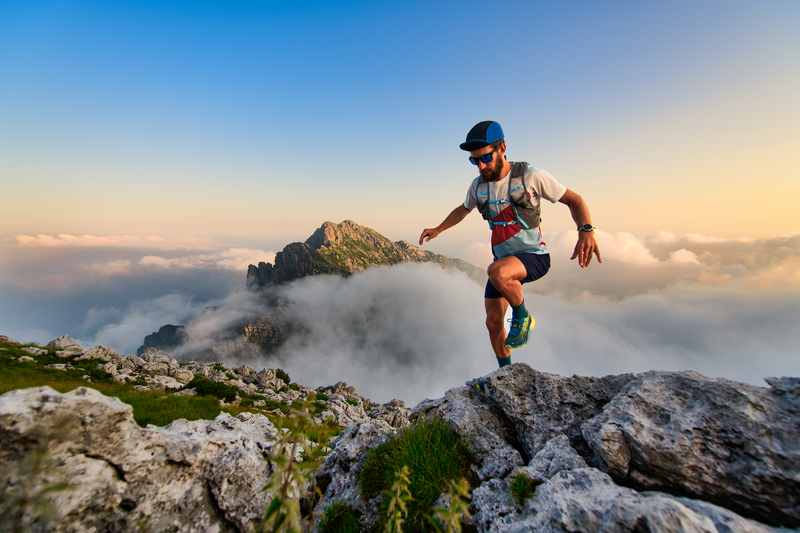
95% of researchers rate our articles as excellent or good
Learn more about the work of our research integrity team to safeguard the quality of each article we publish.
Find out more
ORIGINAL RESEARCH article
Front. Chem. , 09 January 2023
Sec. Theoretical and Computational Chemistry
Volume 10 - 2022 | https://doi.org/10.3389/fchem.2022.1061624
This article is part of the Research Topic Theoretical and Computational Chemistry Editor's Pick 2024 View all 17 articles
Eugenol is a natural compound with well-known repellent activity. However, its pharmaceutical and cosmetic applications are limited, since this compound is highly volatile and thermolabile. Nanoencapsulation provides protection, stability, conservation, and controlled release for several compounds. Here, eugenol was included in β-cyclodextrin, and the complex was characterized through X-ray diffraction analysis (XRD) and Fourier-transform infrared spectroscopy (FTIR). Additionally, we used molecular dynamics simulations to explore the eugenol–β-cyclodextrin complex stability with temperature increases. Our computational result demonstrates details of the molecular interactions and conformational changes of the eugenol–β-cyclodextrin complex and explains its stability between temperatures 27°C and 48°C, allowing its use in formulations that are subjected to varied temperatures.
Mosquitos are the main vectors of viral diseases that manifest predominantly in tropical and subtropical regions of the world, such as dengue, yellow fever, zika, and chikungunya (Paixão et al., 2018; Higuera and Ramírez, 2019; Barreto-Vieira et al., 2020). The chemical protection conferred by repellents against mosquitos has been an effective alternative to prevent their contact with the human skin (Ray, 2015; Mapossa et al., 2021). Natural products remain an interesting source of new bioactive compounds with different applications (Rayan et al., 2017; Galúcio et al., 2019; Do Nascimento et al., 2020; Newman and Cragg, 2020; Santana et al., 2021), and these structures have been widely investigated as a repellent against mosquitos (Tabanca et al., 2016; da Costa et al., 2019a). However, these compounds have been reported to have a short shelf life, in part, due to their volatile nature (Kayaci et al., 2013; Tan et al., 2019; Beltrán Sanahuja and Valdés García, 2021). Eugenol (4-allyl-2-methoxyphenol) is a volatile and lipophilic phenolic natural compound belonging to the class of phenylpropanoid, and it is mainly found in the essential oils of plants. Eugenol can be also produced synthetically by the allylation of guaiacol with allyl chloride (Moyer et al., 2002; Kuskoski et al., 2003). Eugenol is responsible for clove aroma, and it is well known for to its wide range of biological activities, such as antibacterial, antioxidant, anesthetic, and anti-inflammatory (Jaganathan et al., 2011; Kamatou et al., 2012; Roth-Walter et al., 2014; Xu et al., 2016; Mateen et al., 2019; Methods, 2021). The U S Food and Drug Administration also considers eugenol as a safe food additive for human use (Kamatou et al., 2012; El-Saber Batiha et al., 2020), and studies have demonstrated that eugenol has efficient repellent activity against different mosquitos species, such as Aedes aegypti (Miot et al., 2011; Afify and Potter, 2020), Culex quinquefasciatus (Afify et al., 2019), and Anopheles gambiae (Lupi et al., 2013; Thomas et al., 2017).
Several experimental studies have reported the formation of inclusion complexes of eugenol with encapsulating agents, such as β-cyclodextrin, to reduce the undesirable effects (localized irritation of the skin and allergic contact dermatitis), increase its aqueous solubility, and prolong its biological activity (Yang and Song, 2005; Abarca et al., 2016; Gong et al., 2016; Kfoury et al., 2018; de Freitas et al., 2021). β-cyclodextrin (cyclohepta-amylose) is a cyclic oligosaccharide formed by D-glucose monomers which are produced by the enzymatic degradation of starch (Szejtli, 1998; Wüpper et al., 2021), and it is particularly interesting for the encapsulation of volatile compounds, thus representing a viable and efficient strategy to retain and modulate the release of volatile and hydrophobic compounds (Abarca et al., 2016; Kfoury et al., 2018; Zheng et al., 2020). Cyclodextrin inclusion complexation is widely used in food, cosmetics, agrochemical, and pharmaceutical industries to increase the stability of several volatile organic compounds due to its hydrophobic cavities and hydrophilic exterior (Loftsson and Brewster, 1996; Szejtli, 1998; Muthu Vijayan Enoch and Swaminathan, 2004; Enoch and Swaminathan, 2005; Xu et al., 2021), which creates a physical barrier between the nucleus and the shell materials (Anaya-Castro et al., 2017).
The α-, β-, and γ-cyclodextrins are subclasses of cyclodextrins widely used for nanoencapsulation, and they could be differentiated by the presence of 6, 7, and 8 glucopyranose units, respectively, that determine the size of their internal cavity (Szejtli, 1998; Saha et al., 2016; Jansook et al., 2018). These cyclodextrins have a truncated cone-shaped molecular form, and their hydrophobic cavities have a remarkable ability to form non-covalent inclusion complexes with a variety of compounds (Lee et al., 2020; Pena et al., 2022). During the formation of an inclusion complex, water molecules are displaced to the outside of the lipophilic cavity, due to the presence of new lipophilic guest molecules that induce a new equilibrium (Anaya-Castro et al., 2017). This water displacement and the formation of a stable complex depends on the binding forces present in the inclusion complex (e.g., hydrophobic interactions, van der Waals attractions, hydrogen bonds, and electrostatic interactions) and temperature (Alvira, 2018; Lee et al., 2020).
Temperature is an important variable to assess the stability of the inclusion complexes, and understanding its influences on the formation of intermolecular interactions and mass loss is crucial to the experimental tests that evaluate the repellent efficiency (Kayaci and Uyar, 2011; Abarca et al., 2016; Celebioglu et al., 2018). Several studies have performed computational analyses to investigate the formation of inclusion complexes formed between the oligosaccharides and the natural products (de Sousa et al., 2016; Mustafa et al., 2021; Rezaeisadat et al., 2021). Similarly, experimental studies have investigated the formation of these complexes between eugenol and its derivates with β-cyclodextrin (Alvira, 2018; Joardar et al., 2020) and have identified a slower controlled release of eugenol at elevated temperatures, such as 50°C, 75°C, and 100°C (Kayaci et al., 2013; Celebioglu et al., 2018).
In the present study, we analyzed the eugenol–β-cyclodextrin inclusion complex through X-ray diffraction analysis (XRD) and Fourier-transform infrared spectroscopy (FTIR) and its chemical stability and binding affinity using molecular dynamics (MD) simulations and binding free energy calculations, respectively. The representative structures of the analyzed systems are shown in Supplementary Figure S1.
The eugenol (CAS: 97-53-0, medium molecular weight: 164.2 g/ml, and purity: 99%) and the commercial β-cyclodextrin (CAS: 7585-39-9, medium molecular weight: 1,134.98 g/ml, and purity: 97%) were obtained from Sigma Aldrich Laboratory (São Paulo, Brazil).
The inclusion complex was formed through co-precipitation and solvent evaporation (Ayala-Zavala et al., 2008), in which the hydroalcoholic solution of β-cyclodextrin was incorporated into an alcoholic solution of eugenol to obtain molar ratios 1:1, 2:1, and 2:3 in duplicate, to compare and analyze the influence of concentration molars in the final product of the complexes. The physical mixture was obtained by maceration, in grade and pistil, until the formation of a paste and homogenized for 15 min; then, it was allowed to rest for 24 h in an isolated environment, dried at 50°C for 12 h, and stored as other inclusion complexes.
The measurements were recorded in a divergent beam diffractometer (model: Empyrean from PANalytical) with a θ–θ goniometer, ceramic X-ray tube sealed with a cobalt anode, monochromatic radiation of Co-Kα1 (λ = 1.789 ″A"), long fine focus 1800W, and a Fe kβ filter. The detector PIXel3D 2 × 2 area was used with an active length of 3.3473° (2θ–2Theta) and 255 active channels. The following instrumental conditions were applied in the analyses: voltage of 40 kV and current of 40 mA, solar encapsulation slits of 0.04°rad (in the incident and diffracted beams), 2°–80° (2θ) sweep range, and 0.04° step size in 2θ with 1s time/step in continuous scan mode. Phase identification was performed using PANalytical’s HighScore Plus 4.8.0 software.
Powder X-ray diffractometry is a useful method to confirm the formation of complex powder or microcrystalline states; therefore, the XRD technique is only applied to materials (solid-state matter). In the present study, it was applied to analyze the materials: free β-cyclodextrin (β-CD), eugenol–β-cyclodextrin (EG-β-CD) complexes, and physical mixture (PM).
The spectroscopic investigations were performed to identify the functional groups of the eugenol–β-cyclodextrin complex in the middle infrared spectral region—Middle-IR (4,000–400 cm−1)—using a Thermo Scientific Fourier transform infrared spectrometer (model: Nicolet iS50 FTIR), a KBr (potassium bromide) beam splitter, an IR source, and a KBr DTGS detector. The measurements were obtained by transmission with KBr pellets (0.15 g) + sample (0.002 g), with an average of 100 scans and a resolution of 8 cm−1. Data were acquired using OMNIC software. As a pre-treatment, the samples were dried at 105°C for 24 h.
To investigate the most stable conformation of eugenol in complex with β-cyclodextrin, we performed molecular docking using AutoDock Vina (Trott and Olson, 2009). This computational method allowed us to describe the molecular interactions of the eugenol with the internal cavity (lipophilic) and external surface (hydrophilic) of the β-cyclodextrin nanoparticle. Herein, we used the crystallographic structure of β-cyclodextrin (PDB code: 3EDJ) as the starting point to perform the simulations (Buedenbender and Schulz, 2009). We used the following spatial coordinates for the docking grid: X = 69.62, Y = 67.16, and Z = 40.77, with dimensions of X = 40, Y = 40, and Z = 40 Å. The docking simulations were performed with 10 runs, and a total of 10 conformations per compound were set to perform the docking. The formation of the intermolecular interactions, such as H-bond, π-interactions, and hydrophobic interactions, were analyzed using BIOVIA Discovery Studio (BIOVIA, 2017).
To perform the MD simulations, we selected the lowest energy structure of the eugenol/β-cyclodextrin complex obtained from the docking simulations. First, the atomic charges of eugenol were calculated using the restrained electrostatic potential atomic partial charges (RESP) protocol (Wang et al., 2000; Wang et al., 2004) using the Hartree–Fock method with the 6-31G* basis set (Bayly et al., 1993) available in the Gaussian09 program (Frisch et al., 2009). The carbohydrate force field Glycam06 (Kirschner et al., 2008) was used to treat β-cyclodextrin, and the general AMBER force field (GAFF) was used to treat the complex formed with the eugenol (Wang et al., 2004). The complex was solvated in a cubic water box using the TIP3P model (Jorgensen et al., 1983; Jorgensen et al., 1996), and the distance between the box wall and atoms of the system was set to 12.0 Å.
The geometry and the inter- and intra-atomic distances of hydrogen molecules, water molecules, and the eugenol–β-cyclodextrin complex were optimized in seven minimization steps using 100,000 cycles of steepest descent and the conjugate gradient method (Hestenes and Stiefel, 1952). The β-cyclodextrin–eugenol complex was investigated in four different temperatures: 27°C, 38°C, 48°C, and 58°C. In the MD simulations, the systems were heated to their final temperature (300 K) to equilibrate the density and maintain the constant pressure (1 atm). The SHAKE algorithm (AndersenRattle, 1983) was applied for all hydrogen molecules of the analyzed systems. A total time of 50 ns of MD simulation was performed using NPT ensemble.
The diffraction pattern of the β-cyclodextrin–eugenol complexes in different stoichiometric proportions showed a similar trend, demonstrating only some different intensities between the 2:3 ratio and the others, in which the peaks are presented in greater intensity, in 3600 counts (Figure 1). However, all analyzed complexes show considerable differences when compared to the diffraction pattern of free β-cyclodextrin and the physical mixture (Figure 1). The free β-cyclodextrin diffractogram presents many Bragg reflections, highlighting the high-intensity peaks in °2θ (CoKα): 5.3°, 10.5°, 12.4°, 14.8°, 18.2°, 22.1°, and 26.8°, as observed in studies carried out with β-cyclodextrin and other guests (Wang et al., 2011; Gong et al., 2016; Yang et al., 2016; Jiang et al., 2019), having as characteristic peak the angle °2θ at 5.3° provided by the database of software used.
FIGURE 1. Diffraction patterns of free β-cyclodextrin (β-CD), inclusion complex of eugenol–β-cyclodextrin in a 1:1 ratio, inclusion complex of eugenol–β-cyclodextrin in a 2:1 ratio, inclusion complex of eugenol–β-cyclodextrin in a 2:3 ratio, and physical mixture (PM) were analyzed using the diffractograms.
We found a significant difference between the diffractograms of free β-cyclodextrin and the β-cyclodextrin and eugenol complexes, indicating the occurrence of encapsulation and the interaction between eugenol and β-cyclodextrin, given a reordering in the crystal structure, by the disappearance of peaks in 10.5° and 12.4 °2θ (CoKα) and contraction of the unit cell with decreasing dhkl, that is, by increasing the angle, as observed for 6.8, 13.7, 21.2° and 24.4 °2θ (CoKα), data that corroborate the results previously found (Yang and Song, 2005; Abarca et al., 2016; Dos Passos Menezes et al., 2017), which may be associated with changes in the molecular organization of β-cyclodextrin during the production of complexes.
The peak shifts indicate that the diffraction pattern of free β-cyclodextrin was altered when the eugenol was incorporated into the host molecule cavity. In studies that present the physical mixture diffractogram between eugenol and β-cyclodextrin, crystalline peaks of β-cyclodextrin were detected at a lower intensity, indicating that there was no marked difference in the crystalline form of β-cyclodextrin (Abarca et al., 2016; Gong et al., 2016), as seen in Figure 2. Furthermore, it is also important to note that the peak intensities in the eugenol–β-cyclodextrin complex were attenuated in relation to the same peaks in the free β-cyclodextrin spectrum, indicating greater structural disorder or loss in the degree of crystallinity for the complex (Figure 2). This fact is attributed to the rapid precipitation of the complex during preparation, which makes regular crystal growth insufficient (Yang and Song, 2005).
FIGURE 2. Diffraction patterns of free β-cyclodextrin (β-CD), eugenol–β-cyclodextrin (EG-β-CD) complex, and physical mixture (PM) were analyzed using the diffractograms.
In this analysis, the diffractograms of the pure species were compared with the values obtained of the complex (Cao et al., 2005). The differences obtained from the analyses, such as the appearance or disappearance of peaks or changes in relative intensities, evidenced the formation of the inclusion complex since the principle of the complexation is associated with an increase in the degree of amorphization of the substances involved in the formation of the complex in the solid-state (Ribeiro et al., 2008; Gao et al., 2020).
The FTIR technique is a very helpful tool to prove the interaction of both guest and host molecules in their inclusion complexes in a solid phase (Singh et al., 2010). Figure 3 shows the FTIR spectra for 1) β-cyclodextrin, 2) physical mixture of eugenol and β-cyclodextrin, 3) inclusion complex of eugenol–β-cyclodextrin in a 1:1 ratio, 4) inclusion complex of eugenol–β-cyclodextrin in a 2:1 ratio, and 5) inclusion complex of eugenol–β-cyclodextrin in a 2:3 ratio. The spectra bands which characterize the absorption regions of β-cyclodextrin are associated with the stretches, referring to the symmetric and asymmetric deformation of the hydroxyl group (OH) in the range of 3,600–3,000 cm−1, that showed the characteristic bands of primary and secondary OH groups in 3384.57 cm−1.
FIGURE 3. Comparison between the FTIR spectra for the β-cyclodextrin, physical mixture of eugenol and β-cyclodextrin, inclusion complex of eugenol–β-cyclodextrin in a 1:1 ratio, inclusion complex of eugenol–β-cyclodextrin in a 2:1 ratio, and inclusion complex of eugenol–β-cyclodextrin in a 2:3 ratio.
We also observed the CH bands of the β-cyclodextrin ring and methyl groups in the range of 2,940–2,840 cm−1. These results were also described in previous studies (Celebioglu et al., 2018; Hadian et al., 2018), and the bands between 1,700 cm−1 and 1,600 cm−1 are associated with HOH bonds which are also abundant in the compound (Abarca et al., 2016). IR spectra are particularly sensitive to the presence of water; therefore, the spectral region of interest presents this contribution assigned to the HOH bending mode (Venuti et al., 2015). In addition, the spectra display the OH bending vibration in the range of 1,030–1,015 cm−1 and C-O-C stretch, between 1,159 and 1,143 cm−1. These elongation vibrations were described previously in the literature (Celebioglu et al., 2018; Gao et al., 2020). A broad hydroxyl band of pure β-cyclodextrin spectrum (Figures 3, 4) shows the maximum absorption at 3,384.57 cm−1, found in the FTIR spectrum of the inclusion complexes which is a good indication of their formation due to the stretching vibrations of the different β-cyclodextrin OH groups (Yang and Song, 2005; Kayaci et al., 2013; Celebioglu et al., 2018; Briñez-Ortega et al., 2020).
Table 1 shows some increase and decrease in intensity changes due to the insertion of the part ring into the electron-rich cavity of β-cyclodextrin. Some bands showed little or no changes in the band upon complexation, implying that the inclusion of guest molecules in the CD cavity does not affect this vibrational mode. Although the spectrum of the inclusion complex appears almost similar to that of β-cyclodextrin alone, these results indicate the formation of the inclusion complex due to the weak interactions when partial inclusion of the ligand occurs (Stepniak et al., 2015; Vestland et al., 2015).
TABLE 1. Comparison between the intensity of free β-cyclodextrin, inclusion complexes, and physical mixture.
In relation to the presence of eugenol in the composition of the complex, it can be proven by the presence of absorption regions at 1515.50 cm−1 (Figure 3), related to the C=C bonds of the aromatic ring of the compound that usually appears in the region between 1650 and 1250 cm−1 corresponding to the vibration of the C=C groups and CH flexion of the alkene/aromatic groups of the eugenol (Yang and Song, 2005; Kayaci et al., 2013; Celebioglu et al., 2018). Additionally, frequencies between 1,200 cm−1 and 1,000 cm−1 are related to the presence of CO stretches (Yang and Song, 2005; Scremin et al., 2018). In addition, for eugenol, the characteristic bands are found in the ranges of 3,380–3,360 cm−1 due to the axial stretching of the OH group (Rodríguez et al., 2021). Therefore, the results presented previously indicate the efficiency of the encapsulation process of the eugenol molecules by the β-cyclodextrin (Wang et al., 2011).
Generally, the comparative analysis of the physical mixture with the inclusion complex (Figure 4) presents the simple sum of the β-cyclodextrin and ligand bands (Zheng et al., 2020). A similar result was observed in the spectra. In the region of the absorption bands of the C–H stretches, we noted an overlap of the complex bands with that of β-cyclodextrin, without changes in the wavenumbers of the pure components. Similar results were noted in the region between 1,700 cm−1 and 1650 cm−1, where stretch bands C=C with subtle deformation were observed. These results suggest that the simple mixture of the two components in the solid phase is not enough to prove the formation of the inclusion complex, once the shape, intensity, and position of the peaks vary. These observations, combined with the results obtained by XRD, can be considered strong evidence of the formation of the inclusion complex.
Molecular modeling analyses have been widely applied to assess the conformational, magnetic, and electronic properties of molecules (de Castro et al., 2014; de Sousa et al., 2016; de Souza Farias et al., 2021; Mustafa et al., 2021). Here, these computational analyses of the host–guest interactions were performed to better understand the formation of the eugenol–β-cyclodextrin complex and to provide additional insights into the complex model, especially when it is subjected to different temperatures in an aqueous solution.
The molecular analyses of the inclusion complex using molecular docking demonstrated that eugenol predominately had van der Waals interactions with the interior cavity of the β-cyclodextrin. Previous studies demonstrated that the geometric optimization of eugenol using density functional theory led eugenol to project its structure onto the external surface of the host (Mahboub, 2014; Chowdhry et al., 2015). Figure 5 shows the final conformation acquired by eugenol when complexed with β-cyclodextrin, obtained from molecular docking. The complex showed binding energy equal to −4.0 kcal mol−1, and two hydrogen bond interactions were formed in the complex which contributed to the stability of eugenol in the inclusion complex. These molecular findings agree with the results reported previously (Joardar et al., 2020).
FIGURE 5. Inclusion complex formed between the eugenol (green) and the β-cyclodextrin (cyan). (A) 3D structure of BCD with eugenol forming the complex. (B) Exerting hydrogen bonds with the main electronegative group structure of EG. (C) EG (in green) inside the BCD cavity. (D) Eugenol with VDW surface, demonstrating binding in the BCD cavity.
Experimental thermal analyses, in general, reveal marked structural differences between isolated molecules and the inclusion complexes. In addition, these analyses exhibit a typical sharp melting endotherm at temperatures over 250° C, which is indicative of the anhydrous and crystalline state of the analyzed molecules; it also exhibits effects regarding their dehydration and degradation process (Seo et al., 2010; Piletti et al., 2017; Rodríguez et al., 2021). Since previous studies have identified a slower controlled release of eugenol at elevated temperatures, such as 50°C, 75°C, and 100°C (Kayaci et al., 2013; Piletti et al., 2017; Celebioglu et al., 2018), we analyzed the eugenol–β-cyclodextrin complex using a gradual temperature increase to evaluate the behavior and stability of the complex in aqueous solution, which is also compatible with its applications in repellent formulations (Mapossa et al., 2021). Computationally, we demonstrated that when the temperature is increased, it directly affects the interaction and stability of the complex (see RMSD plot, Figure 6A).
FIGURE 6. RMSD plots of the eugenol–β-cyclodextrin complex were evaluated at different temperatures. Complex analyzed over 50 ns of MD simulation at 27°C, 38°C, 48°C, and 58°C.
Between temperatures 27°C and 48°C, the complex showed stability, and eugenol maintained its interactions. However, we noted that for the temperature of 58°C, the RMSD plot showed high fluctuations (Figure 6), and eugenol was shown to be unstable in the complex, thus losing some of its interactions with the host and leaving the β-cyclodextrin cavity. Low stability of the inclusion complex can be attributed to poor interaction and orientation of eugenol inside the β-cyclodextrin cavity. These results demonstrate that the high temperatures impair the formation of the eugenol–β-cyclodextrin complex, reducing its interactions and leading to the departure of the eugenol from the complex. We conjecture that the complex will remain stable in solution when subjected to moderate temperatures due to the protection and stability provided by the β-cyclodextrin.
Since the binding affinity depends on the molecular interactions formed between eugenol and the β-cyclodextrin surface and directly affects the temperature increase (Supplementary Figures S2, S3, S4), we investigated the β-cyclodextrin–eugenol complex using different temperatures. The electrostatic (Eelec) and van der Waals (Evdw) energies of the analyzed systems (Figure 7) were calculated using the LIE implemented in the Cpptraj program (https://amberhub.chpc.utah.edu/lie/) (Åqvist et al., 2002; Brandsdal et al., 2003; Roe and Cheatham, 2013). At 20 ns and 30 ns of MD trajectory, the complex showed an Eelec value of approximately −100 kcal mol−1; however, with temperature increase, the energies tend to move toward values near zero. In contrast, the Evdw increases with increasing temperature , thus indicating the presence of repulsion forces caused by the instability of the complex. Recently, a study demonstrated that the binding affinity in host–guest systems including β-cyclodextrin may be estimated with a root mean square error <1.5 kcal mol−1 from the experimental results using the LIE method (Montalvo-Acosta et al., 2018), which is closely related to the error of <1 kcal mol−1 found in the experimental values obtained in the prediction of the relative binding affinity for a vast range of protein–ligand systems (Gapsys et al., 2020).
FIGURE 7. RMSD plots of the eugenol–β-cyclodextrin complex were obtained from different temperatures. (A) electrostatic energy (Eelec) and (B) van der Waals energy (Evdw) complex analyzed over 50 ns of MD simulation at 27°C, 38°C, 48°C, and 58°C.
Additionally, the binding free energy was calculated using the MM/GBSA method for different temperatures, considering the total complexation time (Figure 8). The LIE and MM/GBSA are both end-point methods widely applied to investigate the binding free energy of biomolecular complexes (Cardoso et al., 2021; Costa et al., 2021; da Costa et al., 2019b; de Oliveira et al., 2020; Fonseca et al., 2020), and their results tend to show a trend similar to the energy values obtained from the experimental methods (Hansson et al., 1998; Aqvist and Marelius, 2001; Zhu et al., 2017; Rifai et al., 2020). The energy at room temperature (27°C) showed a highly stable complex, with eugenol demonstrating a high binding affinity with the β-cyclodextrin. However, with the increase of the temperature, the complex stability is affected and we have a decrease in the free energy of the analyzed systems, thus reducing the affinity of eugenol and causing its departure from the complex. This result indicates that the eugenol–β-cyclodextrin complex is stable at moderate temperatures and guarantees the permanence of the molecule inside the β-cyclodextrin with stronger binding.
FIGURE 8. Binding free energies (kcal mol−1) were calculated using the MM/GBSA method for the eugenol–β-cyclodextrin complex. The inclusion complex was analyzed at different temperatures (27°C, 38°C, 48°C, and 58°C).
Here, we obtained eugenol–β-cyclodextrin inclusion complexes through co-precipitation and solvent evaporation. Then, the inclusion complex was characterized using the X-Ray diffraction and Fourier transform infrared spectroscopy, confirming the formation of the host–guest inclusion complex. Additionally, our computational analyses demonstrated that the eugenol–β-cyclodextrin complex remains stable between temperatures 27°C and 48°C. In contrast, high temperatures impair the formation of the eugenol–β-cyclodextrin complex, reducing its interactions and leading to its premature departure from the complex which is consistent with controlled release of the repellent.
The original contributions presented in the study are included in the article/Supplementary Material; further inquiries can be directed to the corresponding author.
All authors listed have made a substantial, direct, and intellectual contribution to the work and approved it for publication.
The authors are grateful to Conselho Nacional de Desenvolvimento Científico e Tecnológico (CNPq) and Coordenação de Aperfeiçoamento de Pessoal de Nível Superior (CAPES) for their financial support. The authors are also thankful for access to the computational resources of the Supercomputer Santos Dumont (SDumont), provided by the Laboratório de Computação Científica (LNCC). We also would like to thank to the financial support of the Pro-reitoria de Pesquisa da UFPA (PROPESP/UFPA).
The authors declare that the research was conducted in the absence of any commercial or financial relationships that could be construed as a potential conflict of interest.
All claims expressed in this article are solely those of the authors and do not necessarily represent those of their affiliated organizations, or those of the publisher, the editors, and the reviewers. Any product that may be evaluated in this article, or claim that may be made by its manufacturer, is not guaranteed or endorsed by the publisher.
The Supplementary Material for this article can be found online at: https://www.frontiersin.org/articles/10.3389/fchem.2022.1061624/full#supplementary-material
Abarca, R. L., Rodríguez, F. J., Guarda, A., Galotto, M. J., and Bruna, J. E. (2016). Characterization of beta-cyclodextrin inclusion complexes containing an essential oil component. Food Chem. 196, 968–975. doi:10.1016/j.foodchem.2015.10.023
Afify, A., Betz, J. F., Riabinina, O., Lahondère, C., and Potter, C. J. (2019). Commonly used insect repellents hide human odors from Anopheles mosquitoes. Curr. Biol. 29 (21), 3669–3680. e5. doi:10.1016/j.cub.2019.09.007
Afify, A., and Potter, C. J. (2020). Insect repellents mediate species-specific olfactory behaviours in mosquitoes. Malar. J. 19 (1), 127–210. doi:10.1186/s12936-020-03206-8
Alvira, E. (2018). Theoretical study of the β-cyclodextrin inclusion complex formation of eugenol in water. Molecules 23 (4), 928. doi:10.3390/molecules23040928
Anaya-Castro, M. A., Ayala-Zavala, J. F., Muñoz-Castellanos, L., Hernández-Ochoa, L., Peydecastaing, J., and Durrieu, V. (2017). β-Cyclodextrin inclusion complexes containing clove (eugenia caryophyllata) and Mexican oregano (lippia berlandieri) essential oils: Preparation, physicochemical and antimicrobial characterization. Food Packag Shelf Life 14, 96–101. doi:10.1016/j.fpsl.2017.09.002
AndersenRattle, H. C. (1983). Rattle: A “velocity” version of the shake algorithm for molecular dynamics calculations. J. Comput. Phys. 52 (1), 24–34. doi:10.1016/0021-9991(83)90014-1
Åqvist, J., Luzhkov, V. B., Brandsdal, B. O., Aqvist, J., Luzhkov, V. B., and Brandsdal, B. O. (2002). Ligand binding affinities from MD simulations. Acc. Chem. Res. 35 (6), 358–365. doi:10.1021/ar010014p
Aqvist, J., and Marelius, J. (2001). The linear interaction energy method for predicting ligand binding free energies. Comb. Chem. High. Throughput Screen 4 (8), 613–626. doi:10.2174/1386207013330661
Ayala-Zavala, J. F., Soto-Valdez, H., González-León, A., Álvarez-Parrilla, E., Martín-Belloso, O., and González-Aguilar, G. A. (2008). Microencapsulation of cinnamon leaf (cinnamomum zeylanicum) and garlic (allium sativum) oils in β-cyclodextrin. J. Incl. Phenom. Macrocycl. Chem. 60 (3–4), 359–368. doi:10.1007/s10847-007-9385-1
Barreto-Vieira, D. F., Couto-Lima, D., Jácome, F. C., Caldas, G. C., and Barth, O. M. (2020). Dengue, yellow fever, zika and chikungunya epidemic arboviruses in Brazil: Ultrastructural aspects. Mem. Inst. Oswaldo Cruz 115, e200278. doi:10.1590/0074-02760200278
Bayly, C. I., Cieplak, P., Cornell, W., and Kollman, P. A. (1993). A well-behaved electrostatic potential based method using charge restraints for deriving atomic charges: The RESP model. J. Phys. Chem. 97 (40), 10269–10280. doi:10.1021/j100142a004
Beltrán Sanahuja, A., and Valdés García, A. (2021). New trends in the use of volatile compounds in food packaging. Polym. (Basel) 13 (7), 1053. doi:10.3390/polym13071053
Brandsdal, B. O., Österberg, F., Almlöf, M., Feierberg, I., Luzhkov, V. B., and Åqvist, J. (2003). Free energy calculations and ligand binding. Adv. Protein Chem. 66, 123–158. doi:10.1016/S0065-3233(03)66004-3
Briñez-Ortega, E., De Almeida, V. L., Lopes, J. C. D., and Burgos, A. E. (2020). Partial inclusion of bis(1, 10-phenanthroline) silver(i) salicylate in β-cyclodextrin: Spectroscopic characterization, in vitro and in silico antimicrobial evaluation.Acad Bras Cienc 92 (3), e20181323–23. doi:10.1590/0001-3765202020181323
Buedenbender, S., and Schulz, G. E. (2009). Structural base for enzymatic cyclodextrin hydrolysis. J. Mol. Biol. 385 (2), 606–617. doi:10.1016/j.jmb.2008.10.085
Cao, F., Guo, J., and Ping, Q. (2005). The physicochemical characteristics of freeze-dried scutellarin-cyclodextrin tetracomponent complexes. Drug Dev. Ind. Pharm. 31 (8), 747–756. doi:10.1080/03639040500216220
Cardoso, R., Valente, R., Souza da Costa, C. H., da, S., Gonçalves Vianez, J. L., Santana da Costa, K., et al. (2021). Analysis of kojic acid derivatives as competitive inhibitors of tyrosinase: A molecular modeling approach. Molecules 26 (10), 2875. doi:10.3390/molecules26102875
Celebioglu, A., Yildiz, Z. I., and Uyar, T. (2018). Fabrication of electrospun eugenol/cyclodextrin inclusion complex nanofibrous webs for enhanced antioxidant property, water solubility, and high temperature stability. J. Agric. Food Chem. 66 (2), 457–466. doi:10.1021/acs.jafc.7b04312
Chowdhry, B. Z., Ryall, J. P., Dines, T. J., and Mendham, A. P. (2015). Infrared and Raman spectroscopy of eugenol, isoeugenol, and methyl eugenol: Conformational analysis and vibrational assignments from density functional theory calculations of the anharmonic fundamentals. J. Phys. Chem. A 119 (46), 11280–11292. doi:10.1021/acs.jpca.5b07607
Costa, C. H. S., Santos, A. M., Alves, C. N., Martí, S., Moliner, V., Santana, K., et al. (2021). Assessment of the PETase conformational changes induced by poly(ethylene terephthalate) binding. Proteins Struct. Funct. Bioinforma. 89 (10), 1340–1352. doi:10.1002/prot.26155
da Costa, K. S., Galúcio, J. M., Da Costa, C. H. S., Santana, A. R., Dos Santos Carvalho, V., Do Nascimento, L. D., et al. (2019a). Exploring the potentiality of natural products from essential oils as inhibitors of odorant-binding proteins: A structure- and ligand-based virtual screening approach to find novel mosquito repellents. ACS Omega 4 (27), 22475–22486. doi:10.1021/acsomega.9b03157
da Costa, K. S., Galúcio, J. M., de Jesus, D. A., Gomes, G. C., Lima e Lima, A. H., Taube, P. S., et al. (2019b). Targeting peptidyl-prolyl cis-trans isomerase NIMA-interacting 1: A structure-based virtual screening approach to find novel inhibitors. Curr. Comput. Aided Drug Des. 15, 605–617. doi:10.2174/1573409915666191025114009
de Castro, E. A. S., de Oliveira, D. A. B., Farias, S. A. S., Gargano, R., and Martins, J. B. L. (2014). Structure and electronic properties of azadirachtin. J. Mol. Model 20 (2), 2084. doi:10.1007/s00894-014-2084-0
de Freitas, C. A. B., de Araújo, R. C. S., da Paz, S. P. A., de Araújo Silva, J. R., Alves, C. N., and Lameira, J. (2021). Obtenção E caracterização de complexo de inclusão de β-ciclodextrina E eugenol/ preparation and characterization of β-cyclodextrin inclusion complex of eugenol. Braz. J. Dev. 7 (3), 33056–33070. Obtenção E Caracterização De Complexo De Inclusão De Β-Ciclodextrina E. doi:10.34117/bjdv7n3-838
de Oliveira, M. D., Araújo, J. de O., Galúcio, J. M. P., Santana, K., and Lima, A. H. (2020). Targeting shikimate pathway: In silico analysis of phosphoenolpyruvate derivatives as inhibitors of EPSP synthase and DAHP synthase. J. Mol. Graph Model 101, 107735. doi:10.1016/j.jmgm.2020.107735
de Sousa, S. M. R., Guimarães, L., Ferrari, J. L., De Almeida, W. B., and Nascimento, C. S. (2016). A DFT investigation on the host/guest inclusion process of prilocaine into β-cyclodextrin. Chem. Phys. Lett. 652, 123–129. doi:10.1016/j.cplett.2016.04.053
de Souza Farias, S. A., da Costa, K. S., and Martins, J. B. L. (2021). Analysis of conformational, structural, magnetic, and electronic properties related to antioxidant activity: Revisiting flavan, anthocyanidin, flavanone, flavonol, isoflavone, flavone, and flavan-3-ol. ACS Omega 6 (13), 8908–8918. doi:10.1021/acsomega.0c06156
Do Nascimento, L. D., de Moraes, A. A. B., da Costa, K. S., Galúcio, J. M. P., Taube, P. S., Costa, C. M. L., et al. (2020). Bioactive natural compounds and antioxidant activity of essential oils from spice plants: New findings and potential applications. Biomolecules 10 (7), 988–1037. doi:10.3390/biom10070988
Dos Passos Menezes, P., Dos Santos, P. B. P., Dória, G. A. A., de Sousa, B. M. H., Serafini, M. R., Nunes, P. S., et al. (2017). Molecular modeling and physicochemical properties of supramolecular complexes of limonene with α- and β-cyclodextrins. AAPS PharmSciTech 18 (1), 49–57. doi:10.1208/s12249-016-0516-0
El-Saber Batiha, G., Alkazmi, L. M., Wasef, L. G., Beshbishy, A. M., Nadwa, E. H., and Rashwan, E. K. (2020). Syzygium aromaticum L. (Myrtaceae): Traditional uses, bioactive chemical constituents, pharmacological and toxicological activities. Biomolecules 10 (2), 202. doi:10.3390/biom10020202
Enoch, I. V. M. V., and Swaminathan, M. (2005). Dual fluorescence and photoprototropic characteristics of 2-Aminodiphenylsulphone-β-Cyclodextrin inclusion complex. J. Incl. Phenom. Macrocycl. Chem. 53 (3–4), 149–154. doi:10.1007/s10847-005-2633-3
Fonseca, E. C. M., da Costa, K. S., Lameira, J., Alves, C. N., and Lima, A. H. (2020). Investigation of the target-site resistance of EPSP synthase mutants P106T and T102I/P106S against glyphosate. RSC Adv. 10 (72), 44352–44360. doi:10.1039/D0RA09061A
Frisch, M. J., Nathan, A. J., and Scobell, A., (2009). Gaussian 09. Wallingford CT: Gaussian, Inc., 2111–2113. Gaussian Inc.: Wallingford CT.
Galúcio, J. M., Monteiro, E. F., de Jesus, D. A., Costa, C. H., Siqueira, R. C., Santos, G. B. D., et al. (2019). In silico identification of natural products with anticancer activity using a chemo-structural database of Brazilian biodiversity. Comput. Biol. Chem. 83, 107102. doi:10.1016/j.compbiolchem.2019.107102
Gao, S., Jiang, J., Li, X., Liu, Y., Zhao, L., Fu, Y., et al. (2020). Enhanced physicochemical properties and herbicidal activity of an environment-friendly clathrate formed by β-cyclodextrin and herbicide cyanazine. J. Mol. Liq. 305, 112858. doi:10.1016/j.molliq.2020.112858
Gapsys, V., Pérez-Benito, L., Aldeghi, M., Seeliger, D., van Vlijmen, H., Tresadern, G., et al. (2020). Large scale relative protein ligand binding affinities using non-equilibrium alchemy. Chem. Sci. 11 (4), 1140–1152. doi:10.1039/C9SC03754C
Gong, L., Li, T., Chen, F., Duan, X., Yuan, Y., Zhang, D., et al. (2016). An inclusion complex of eugenol into β-cyclodextrin: Preparation, and physicochemical and antifungal characterization. Food Chem. 196, 324–330. doi:10.1016/j.foodchem.2015.09.052
Hadian, Z., Maleki, M., Abdi, K., Atyabi, F., Mohammadi, A., and Khaksar, R. (2018). Preparation and characterization of nanoparticle β-cyclodextrin:geraniol inclusion complexes. Iran. J. Pharm. Res. 17 (1), 39–51.
Hansson, T., Marelius, J., and Åqvist, J. (1998). Ligand binding affinity prediction by linear interaction energy methods. J. Comput. Aided Mol. Des. 12 (1), 27–35. doi:10.1023/A:1007930623000
Hestenes, M. R., and Stiefel, E. (1952). Methods of conjugate gradients for solving linear systems. J. Res. Natl. Bur. Stand (1934) 49 (6), 409. doi:10.6028/jres.049.044
Higuera, A., and Ramírez, J. D. (2019). Molecular epidemiology of dengue, yellow fever, zika and chikungunya arboviruses: An update. Acta Trop. 190, 99–111. doi:10.1016/j.actatropica.2018.11.010
Jaganathan, S. K., Mazumdar, A., Mondhe, D., and Mandal, M. (2011). Apoptotic effect of eugenol in human colon cancer cell lines. Cell Biol. Int. 35 (6), 607–615. doi:10.1042/CBI20100118
Jansook, P., Ogawa, N., and Loftsson, T. (2018). Cyclodextrins: Structure, physicochemical properties and pharmaceutical applications. Int. J. Pharm. 535 (1–2), 272–284. doi:10.1016/j.ijpharm.2017.11.018
Jiang, L., Yang, J., Wang, Q., Ren, L., and Zhou, J. (2019). Physicochemical properties of catechin/β-cyclodextrin inclusion complex obtained via Co-precipitation. CyTA - J. Food 17 (1), 544–551. doi:10.1080/19476337.2019.1612948
Joardar, A., Meher, G., Bag, B. P., and Chakraborty, H. (2020). Host-guest complexation of eugenol in cyclodextrins for enhancing bioavailability. J. Mol. Liq. 319, 114336. doi:10.1016/j.molliq.2020.114336
Jorgensen, W. L., Chandrasekhar, J., Madura, J. D., Impey, R. W., and Klein, M. L. (1983). Comparison of simple potential functions for simulating liquid water. J. Chem. Phys. 79 (2), 926–935. doi:10.1063/1.445869
Jorgensen, W. L., Maxwell, D. S., and Tirado-Rives, J. (1996). Development and testing of the OPLS all-atom force field on conformational energetics and properties of organic liquids. J. Am. Chem. Soc. 118 (45), 11225–11236. doi:10.1021/ja9621760
Kamatou, G. P., Vermaak, I., and Viljoen, A. M. (2012). Eugenol—from the remote maluku islands to the international market place: A review of a remarkable and versatile molecule. Molecules 17 (6), 6953–6981. doi:10.3390/molecules17066953
Kayaci, F., Ertas, Y., and Uyar, T. (2013). Enhanced thermal stability of eugenol by cyclodextrin inclusion complex encapsulated in electrospun polymeric nanofibers. J. Agric. Food Chem. 61 (34), 8156–8165. doi:10.1021/jf402923c
Kayaci, F., and Uyar, T. (2011). Solid inclusion complexes of vanillin with cyclodextrins: Their formation, characterization, and high-temperature stability. J. Agric. Food Chem. 59 (21), 11772–11778. doi:10.1021/jf202915c
Kfoury, M., Landy, D., and Fourmentin, S. (2018). Characterization of cyclodextrin/volatile inclusion complexes: A review. Molecules 23 (5), 1204. doi:10.3390/molecules23051204
Kirschner, K. N., Yongye, A. B., Tschampel, S. M., González-Outeiriño, J., Daniels, C. R., Foley, B. L., et al. (2008). GLYCAM06: A generalizable biomolecular force field. Carbohydrates. J. Comput. Chem. 29 (4), 622–655. doi:10.1002/jcc.20820
Kuskoski, E. M., Vega, J. M., Rios, J. J., Fett, R., Troncoso, A. M., and Asuero, A. G. (2003). Characterization of anthocyanins from the fruits of baguaçu ( eugenia umbelliflora berg). J. Agric. Food Chem. 51 (18), 5450–5454. doi:10.1021/jf030014z
Lee, J., Lee, S.-S., Lee, S., and Oh, H. B. (2020). Noncovalent complexes of cyclodextrin with small organic molecules: Applications and insights into host–guest interactions in the gas phase and condensed phase. Molecules 25 (18), 4048. doi:10.3390/molecules25184048
Loftsson, T., and Brewster, M. E. (1996). Pharmaceutical applications of cyclodextrins. 1. Drug solubilization and stabilization. J. Pharm. Sci. 85 (10), 1017–1025. doi:10.1021/js950534b
Lupi, E., Hatz, C., and Schlagenhauf, P. (2013). The efficacy of repellents against Aedes, Anopheles, Culex and ixodes spp. – a literature review. Travel Med. Infect. Dis. 11 (6), 374–411. doi:10.1016/j.tmaid.2013.10.005
Mahboub, R. (2014). Structural conformational study of eugenol derivatives using semiempirical methods. Adv. Chem. 2014, 1–5. doi:10.1155/2014/490358
Mapossa, A. B., Focke, W. W., Tewo, R. K., Androsch, R., and Kruger, T. (2021). Mosquito-repellent controlled-release formulations for fighting infectious diseases. Malar. J. 20 (1), 165. doi:10.1186/s12936-021-03681-7
Mateen, S., Shahzad, S., Ahmad, S., Naeem, S. S., Khalid, S., Akhtar, K., et al. (2019). Cinnamaldehyde and eugenol attenuates collagen induced arthritis via reduction of free radicals and pro-inflammatory cytokines. Phytomedicine 53, 70–78. doi:10.1016/j.phymed.2018.09.004
Methods, D. (2021). Venugopal jayapal mahatma gandhi medical college & research Institute. Pillaiyar Kuppam - 607402, Puducherry, India 12 (1), 330–335. doi:10.13040/IJPSR.0975-8232.12(1).330-35
Miot, H. A., Lauterbach, G. d. P., Ribeiro, F. A. H., Júnior, F. É. L., Hercos, G. N., Madeira, N. G., et al. (2011). Comparison among homemade repellents made with cloves, picaridin, andiroba, and soybean oil against Aedes aegypti bites. Rev. Soc. Bras. Med. Trop. 44 (6), 793–794. doi:10.1590/S0037-86822011000600030
Montalvo-Acosta, J. J., Pacak, P., Gomes, D. E. B., and Cecchini, M. (2018). A linear interaction energy model for cavitand host–guest binding affinities. J. Phys. Chem. B 122 (26), 6810–6814. doi:10.1021/acs.jpcb.8b03245
Moyer, R. A., Hummer, K. E., Finn, C. E., Frei, B., and Wrolstad, R. E. (2002). Anthocyanins, phenolics, and antioxidant capacity in diverse small fruits: Vaccinium , rubus , and ribes. J. Agric. Food Chem. 50 (3), 519–525. doi:10.1021/jf011062r
Mustafa, S. F. Z., Arsad, S. R., Mohamad, H., Abdallah, H. H., and Maarof, H. (2021). Host-guest molecular encapsulation of cucurbit[7]Uril with dillapiole congeners using docking simulation and density functional theory approaches. Struct. Chem. 32 (3), 1151–1161. doi:10.1007/s11224-020-01708-4
Muthu Vijayan Enoch, I. V., and Swaminathan, M. (2004). Inclusion complexation of 2-amino-7-bromofluorene by β-cyclodextrin: Spectral characteristics and the effect of PH. J. Fluoresc. 14 (6), 751–756. doi:10.1023/B:JOFL.0000047226.60559.09
Newman, D. J., and Cragg, G. M. (2020). Natural products as sources of new drugs over the nearly four decades from 01/1981 to 09/2019. J. Nat. Prod. 83 (3), 770–803. doi:10.1021/acs.jnatprod.9b01285
Paixão, E. S., Teixeira, M. G., and Rodrigues, L. C. (2018). Zika, chikungunya and dengue: The causes and threats of new and reemerging arboviral diseases. BMJ Glob. Health 3, e000530–e000536. doi:10.1136/bmjgh-2017-000530
Pena, G. A., da Costa Lopes, A. S., de Morais, S. H. S., do Nascimento, L. D., dos Santos, F. R. R., da Costa, K. S., et al. (2022). Host-guest inclusion complexes of natural products and nanosystems: Applications in the development of repellents. Molecules 27 (8), 2519. doi:10.3390/molecules27082519
Piletti, R., Bugiereck, A. M., Pereira, A. T., Gussati, E., Dal Magro, J., Mello, J. M. M., et al. (2017). Microencapsulation of eugenol molecules by β-cyclodextrine as a thermal protection method of antibacterial action. Mater Sci. Eng. C Mater Biol. Appl. 75, 259–271. doi:10.1016/j.msec.2017.02.075
Ray, A. (2015). Reception of odors and repellents in mosquitoes. Curr. Opin. Neurobiol. 34, 158–164. doi:10.1016/j.conb.2015.06.014
Rayan, A., Raiyn, J., and Falah, M. (2017). Nature is the best source of anticancer drugs: Indexing natural products for their anticancer bioactivity. PLoS One 12 (11), e0187925. doi:10.1371/journal.pone.0187925
Rezaeisadat, M., Salehi, N., and Bordbar, A.-K. (2021). Inclusion of levodopa into β-cyclodextrin: A comprehensive computational study. ACS Omega 6 (37), 23814–23825. doi:10.1021/acsomega.1c02637
Ribeiro, A., Figueiras, A., Santos, D., and Veiga, F. (2008). Preparation and solid-state characterization of inclusion complexes formed between miconazole and methyl-beta-cyclodextrin. AAPS PharmSciTech 9 (4), 1102–1109. doi:10.1208/s12249-008-9143-8
Rifai, E. A., van Dijk, M., and Geerke, D. P. (2020). Recent developments in linear interaction energy based binding free energy calculations. Front. Mol. Biosci. 7, 114. doi:10.3389/fmolb.2020.00114
Rodríguez, F. J., Guarda, A., Galotto, M., and J. Galotto, M. (2021). Beta-Cyclodextrin: Eugenol inclusion complexes: Characterization and antifungal capacity. ESPOCH Congr. Ecuadorian J. S.T.E.A.M. 1 (1), 463–477. doi:10.18502/espoch.v1i1.9584
Roe, D. R., and Cheatham, T. E. (2013). PTRAJ and CPPTRAJ: Software for processing and analysis of molecular dynamics trajectory data. J. Chem. Theory Comput. 9 (7), 3084–3095. doi:10.1021/ct400341p
Roth-Walter, F., Moskovskich, A., Gomez-Casado, C., Diaz-Perales, A., Oida, K., Singer, J., et al. (2014). Immune suppressive effect of cinnamaldehyde due to inhibition of proliferation and induction of apoptosis in immune cells: Implications in cancer. PLoS One 9 (10), e108402. doi:10.1371/journal.pone.0108402
Saha, S., Roy, A., Roy, K., and Roy, M. N. (2016). Study to explore the mechanism to form inclusion complexes of β-cyclodextrin with vitamin molecules. Sci. Rep. 6 (1), 35764. doi:10.1038/srep35764
Santana, K., do Nascimento, L. D., Lima e Lima, A., Damasceno, V., Nahum, C., Braga, R. C., et al. (2021). Applications of virtual screening in bioprospecting: Facts, shifts, and perspectives to explore the chemo-structural diversity of natural products. Front. Chem. 9, 662688. doi:10.3389/fchem.2021.662688
Scremin, F. R., Veiga, R. S., Silva-Buzanello, R. A., Becker-Algeri, T. A., Corso, M. P., Torquato, A. S., et al. (2018). Synthesis and characterization of protein microcapsules for eugenol storage. J. Therm. Anal. Calorim. 131 (1), 653–660. doi:10.1007/s10973-017-6302-8
Seo, E.-J., Min, S.-G., and Choi, M.-J. (2010). Release characteristics of freeze-dried eugenol encapsulated with β-cyclodextrin by molecular inclusion method. J. Microencapsul. 27 (6), 496–505. doi:10.3109/02652041003681398
Singh, R. B., Mahanta, S., and Guchhait, N. (2010). Spectral modulation of charge transfer fluorescence probe encapsulated inside aqueous and non-aqueous β-cyclodextrin nanocavities. J. Mol. Struct. 963 (1), 92–97. doi:10.1016/j.molstruc.2009.10.024
Stepniak, A., Belica-Pacha, S., Rozalska, S., Dlugonski, J., Urbaniak, P., and Palecz, B. (2015). Study on a host–guest interaction of β-cyclodextrin with tebuconazole in water. J. Mol. Liq. 211, 288–293. doi:10.1016/j.molliq.2015.07.023
Szejtli, J. (1998). Introduction and general overview of cyclodextrin Chemistry. Chem. Rev. 98 (5), 1743–1754. doi:10.1021/cr970022c
Tabanca, N., Bernier, R. U., Agramonte, M. N., Tsikolia, M., and Bloomquist, R. J. (2016). Discovery of repellents from natural products. Curr. Org. Chem. 20 (25), 2690–2702. doi:10.2174/1385272820666160421151503
Tan, K., Faierstein, G. B., Xu, P., Barbosa, R. M. R., Buss, G. K., and Leal, W. S. (2019). A popular Indian clove-based mosquito repellent is less effective against Culex quinquefasciatus and Aedes aegypti than DEET. PLoS One 14 (11), e0224810. doi:10.1371/journal.pone.0224810
Thomas, A., Mazigo, H. D., Manjurano, A., Morona, D., and Kweka, E. J. (2017). Evaluation of active ingredients and larvicidal activity of clove and cinnamon essential oils against Anopheles gambiae (sensu lato). Parasit. Vectors 10 (1), 411. doi:10.1186/s13071-017-2355-6
Trott, O., and Olson, A. J. (2009). AutoDock Vina: Improving the speed and accuracy of docking with a new scoring function, efficient optimization, and multithreading. J. Comput. Chem. 31 (2), 455–461. NA-NA. doi:10.1002/jcc.21334
Venuti, V., Rossi, B., D’Amico, F., Mele, A., Castiglione, F., Punta, C., et al. (2015). Combining Raman and infrared spectroscopy as a powerful tool for the structural elucidation of cyclodextrin-based polymeric hydrogels. Phys. Chem. Chem. Phys. 17 (15), 10274–10282. doi:10.1039/C5CP00607D
Vestland, T. L., Jacobsen, Ø., Sande, S. A., Myrset, A. H., and Klaveness, J. (2015). Compactible powders of omega-3 and β-cyclodextrin. Food Chem. 185, 151–158. doi:10.1016/j.foodchem.2015.03.132
Wang, J., Cao, Y., Sun, B., and Wang, C. (2011). Physicochemical and release characterisation of garlic oil-β-cyclodextrin inclusion complexes. Food Chem. 127 (4), 1680–1685. doi:10.1016/j.foodchem.2011.02.036
Wang, J., Cieplak, P., and Kollman, P. A. (2000). How well does a restrained electrostatic potential (RESP) model perform in calculating conformational energies of organic and biological molecules? J. Comput. Chem. 21 (12), 1049–1074. doi:10.1002/1096-987x(200009)21:12<1049:aid-jcc3>3.0.co;2-f
Wang, J., Wolf, R. M., Caldwell, J. W., Kollman, P. A., and Case, D. A. (2004). Development and testing of a general amber force field. J. Comput. Chem. 25 (9), 1157–1174. doi:10.1002/jcc.20035
Wüpper, S., Lüersen, K., and Rimbach, G. (2021). Cyclodextrins, natural compounds, and plant bioactives—A nutritional perspective. Biomolecules 11 (3), 401–421. doi:10.3390/biom11030401
Xu, J. G., Liu, T., Hu, Q. P., and Cao, X. M. (2016). Chemical composition, antibacterial properties and mechanism of action of essential oil from clove buds against Staphylococcus aureus. Molecules 21 (9), 1194–1213. doi:10.3390/molecules21091194
Xu, W., Li, X., Wang, L., Li, S., Chu, S., Wang, J., et al. (2021). Design of cyclodextrin-based functional systems for biomedical applications. Front. Chem. 9, 635507. doi:10.3389/fchem.2021.635507
Yang, Y., and Song, L. X. (2005). Study on the inclusion compounds of eugenol with α-β-γ- and heptakis (2, 6-di-O-Methyl)-β-Cyclodextrins. J. Incl. Phenom. Macrocycl. Chem. 53 (1–2), 27–33. doi:10.1007/s10847-005-0247-4
Yang, Z., Yao, X., Xiao, Z., Chen, H., and Ji, H. (2016). Preparation and release behaviour of the inclusion complexes of phenylethanol with β-cyclodextrin. Flavour Fragr. J. 31 (3), 206–216. doi:10.1002/ffj.3302
Zheng, D., Xia, L., Ji, H., Jin, Z., and Bai, Y. (2020). A cyclodextrin-based controlled release system in the simulation of in vitro small intestine. Molecules 25 (5), 1212. doi:10.3390/molecules25051212
Keywords: repellents, nanoencapsulation, eugenol, molecular dynamics, molecular modeling
Citation: Freitas CABd, Costa CHS, da Costa KS, da Paz SPA, Silva JRA, Alves CN and Lameira J (2023) Assessment of host–guest molecular encapsulation of eugenol using β-cyclodextrin. Front. Chem. 10:1061624. doi: 10.3389/fchem.2022.1061624
Received: 05 October 2022; Accepted: 06 December 2022;
Published: 09 January 2023.
Edited by:
William Tiznado, Andres Bello University, ChileReviewed by:
Venkatesan Thimmakondu, San Diego State University, United StatesCopyright © 2023 Freitas, Costa, da Costa, da Paz, Silva, Alves and Lameira. This is an open-access article distributed under the terms of the Creative Commons Attribution License (CC BY). The use, distribution or reproduction in other forums is permitted, provided the original author(s) and the copyright owner(s) are credited and that the original publication in this journal is cited, in accordance with accepted academic practice. No use, distribution or reproduction is permitted which does not comply with these terms.
*Correspondence: Jerônimo Lameira, lameira@ufpa.br
†ORCID: Camila Auad Beltrão de Freitas, orcid.org/0000-0003-1765-7925; Clauber Henrique Souza da Costa, orcid.org/0000-0002-6915-1056; Simone Patrícia Aranha da Paz, orcid.org/0000-0002-5880-7638; Cláudio Nahum Alves, orcid.org/0000-0001-6576-4229; José Rogério A. Silva: orcid.org/0000-0003-2310-5107; Kauê S. da Costa, orcid.org/0000-0002-2735-8016; Jerônimo Lameira: orcid.org/0000-0001-7270-1517
Disclaimer: All claims expressed in this article are solely those of the authors and do not necessarily represent those of their affiliated organizations, or those of the publisher, the editors and the reviewers. Any product that may be evaluated in this article or claim that may be made by its manufacturer is not guaranteed or endorsed by the publisher.
Research integrity at Frontiers
Learn more about the work of our research integrity team to safeguard the quality of each article we publish.