, 952–962. doi:10.1016/j.jclepro.2017.09.008
Kumar, A. A., Rapoport, A., Kunze, G., Kumar, S., Singh, D., Singh, B., et al. (2020). Multifarious pretreatment strategies for the lignocellulosic substrates for the generation of renewable and sustainable biofuels: A review. Renew. Energy 160, 1228–1252. doi:10.1016/j.renene.2020.07.031
Kumar, K., Pathak, S., and Upadhyayula, S. (2020). 2nd generation biomass derived glucose conversion to 5-hydroxymethylfurfural and levulinic acid catalyzed by ionic liquid and transition metal sulfate: Elucidation of kinetics and mechanism. J. Clean. Prod. 256, 120292. doi:10.1016/j.jclepro.2020.120292
Le, H. S., Said, Z., Pham, M. T., Le, T. H., Veza, I., Nguyen, V. N., et al. (2022). Production of HMF and DMF biofuel from carbohydrates through catalytic pathways as a sustainable strategy for the future energy sector. Fuel 324, 124474. doi:10.1016/j.fuel.2022.124474
Li, X. C., Zhang, Y. Y., Xia, Q. N., Liu, X. H., Peng, K. H., Yang, S. H., et al. (2018). Acid-free conversion of cellulose to 5-hydroxymethyl-furfural catalyzed by hot seawater. Ind. Eng. Chem. Res. 57, 3545–3553. doi:10.1021/acs.iecr.8b00443
Liu, D. T., Xia, K. F., Cai, W. H., Yang, R. D., Wang, L. Q., and Wang, B. (2012). Investigations about dissolution of cellulose in the 1-allyl-3-alkylimidazolium chloride ionic liquids. Carbohydr. Polym. 87 (2), 1058–1064. doi:10.1016/j.carbpol.2011.08.026
Liu, S. Y., Zheng, W. W., Wen, X. F., Fang, Z. Q., Li, H., Li, C. L., et al. (2022). Molecular design and experimental study of cellulose conversion to 5-hydroxymethylfurfural catalyzed by different ratios of Brønsted/Lewis acid ionic liquids. Carbohydr. Polym. 278, 118936. doi:10.1016/j.carbpol.2021.118936
Marullo, S., Rizzo, C., and Anna, F. D՛. (2019). Task-specific organic salts and ionic liquids binary mixtures: A combination to obtain 5-hydroxymethylfurfural from carbohydrates. Front. Chem. 7, 134–215. doi:10.3389/fchem.2019.00134
Menegazzo, F., Ghedini, E., and Signoretto, M. (2018). 5-Hydroxymethylfurfural (HMF) production from real biomasses. Molecules 23 (9), 2201. doi:10.3390/molecules23092201
Mood, S. H., Golfeshan, A. H., Tabatabaei, M., Jouzani, G. S., Najafi, G. H., Gholami, M., et al. (2013). Lignocellulosic biomass to bioethanol, a comprehensive review with a focus on pretreatment. Renew. Sustain. Energy Rev. 27, 77–93. doi:10.1016/j.rser.2013.06.033
Nasrollahzadeh, M., Nezafat, Z., Momenbeik, F., and Orooji, Y. (2022). Polystyrene immobilized Brønsted acid ionic liquid as an efficient and recyclable catalyst for the synthesis of 5-hydroxymethylfurfural from fructose. J. Mol. Liq. 345, 117811. doi:10.1016/j.molliq.2021.117811
Naz, S., Uroos, M., and Muhammad, N. (2021). Effect of molecular structure of cation and anions of ionic liquids and co-solvents on selectivity of 5-hydroxymethylfurfural from sugars, cellulose and real biomass. J. Mol. Liq. 334, 116523. doi:10.1016/j.molliq.2021.116523
Ngo, H. L., LeCompte, K., Hargens, L., and McEwen, A. B. (2000). Thermal properties of imidazolium ionic liquids. Thermochim. Acta 357, 97–102. doi:10.1016/S0040-6031(00)00373-7
Osatiashtiani, A., Lee, A. F., Granollers, M., Brown, D. R., Olivi, L., Morales, G., et al. (2015). Hydrothermally stable, conformal, sulfated zirconia monolayer catalysts for glucose conversion to 5-HMF. ACS Catal. 5, 4345–4352. doi:10.1021/acscatal.5b00965
Prasad, D., Patil, K. N., Manoorkar, V. K., Dateer, R. B., Nagaraja, B. M., and Jadhav, A. H. (2021). Sustainable catalytic process for fructose dehydration using dicationic ionic liquid assisted ZSM-5 zeolite. Mater. Manuf. Process. 36 (13), 1571–1578. doi:10.1080/10426914.2021.1905828
Puripat, A. M., Yokogawa, D., Parasuk, V., and Irle, S. (2016). Glucose transformation to 5-hydroxymethylfurfural in acidic ionic liquid: A quantum mechanical study. J. Comput. Chem. 37 (3), 327–335. doi:10.1002/jcc.24214
Rathod, P. V., Mujmule, R. B., Chung, W. J., Jadhav, A. R., and Kim, H. (2019). Efficient dehydration of glucose, sucrose, and fructose to 5-hydroxymethylfurfural using tri-cationic ionic liquids. Catal. Lett. 149 (3), 672–687. doi:10.1007/s10562-019-02667-0
Ruiz, H. A., Cerqueira, M. A., Silva, H. D., Rodríguez-Jasso, R. M., Vicente, A. A., and Teixeira, J. A. (2013). Biorefinery valorization of autohydrolysis wheat straw hemicellulose to be applied in a polymer-blend film. Carbohydr. Polym. 92, 2154–2162. doi:10.1016/j.carbpol.2012.11.054
Tyufekchiev, M., Duan, P., Schmidt-Rohr, K., Focil, S. G., Timko, M. T., and Emmert, M. H. (2018). Cellulase-inspired solid acids for cellulose hydrolysis: Structural explanations for high catalytic activity. ACS Catal. 8, 1464–1468. doi:10.1021/acscatal.7b04117
van Putten, R. J., Soetedjo, J. N. M., Pidko, E. A., van der Waalet, J. C., Hensen, E. J. M., Jong, E. D., et al. (2013). Dehydration of different ketoses and aldoses to 5-hydroxymethyl furfural. ChemSusChem 6 (9), 1681–1687. doi:10.1002/cssc.201300345
Walden, P. (1914). Ueber die Molekulargrösse und elektrische Leitfähigkeit einiger geschmolzenen Salze. Bull. Acad. Imp. Sci. Petersbg. 8, 405–422.
Weingärtner, H. (2008). Understanding ionic liquids at themolecular level: Facts, problems, and controversies. Angew. Chem. Int. Ed. 47, 654–670. doi:10.1002/anie.200604951
Xue, L. J., Gurung, E., Tamas, G., Koh, Y. P., Shadeck, M., Simon, S. L., et al. (2016). Effect of alkyl chain branching onphysicochemical properties of imidazolium-based ionic liquids. J. Chem. Eng. Data 61, 1078–1091. doi:10.1021/acs.jced.5b00658
Zabed, H., Sahu, J. N., Suely, A., Boyce, A. N., and Faruq, G. (2017). Bioethanol production from renewable sources: Current perspectives and technological progress. Renew. Sustain. Energy Rev. 71, 475–501. doi:10.1016/j.rser.2016.12.076
Zhang, S., Sun, J., Zhang, X., Xin, J., Miao, Q., and Wang, J. (2014). Ionic liquid-based green processes for energy production. Chem. Soc. Rev. 43, 7838–7869. doi:10.1039/C3CS60409H
Zhang, Y. L., Liu, M., Zhao, J. J., Wang, K., Meng, M. J., and Yan, Y. S. (2018a). Halloysite nanotubes templated acid-base bi-functional hollow polymeric solids for select conversion of cellulose to 5-hydroxymethylfurfural. ChemistrySelect 3, 5950–5959. doi:10.1002/slct.201800829
Zhang, Y. L., Xiong, Q., Chen, G. Y., Liu, M., Jin, P., Yan, Y. S., et al. (2018b). Synthesis of Ceria and sulfated zirconia catalysts supported on mesoporous SBA-15 toward glucose conversion to 5-hydroxymethylfurfural in a green isopropanol-mediated system. Ind. Eng. Chem. Res. 57, 1968–1979. doi:10.1021/acs.iecr.7b04671
Keywords: 5-hydroxymethylfurfural, ionic liquids, lignocellulosic biomass, cellulose, hemicellulose
Citation: Liu X, Luo H, Yu D, Pei Z, Zhang Z and Li C (2022) The development of novel ionic liquid-based solid catalysts and the conversion of 5-hydroxymethylfurfural from lignocellulosic biomass. Front. Chem. 10:1084089. doi: 10.3389/fchem.2022.1084089
Received: 30 October 2022; Accepted: 16 November 2022;
Published: 02 December 2022.
Edited by:
Hu Li, Guizhou University, ChinaReviewed by:
Qiuyun Zhang, Anshun University, ChinaCopyright © 2022 Liu, Luo, Yu, Pei, Zhang and Li. This is an open-access article distributed under the terms of the Creative Commons Attribution License (CC BY). The use, distribution or reproduction in other forums is permitted, provided the original author(s) and the copyright owner(s) are credited and that the original publication in this journal is cited, in accordance with accepted academic practice. No use, distribution or reproduction is permitted which does not comply with these terms.
*Correspondence: Can Li, bGljYW43OTAxMDhAMTYzLmNvbQ==
Disclaimer: All claims expressed in this article are solely those of the authors and do not necessarily represent those of their affiliated organizations, or those of the publisher, the editors and the reviewers. Any product that may be evaluated in this article or claim that may be made by its manufacturer is not guaranteed or endorsed by the publisher.
Research integrity at Frontiers
Learn more about the work of our research integrity team to safeguard the quality of each article we publish.