- 1School of Biochemical Engineering, IIT BHU, Varanasi, India
- 2Department of Ageing Research, Manipal School of Life Sciences, Manipal Academy of Higher Education, Manipal, Karnataka, India
- 3Department of Biotechnology, Koneru Lakshmaiah Education Foundation, Vaddeswaram, Andhra Pradesh, India
Over the past few decades, various bioactive material-based scaffolds were investigated and researchers across the globe are actively involved in establishing a potential state-of-the-art for bone tissue engineering applications, wherein several disciplines like clinical medicine, materials science, and biotechnology are involved. The present review article’s main aim is to focus on repairing and restoring bone tissue defects by enhancing the bioactivity of fabricated bone tissue scaffolds and providing a suitable microenvironment for the bone cells to fasten the healing process. It deals with the various surface modification strategies and smart composite materials development that are involved in the treatment of bone tissue defects. Orthopaedic researchers and clinicians constantly focus on developing strategies that can naturally imitate not only the bone tissue architecture but also its functional properties to modulate cellular behaviour to facilitate bridging, callus formation and osteogenesis at critical bone defects. This review summarizes the currently available polymeric composite matrices and the methods to improve their bioactivity for bone tissue regeneration effectively.
1 Introduction
Tissue engineering makes an attempt to recover, retain, strengthen, or significantly boost tissue functionality when ailment or trauma hampers it. To accomplish the above, novel biological alternative substitutes must be established (Baptista and Guedes, 2021). Even though bone tissue may quickly regenerate, when disease, injury, or infection impairs this ability, new approaches are needed to aid bone regeneration (Amini et al., 2012). Bone tissue engineering focuses on methods to repair damaged bone by creating substitute structures that can perform the protective and structural functions of healthy bone while sustaining stresses placed on the human body from inside and outside (Amini et al., 2012). For bone healing to occur, the generated BTE matrix must simultaneously promote cell growth and the synthesis of new bone tissue (Amini et al., 2012). The most often transplanted human tissue, after blood, is bone (Shegarfi and Reikeras, 2009). Therefore, bone autografts, in which a portion of a patient’s normal bone tissue is surgically removed and relocated to the afflicted region, are still the gold standard approach for BTE. Although this operation is simple to perform, it has drawbacks such as a limited supply, a risk of rejection, and morbidities at the donor site (Nuss and von Rechenberg, 2008). Researchers have created new approaches to address these issues, including biocompatible permanent metal implants. However, some individuals are at risk for rejection, necessitating additional surgical procedures to remove these implants. Additionally, they have incompatible mechanical characteristics that might cause stress shielding and fracture or fatigue failure modes (Nuss and von Rechenberg, 2008).
However, ceramic implants can be used temporarily and permanently for such conditions. Yet, polymeric and composite materials have recently been the focus of research and development (Skorulska et al., 2021). Almost all polymeric materials and their composites are biologically compatible, bio-resorbable, and also have Young’s modulus interoperable with bone. It implies that once transplanted, they will promote the bone regeneration process and gradually be overtaken by newly formed tissue, leaving no scars once the process of bone healing is complete (Roseti et al., 2017a). Some other alternative choice is ceramic and bio-polymeric composites, which combines the functions of a polymer network reinforced with biologically active ceramic particles. These materials outperform others in terms of performance and mechanical behaviour, including osteoconductive effects (Wang et al., 2017). The scaffold (Do et al., 2015; Wang et al., 2020), a three-dimensional biological or synthetic artificial structure used to support bone compressive damages and allow bone tissue repair and re-growth (Roseti et al., 2017a; Wang et al., 2017), is the principal temporary treatment for BTE. For BTE to be successfully applied, four key scaffold characteristics must be met: biological, structural, related material composition, and regarding production procedures. The resultant materials have outstanding osteoinductivity, osteoconductivity, and biocompatibility as potential benefits, offering a promising new technique for bone healing. Scaffold material composition is essential in creating artificial bone because they serve as the physical foundation for artificial grafts (Noori et al., 2017). The ideal and effective scaffold material should include attributes comparable to native bone, ensuring favourable physiochemical surroundings and biomechanical assistance for seed cell attachment, migration, multiplication, osteogenic differentiation potential, and neo-angiogenesis on the scaffold framework.
Last but not least, it must permit gradual integration into the host tissue throughout the healing process to support regular loads (Mishra et al., 2016; Roseti et al., 2017b). To be organically digested, scaffold degradation products also need to be non-toxic and non-immunogenic. Mesenchymal stem cells homing, osteoblast differentiation, extracellular matri and osteoid mineralization, and the development of terminally differentiated osteocytes all play significant roles in bone formation during the regeneration process (Wang et al., 2013a).
Several materials are being utilized to fabricate bone tissue scaffolds, including both natural and synthetic polymers. Researchers incorporate various bioactive molecules, inorganic materials like hydroxyapatite, bioactive glass, and metallic and non-metallic nanoparticles to enhance these scaffolds’ bioactivity. Since inorganic materials bioglass possesses elastic modulus similar to the cortical bone, they are widely used in the fabrication process. Whereas natural polymers possess less than 70 MPa compressive strength, and synthetic polymers exhibit about 10 GPa (Gunatillake et al., 2003; Pilipchuk et al., 2015; Chocholata et al., 2019). Therefore, they are combined to form a replicating matrix for bone tissue. With the help of bioactive scaffolds, the delivery of drugs and growth factors has been enhanced, which helps in the overall bone healing process and improves bone regeneration.
Bioactivity of the scaffolds is the ability to influence the scaffold’s biological surroundings. Enhancing the capacity for the formation of apatite layer, differentiation of osteoblast cells, and the formation of bone ECM falls under this category. Because of their superior bioactivity and ability to form strong bonds with the host bone tissue, modern bioceramics including hydroxyapatite, tricalcium phosphate, composite of HAp/TCP, and 45S5® bioglass are broadly employed as bone biomaterials. Their comparatively poor mechanical strength, especially their lower toughness value, which restricts their application to just low-load bearing parts of the human body, is these bioceramics’ principal drawback. Understanding the attachment of ceramics to living bone and methods for testing the bonding potential is crucial for the development of neo-bioactive ceramics for load-bearing based bone tissue restoration (Wu and Xiao, 2009a).
Recently researchers have started exploring the potential of 3D scaffolds for tissue regeneration. They have developed a number of applications for these scaffolds, with researchers hypothesising that these 3D scaffolds will possess high structural stability and they will also provide a 3D microenvironment for tissue regeneration, mimicking the functionality of natural tissue. When these scaffolds are implanted, they create a local bioactive environment that helps the injured or missing tissue to recover. Synthetic and natural polymers have been widely used as biomaterials to create these scaffolds, in large part because of their enormous diversity (Stratton et al., 2016).
The natural materials include polysaccharides like chitosan, hyaluronic acid, gelatin, starch, etc. Likewise, collagen, fibrin gels, silk fibroin, etc., also help cell adhesion. The only limitation is their mechanical strength and pathogenic impurities that can result in immunogenicity. However, synthetic polymers like poly lactic acid, polyvinyl alcohol, polyglycolic acid, etc., and their copolymers, on the other hand, are utilized widely in scaffold development due to their tunable mechanical properties and degradation rate control. Inorganic materials include tricalcium phosphate, metals, HAp, and their combinations replicating the bone mineral phase.
However, the development of hydrogels and scaffolds with better bioactivity, osteoconductivity and osseointegration capabilities in combination with high toughness value is very difficult to achieve. A team of researchers developed a robust and mechanically tougher osteoconductive hydrogel in a recent study. They followed a single-step micellar copolymerization of acrylamide and urethacrylate dextran after which they performed the in-situ mineralization of Hap nanocrystals. They demonstrated that the mineralized HAp concurrently enhances the mechanical and osteoconductivity properties. In comparison to pure PAAm hydrogels, the resulting HAp mineralized PAAm/Dex-U hydrogel (HAp-PADH) possessed improved fracture resistance of around 2300 Jm2 and an unusually high compressive strength of 6.5 MPa. They showed the enhanced osteogenic differentiation potential of the mineralized HAp layer that improved the osteoblast cell adhesion and proliferation in-vitro. They have concluded that HAp combination with PADH possesses an excellent potential for bone tissue repair and regeneration in-vivo when tested in a rabbit model of femoral condyle defect.
Similarly in another study, the synergistic effects of bioactive glass and halloysite nanotubes (i.e., BG/HNT) were assessed over the physicochemical and bioactive properties of polyacrylamide/poly (vinyl alcohol) (PMPV) based nanocomposite hydrogels. They applied the in-situ free-radical polymerization and a freeze-thaw method to successfully create a double-network hydrogel made up of organic-inorganic components. Bioactivity was tested for the developed scaffolds after immersion in the simulated bodily fluid (Ca/P: 1.21 0.14) and noticeably improved dynamic mechanical characteristics with compressive strength of 102.1 kPa at 45% of strain and stiffness of 3115.0 N/m at 15% of strain were revealed with enhanced biomineralization capacity of PMPV/BG/HNT. They demonstrated hFOB1.19 osteoblast cells growth and attachment over the developed hydrogels and concluded their efficacy for low-load bearing bone tissue.
Several researchers have applied additive manufacturing, bone-healing materials and functionally graded structures for bone regeneration and treatment of bone tumours. In a recent study, a team has developed novel AM-prepared polyvinyl alcohol/sodium alginate/hydroxyapatite hydrogel composite scaffolds at low temperatures. For which they placed different concentrations of magnetic graphene oxide/Fe3O4 nanoparticles onto the scaffolds. Characterization of the developed scaffolds showed their improved moldability and stronger hydrogen bonds between the composite material. They demonstrated that by adjusting the MGO’s composition and the strength of an external alternating magnetic field, thermal effects can be controlled. They tested in-vitro bone mesenchymal stem cell activity over these scaffolds which showed improved cell growth while the in-vivo study was performed which showed that the scaffolds exhibited favourable anti-tumour properties and efficient magnetothermal conversion in-vivo.
Bioceramics also play an essential role in improving the poor osteogenic characteristics and mechanical properties of the scaffolds for bone tissue repair and regeneration. Recent study conducted by a team demonstrated the application of free radical polymerization, to develop CaO.40SiO2.(12-y)P2O5.ySeO3.5MgO hydrogels containing polyacrylamide-carboxymethylcellulose. They enhanced the poor osteogenic property, controlled the rate of breakdown, and improved the mechanical stability by adding strontium and selenium doped xSrO. They achieved considerable apatite growth on the first day, and samples doped with bioceramics displayed outstanding bioactive behaviour, which was considered a factor for bone tissue regeneration and bone repair from flaws. Hydrogels impregnated with strontium and selenium and doped bioceramics showed an inhibitory effect on the human osteosarcoma MG63 cell line. Selenium and strontium-doped bioceramics offered a favourable environment for the cellular proliferation, adhesion, and excellent alkaline phosphatase activity of the MC3T3-E1 osteoblast cell line. Synthesized hydrogels represented unique osteogenic capabilities and therefore they may be used for bone cancer patients’ recovery as well as for the regeneration of hard tissues.
This article discusses in detail the fabrication of composite matrix and the potential ways for enhancing the bioactivity of scaffolds by doping and its advantages.
2 Bone tissue: ECM and its healing mechanism
Bone regeneration is the process of replacing bone tissue that has been damaged or lost due to trauma, injuries, cancer, or congenital defects. The extracellular space of the bone is filled with the ECM, a non-cellular, 3D material that cells release. Particular proteins and carbohydrates make up its structure. It is a complex, dynamic bio-environment with carefully controlled mechanical and biochemical properties. ECMs play a role in controlling cell attachment, proliferation, and responses to growth hormones in bone. They also significantly affect differentiation and, subsequently, the structural and functional attributes of the mature bone. Osteoblast-lineage cells, including MSCs, osteoblasts, and osteocytes, as well as osteoclasts, can both produce new bone and absorb existing bone when exposed to bone ECM. As bone regenerative medicine has advanced, researchers have become more interested in the osteoinductive and osteoconductive potential of ECM-based polymeric scaffolds.
Each type of tissue’s ECM develops with distinct composition and architecture (Frantz et al., 2010). The ECM provides consistency and flexibility to the body tissues and organs in terms of controlling their growth, activity, and homeostasis. It is constantly restructured as the wide range of receptors, growth regulators, and the pH of the native surrounding changes (Bonnans et al., 2014; Mouw et al., 2014). The fourth component in the evolution of BTE is thought to be the ECM (Ravindran et al., 2012). 60% of the bone matrix is made up of inorganic, and 40% of it is organic substances.
2.1 Major components of bone ECM
2.1.1 Organic ECM
Collagenous proteins are found in collagen. The organic ECM in bones is mostly composed of collagen types I, III, and V. The principal function of collagens is to supply mechanical stability and support but to also act as a framework for osteocytes (Saito and Marumo, 2015). 90% of the collagen in bone tissue is type I collagen, which assembles into triple helices of polypeptides to create collagen fibrils. In order to create higher-order fibril bundles and fibers, these fibrils engage in interactions with other collagenous and non-collagenous proteins (Varma et al., 2016). Less common collagen types III and V control the size of type I collagen fibers and the process of fibrillogenesis (Garnero, 2015). The mechanical characteristics of collagen keep the polypeptide chains in a neatly structured fibril framework, depending on the inter and intra-chain crosslinks. Bone strength is significantly influenced by collagen. The ECM is altered by type I collagen deficiency or collagen structural mutations, which significantly raises the risk of fracture (Fonseca et al., 2014).
2.1.2 Non-collagenous protein: Proteoglycans
Proteoglycans are defined as glycosaminoglycan (GAG) residues that have been covalently attached to the core of the protein molecule. Keratan sulfate, chondroitin sulfate, heparan sulfate, and dermatan sulfate are among the six varieties of GAG residues discovered in proteoglycans (Walimbe and Panitch, 2020). The bone has a significant family of small leucine-rich proteoglycans including biglycan, decorin, keratocan, and asporin. SLRPs are extracellular proteins secreted by cells that collaborate with cell-surface receptors and cytokines to regulate both healthy and unhealthy cellular functions. SLRPs are involved in all phases of bone development, such as cellular multiplication, osteogenesis, mineral deposition, and bone remodelling (Kirby and Young, 2018). SLRPs also control the collagen fibrillogenesis process. Dysregulation results in flaws in the structure and production of collagen and leads to fibrosis brought on by either orthopaedic traumas or genetic deficiencies (Moorehead et al., 2019). They control the collagen fibrillogenesis process, whose dysregulation results in flaws in the structure and production of collagen and leads to fibrosis brought on by either orthopaedic traumas or genetic deficiencies (Moorehead et al., 2019). The class I SLRPs biglycan and decorin each comprise dermatan or chondroitin sulfate GAG chains. Biglycan is expressed throughout the growth and mineralization of cells, whereas decorin is continually expressed once the bone matrix has begun to form. Keratocan plays a role in controlling the pace of mineral deposition and bone formation. It is mostly expressed in osteoblasts (Coulson-Thomas et al., 2015). It has been demonstrated that type I collagen and asporin, another SLRP component, bind together to induce collagen mineralization (Kalamajski et al., 2009). As a result, SLRPs are crucial for preserving bone homeostasis.
2.1.3 Inorganic ECM
HA is a substance that promotes the production of bone tissue and is simple to combine with polymeric materials (Amini et al., 2012). Since the 1950s, HAp has been employed as an unreactive scaffold for filling and processing bone abnormalities in regenerative scientific knowledge (Dubok, 2000). Due to its strong osteoconductivity and biocompatibility, the calcium phosphate bio-ceramic [Ca10(PO4)6(OH)2] has been ubiquitously used as scaffolds in BTE. It is a well-known bioceramic that is present in significant amounts in bone and teeth (Amornkitbamrung et al., 2020). HAp serves as one of the most extensively utilized bio-ceramics in BTE because it exhibits physiochemical characteristics that are remarkably similar to carbonate apatite, the major inorganic constituent of bone tissue (Yuan et al., 1999; Ripamonti et al., 2009a; Pepla et al., 2014). Due to its advantageous biological characteristics, such as biological compatibility, bio-affinity, bio-activity, and osteoconduction (Habibovic et al., 2008), HAp bioceramics are frequently employed as artificial bone substitutes.
3 Healing of fracture: Mechanism
A fracture is a break in the internal structure of the bone cortex that causes damage to soft tissue. After the fracture, secondary healing starts, and it entails four stages: the development of a hematoma, a fibrocartilaginous callus, a bony callus, and bone remodelling. Up to 10% of all fractures may experience failed or delayed healing, which may be brought on by a variety of conditions, including comminution, infection, malignancy, and interrupted vascular supply. All these processes are discussed below in this article.
3.1 Phases of healing
3.1.1 Hematoma formation
This phase starts right after the fracture from day 1 to day 5. A hematoma forms surrounding the fracture site as a result of the blood arteries supplying the bone and the periosteum being torn during the fracture. The hematoma clots, creating a framework that is eventually used during healing. Pro-inflammatory cytokines such as tumor necrosis factor-alpha, bone morphogenetic proteins, along with interleukins are released as a result of the bone injury (IL-1, IL-6, IL-11, IL-23). These cytokines draw macrophages, monocytes, and lymphocytes by stimulating the vital cellular biology at the location. These cells collaborate in order to remove necrotic, injured tissue and to aid the healing process by releasing cytokines such as vascular endothelial growth factor.
3.1.2 Fibro-cartilaginous callus formation
When VEGF is released, angiogenesis occurs and fibrin-rich granulation tissue begins to form within the hematoma. More mesenchymal stem cells are brought in, where they start to develop into fibroblasts, chondroblasts, and osteoblasts under the control of BMPs between day 5 and day 11. Chondrogenesis begins, resulting in the formation of a collagen-rich fibro-cartilaginous network spanning the fatigue crack ends and a sleeve of hyaline cartilage all around it. The osteoprogenitor cells also lay down a layer of woven bone next to the periosteal layers at the same time.
3.1.3 Bony callus formation
Endochondral ossification starts to take place in the cartilaginous callus between day 11 to day 28. When RANK-L is expressed, it encourages chondroblasts, chondroclasts, osteoblasts, and osteoclasts to differentiate further. As a result, the cartilaginous callus is resorbed and begins to calcify and the patterned woven bone is still being deposited subperiosteally. Mesenchymal stem cells can continue to migrate as the newly created blood arteries continue to multiply. An immature bone callus that is firm and calcified forms at the conclusion of this stage.
3.1.4 Bone remodelling
The phrase “coupled remodelling” refers to the recurring remodelling of the hard callus that occurs as a result of the osteoblasts’ and osteoclasts’ ongoing migration after day 18 and it lasts for months. Osteoclast-driven bone resorption and osteoblast-driven bone formation coexist in this “coupled remodelling” process. Compact bone eventually replaces the callus’s centre, while lamellar bone eventually replaces the callus’ margins. Alongside these changes, the vasculature undergoes significant remodelling. The typical bone structure eventually regenerates after a protracted, months-long process of bone remodelling (Frost, 1989; Ripamonti et al., 2009b; Parvizi, 2010; Marsell and Einhorn, 2011; Kostenuik and Mirza, 2017).
Endochondral ossification is used to describe the transformation of cartilage to bone. As previously stated, this occurs when a bony callus forms, replacing the recently formed, collagen-rich cartilaginous callus with juvenile bone. This process, which involves the bony skeleton substituting the hyaline cartilage prototype, is also required for the foetus to develop long bones. The second type of ossification, intra-membranous ossification, also occurs in the developing foetus. During this process, mesenchymal tissue (primary connective tissue) is transformed straight into bone without cartilage as an intermediary. The flat bones of the cranium are where this process occurs (Berendsen and Olsen, 2015). The whole process from fracture to the complete healing of bone is explained in Figure 1. Figure 2 explains the factors involved in fracture healing including both local and systemic factors.
Given the high mortality and morbidity associated with fractures, an interdisciplinary approach is crucial for successful outcomes (Einhorn, 2005; Bishop et al., 2012; Karpouzos et al., 2017). The interprofessional team can employ various strategies to encourage/promote fracture restoration, such as calcium salts, protein molecules, and vitamins C and D as examples of dietary supplements. Ultrasound, electromagnetic, and electrical bone stimulators are all possible. Further study is needed in this area because it is still unclear whether these strategies are currently effective. Bone is used in a bone graft procedure to act as a framework of the scaffold for the developing bone. The patient’s body (an autograft) or a deceased donor’s body provides this graft (allograft) (Zhang and Williams, 2019). But there are still chances of immune rejection, which may lead to a necessary surgical procedure. For such conditions, ceramic and polymeric composite materials can be a good choice. They are biocompatible, mechanically stable, and also bioresorbable; therefore, they will support bone regeneration. This article discusses natural and synthetic polymeric composite matrices in detail, their advantages, and associated challenges.
4 Natural polymeric composite matrix: Advantages and challenges
TE is a multi-disciplinary field that develops bio-mimetic equivalents that reinstate, maintain, or enhance tissue functioning using precepts and developments from the biomedical sciences and technology (Montoya et al., 2021a). Biomaterials for TE applications can be defined as “a material designed to take a form that can direct, through interactions with living systems, the course of any therapeutic or diagnostic procedure” (Zhang and Kohn, 2012). Polymeric materials are nevertheless the most prevalently used biomaterial category in the fabrication and design of scaffolds for biomedical applications. They are excellent contenders for the construction of artificial bone and other body tissue scaffolds owing to their structural and bio-mechanical properties, appropriate biodegradation rates, and biocompatibility that perfectly approximates those of protein molecules in both soft as well as hard tissues (Mao et al., 2021). Scaffolding frameworks for BTE are porous materials employed to promote cellular attachment, growth, and multiplication while supporting the regeneration of new bone tissue (Lei et al., 2019). The selection of biomaterials or their hybrids used as scaffolds is critical for BTE applications. The usage of different materials and their composites in biomedical treatments has recently grown rapidly. The therapies for various bone-related ailments and disorders using biodegradable materials composites is one domain currently expecting to receive substantial research attention from the scientific community.
Biopolymers, the most widely accepted component of TE used to restore or replace or regenerate injured human body parts, are divided into natural or synthetic polymers (Taghipour et al., 2020). Polymers synthesized by biological systems, including microbial cells, plants, and animals, are classified as natural polymeric materials. Natural polymers have numerous applications, including adhesive bandages, absorbent materials, primed cosmetic products, therapeutic drug delivery, and clinical scaffolds (Titorencu et al., 2017). Natural or biological polymers can further be classified as protein- or polysaccharide-based polymers. The most intensively investigated naturally-derived polymeric materials for BTE are collagen, gelatin, chitosan, hyaluronic acid, silk fibroin, and many more (Kim et al., 2017). These natural polymers have their own sorts of advantages and disadvantages during the bone regeneration process and are briefly discussed in Table 1. These biopolymers have been blended in a plethora of forms, such as 3D porous scaffolds, hydrogels, nanofibrous scaffolds, films, microspheres, sponges, and composites (Han et al., 2017; Kim et al., 2017; Ikono et al., 2019; Filippi et al., 2020; Yao et al., 2020).
Protein-based polymers, unlike polysaccharides, contain amino acid (AA) sequences that are traditionally coupled with cell adhesion via integrin-binding domains or RGD sequences (Guo et al., 2021). As a result, cell attachment and osteoconductivity in polysaccharide-based polymeric scaffolds must be improved through surface chemical functionalization, blending with osteoconductive components, and integration of cell adhesion protein sequences or by blending them with protein-based polymers (Yang et al., 2021). Bio-composites are created by blending two or more biomaterials to improve cytocompatibility and mechanical characteristics of scaffolds for various applications (Goonoo et al., 2013; Basha et al., 2015; Mao et al., 2018). Various studies have been conducted over the last few decades by blending various polymers and other ceramic-based components to achieve the desired cell behaviours and mechanical strength to promote bone tissue renewal and repair, as summarized in Table 1.
5 Synthetic polymer composite matrix: Advantages and challenges
In general, polymeric materials and their composites provide greater control over scaffolds’ physical and chemical properties, including pore size and shape, porosity, hydrophilicity, cytocompatibility, non-toxicity, enzymatic activities, and inflammatory response (Liu and Ma, 2004; Prasad, 2021). The physicochemical properties of scaffolds and the biomaterials used in their construction significantly impact their performance in transplantation, and as a result, various biomaterials are being employed in BTE. The physicochemical and biological attributes are among the factors to consider when selecting biomaterials for therapeutic bone implants. Ceramics, polymeric materials, metals, and various nano-composites are frequently reported classes of materials for hard tissue regeneration (Koons et al., 2020; Ye et al., 2020).
Synthetic polymers were developed and are extensively used in the designing and construction of scaffolds for bone tissue repair and regeneration due to their superior mechanical characteristics. Multiple synthetic materials, such as PLA, PLGA, PVA, and others, have been extensively used for bone tissue regeneration and remodelling (Donnaloja et al., 2020). Also, each type of above discussed synthetic material has its own set of benefits and drawbacks, and high-performance composite polymeric materials are constantly being researched and are discussed in Table 2. They include more possibilities for customizing and modifying their physiochemical behaviour, mechanical stabilities, cell binding domains, and immobilization of different NPs, drugs, or bioactive compounds (Amani et al., 2019). Introducing RGD peptide sequences or growth factors to polymer matrix could further significantly change their properties for cell attachment and proliferation (Zhang et al., 2011; Udomluck et al., 2020).
Furthermore, the polymers’ hydrophilic and hydrophobic properties could be customized to minimize immunogenicity and promote osteoconductivity. For instance, PLGA is lactic acid and glycolic acid copolymer (Castillo-Dalí et al., 2015). Their biodegradation rate is determined by the proportion of lactic acid to glycolic acid; consequently, it could be controllable and modified to meet their specific requirements. This may have an impact on their use in sustaining the delivery of therapeutic drugs and GFs at the site of orthopedic defects (Hines and Kaplan, 2013). Reportedly, NBG-coated PCL/PLGA composite scaffolds demonstrated improved compressive strength, in-vitro biocompatibility, sustained drug release, and increased MC3T3-E1 cell attachment and proliferation (Ensoylu et al., 2021).
6 Smart materials for bone tissue engineering
A biocompatible and biodegradable polymer-based scaffold provides superior mechanical and porous interconnected network, frame, and hierarchy structures with physiochemical properties for cell adhesion, proliferation, and cell-matrix interaction through the porous structures for tissue regeneration in TE. To date, most of the synthetic, natural materials and their composites have been explored for TE applications (Arif et al., 20221081; Biswal, 2021). Several methods and approaches for constructing bio-mimetic scaffolds with control over structure, shape, pore size, porosity, and architectural style for TE application domains have been devised (Turnbull et al., 2018; Kanwar and Vijayavenkataraman, 2021). To date, a wide variety of biomaterials have been employed in tissue engineering, a majority of which lack biocompatible properties and necessitate the cost-effective designing and construction of new smart materials for the highest performance. Smart materials are a material wherein one or more attributes can be changed simply by altering the surrounding environment (Zhang et al., 2018b; Jia et al., 2019). Additionally, smart biomaterials are distinct from conventional biopolymers in that they respond to both external and internal stimuli in their surroundings (Fu et al., 2021). These smart biomaterials can maximize therapeutic potential while minimizing unwanted complications by speedily recognizing and responding to the target tissue surroundings, impart therapeutic value whilst conserving physiologically normal cells and tissues, and thus improve the quality and performance of patient care (Zhang et al., 2018b; Zeng et al., 2019).
Furthermore, Montoya et al. (2021b) notably re-defined the concept of “smart materials” and postulated four levels of material smartness predicated on their inert, active, responsive, and autonomous behaviour. They also divide smart biomaterials into two types: those that respond to internal stimuli, such as surface topography, structural properties, and charge density, and those that respond to external stimuli, such as piezoelectricity, magnetic fields, ionic strength, and enzymes (Montoya et al., 2021b). The aforementioned external/internal stimuli produce heat or enhance osteoblasts to adhere, rapidly proliferate, and differentiate in polymeric scaffolds during bone regeneration and tissue repair. One well-known piezoelectric material with BTE applications is Polyvinylidene fluoride is used in restorative engineering because of its adaptability and cytocompatibility. Fernandes et al. (2019), for example, used a piezoelectric polymer, PVDF, and magnetostrictive CoFe2O4 NPs in a solvent cast method to create 3D magnetoactive porous structures. The use of magnetic stimuli increased the proliferation of preosteoblasts in this study. Bioelectrical signals and internal and external electrical stimulation play important functions in regulating cellular behaviour and bone restoration, and electrically conducting polymeric materials (such as polyaniline, CNTs, polypyrrole, etc.) have received a significant amount of attention as a good source of biomaterials (Guo and Ma, 2018). In the latest study, a construct made of functionalized Multiwalled Carbon Nanotubes, chitosan, and β-Glycerophosphate demonstrated improved electrical conductivity, mechanical stability, less cytotoxicity, and increased ALP activity (Gholizadeh et al., 2017). The preferential catalytic activity of particular enzymes activates enzyme-responsive materials (Ding et al., 2020). The key benefit of ERMs is that their stimulation does not necessitate environmental stimuli because the enzyme-mediated differences exist within the biological system, and enzymatic activities are governed by changes in the physiological environment, resulting in highly beneficial effects in enzymatic reactions (Ding et al., 2020). Zhang et al., for instance, developed a poly(L-lactic acid) nanofibrous construct embedded with a HP/miRNA polyplex enclosed in PLGA microspheres that demonstrated excellent cytocompatibility, non-toxicity, regulated two-stage release of miRNA-26a and in-vivo regrowth of the bone defects (Zhang et al., 2016a).
Moreover, highly developed processing techniques are essential for the production of SBMs. Biopolymers with the relatively low extent of smartness levels can enhance their interrelations with their surroundings and acquire some novelty by using innovative manufacturing methods such as 3D/4D bioprinting, electrospinning, blow spinning, and many other addictive manufacturing techniques. These techniques have attracted wide recognition owing to the potential of producing customized products with governed structure, distinguishable nano- or micro-morphology, and a higher level of alignment with defined physical attributes for BTE (Zhang et al., 2016b; Qasim et al., 2019a). Sharma et al. developed a 3D-printed PLA construct fortified with polydopamine-reduced graphene oxide for new bone tissue development (Sharma et al., 2022a). The study demonstrated the dependence of hMSCs cell growth on fiber direction and nano-coating. In one analysis, electrospun polyurethane urea nanofibrous scaffolds loaded with carboxyl functionalized CNTs (CCNTs)-doped nHAp illustrated superior mechanical properties, excellent cytocompatibility, enhanced expression of osteocalcin and ALP in-vitro, and impressive bone regeneration efficacy and non-toxicity in-vivo (Ghorai et al., 2022). Table 3 shows the recently developed smart materials like photosensitive, stimuli-responsive, electromechanical responsive and other advanced biomaterials with their applications for BTE along with their properties and fabrication technology.
7 Biopolymeric modification for enhanced bone cell adhesion and growth
7.1 Modification strategies
Biomaterials with novel biological functionalities are recognized as promising candidates for bone-regenerating materials. These materials aim to mimic the ECM at the nanoscale by imitating cues in topography, biological factors, and gene transport. Cell adhesion, cell proliferation, spreading, differentiation, and subsequent tissue development are all influenced by surface characteristics such as surface chemistry, net charge, roughness, hardness, and wettability. Thus, many recent studies have addressed surface modification of scaffolds to create physical and chemical properties for cell homing. The goal of biomaterial surface modification is to enhance selectivity. Coating or modifying the surface of biomaterials by a new functional group is one surface modification method. Alternative surface topologies are produced when functional groups are present, resulting in structures with minimum unfavourable interactions. Scaffold architecture is directly correlated to the binding capacity of functional groups, surface topology, as well as cell behaviours. The different types of surface modifications are broadly categorized into chemical modifications and physical modifications (Fabbri and Messori, 2017). Protein and cell adhesion are significantly influenced by the surfaces of polymeric scaffolds. To enable better protein and cell interactions, numerous strategies have been devised to give micrometre to nanometer size modifications in the surface architecture of scaffolds. One of the forthcoming techniques that offer increased biocompatibility while offering a protein delivery vehicle is chemical modification of polymeric scaffold surfaces (Katti et al., 2008). Chemical modification methods include wet chemical reaction (Duan and Wang, 2006), click chemistry (Bayraktar et al., 2012), crosslinkers assisted modification (Yang and Moloney, 2017). Further, the crosslinking-assisted modification encompasses of various crosslinkers like carbodiimide (Ikeda et al., 2010), aldehyde (Lin et al., 2016), ether (Miyagaki et al., 2019) and green crosslinking agents (Hubbe et al., 2015). In order to overcome the main drawbacks associated with chemical methods, which frequently require stringent process control, may result in environmental issues due to the chemical agents used, and may involve undesirable changes in the polymer surface morphology, surface treatment methods based on physical principles have been developed to introduce oxygen-containing functional groups on to polymer surfaces. These functional groups are primarily intended to improve adhesion, wettability, and printability. Today, the industrial level makes extensive use of some of the most popular physical surface modification techniques based on UV radiation (Sionkowska, 2014), plasma-induced treatments (Chu et al., 2002) and laser treatments (Jasso-Gastinel and Kenny, 2016). Different types of polymeric scaffold surface modifications are shown in Figure 3.
7.2 Factors influencing the surface properties
7.2.1 Surface topography
The surface morphology or roughness of the substrate has been shown to influence cell adhesion. The fibres, pores, and pits that make up the surface of biological tissues are just a few examples of the broad range of morphological features found on their surfaces. This influence on cell activity is referred to as “contact guidance,” and a wide range of micro-morphological traits mediates it. Studies in this field have demonstrated that while micro-morphology mainly influences the shape of entire cells, nano-morphology primarily determines the sensing mechanism at the subcellular level. Surface roughness could be subdivided into many categories based on the irregular surface size: macro, micro, submicron, and nanoscale roughness. There is a wide range of cellular responses to surface roughness. Since there is plenty of room for cells to expand and proliferate within macroscopic irregularities, surface roughness has minimal impact on cell attachment behaviour. Surface roughness together at micron and submicron levels have opposing but positive effects on cell adhesion and proliferation. Zhao et al. (2014) discovered that the number of functional MG-63 cells on titanium discs with sub-micron surface roughness was lower than on flat nano-structured materials. Nano-roughness, on the contrary, has been shown to have a beneficial effect on cell adhesion, development, and maturation since it most closely mimics the morphology of real tissues. It has been illustrated that improving a biomaterial’s surface roughness at the nanoscale scale can improve cell adherence and proliferation on the rough surface, at least in human venous endothelial cells (Nguyen et al., 2016; Zhou et al., 2018) Pore size seems to be the key factor controlling cell adhesion. Previous research has demonstrated that nanoscale holes are highly susceptible to the formation of collagen fibers and ECM. In-vivo, larger pores have an impact on cell seeding, dispersion, adhesion, migration, and subsequent neovascularization. Large pore size would inhibit the adherence of cells. Researchers have found that the MG-63 cells adhere efficiently to the membrane’s surface, having a pore size range of 0.2–1 μm, while in the case of membranes with bigger micropores (3.0–8.0 μm), cells appear to be spherical in shape. Furthermore, cells differentiate more extensively when cultured on a membrane with pores between 5.0 and 8.0 nm, with the maximum differentiation occurring on the pore size of 8 nm (Schmitt et al., 2016; CM and O’Brien, 2010; Jeon et al., 2015).
7.2.2 Physical properties
Bone cells, under native conditions, adhere to ECM fibers of varying hardness and flexibility. Bone ECM in-vivo has a rigidity of around 100 GPa. The elasticity and rigidity of fiber ECM are both controlled by the ratio of collagen to elastin. When cells apply stresses to the ECM and detect the ensuing gaps, they gain insight into the mechanical characteristics of the ECM. They can then adjust the local adhesion architecture, cytoskeleton components, and their overall state accordingly (Yang et al., 2017; Padhi and Nain, 2020). Even though cells appear to be more prone to adhere to hydrophilic substrate surface, wettability (hydrophobicity and hydrophilicity) can also influence surface protein adsorption and cell adhesion (Guo et al., 2016). Wei et al. (2022) showed that increasing the contact angle from 0° to 106° reduced the adhesion of osteoblasts. Fibroblast adhesion is significantly greater when the contact angle is between 60° and 80°. As a result, it demonstrates that the physical attributes of the material surface are critical in cell adhesion.
7.2.3 Chemical Properties
Multiple studies have established that surface chemistry plays a vital role in how cells adhere to different substrates. In-vivo, ECM provides cells with a wealth of chemical cues that direct their actions. Surface charges, surface energy, as well as bioactive substances are the most important chemical properties that influence cell adhesion (Kao et al., 2018). To quantify the unsaturated bond energy introduced by that of the surface material hanging bond, scientists have developed the concept of “surface energy”. Because of this, it has the potential to alter cellular function. For instance, the serum protein adsorption and cell adhesion that occurs upon contact between the polymer material surface and a biological fluid are both energy-dependent. The adherence and spreading of cells are promoted by surfaces having high free energy, whereas the opposite is true for surfaces with low free energy. Plasma treatment is also one of the techniques being widely used for altering the energy level of polymer surfaces (CG et al., 2006; Yongabi et al., 2020). Cell adhesion can also be affected by the polymers’ charge properties of adhesion surfaces. A substantial body of research shows that cells are more likely to cling to positively charged materials. Subtle alterations in cell activity can be induced by surface charge via the chemical functional groups of polymeric material (Terada et al., 2012). According to research published by Abarrategi et al. (2010), surfaces with varying charges (−CH3, −OH, −COOH, and −NH2 groups) controlled FN adsorption as well as integrin direct binding, along with OH > COOHNH2 > CH3 being the preferred pattern for MC3T3 osteoblast adherence to FN-coated surfaces. Figure 4 shows the mechanism of cell attachment to the biomaterial surface.
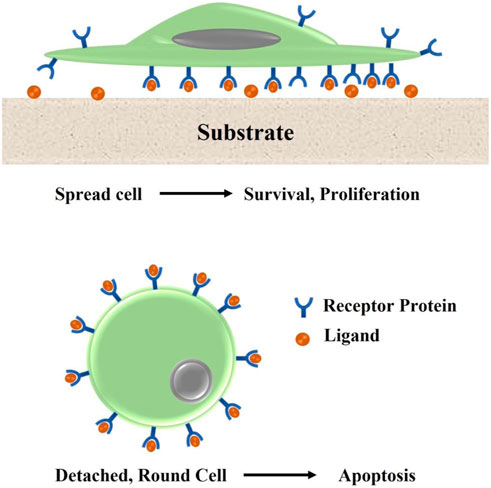
FIGURE 4. Immobilized ligand molecules that function as agonists of the ECM modify the surface. Non-immobilized ligands prevent cells from adhering to the scaffold surface, which causes apoptosis.
7.3 Techniques for surface modification for improved cell adhesion
Several techniques for modifying cellular adhesion surfaces have been developed in an attempt to better comprehend the process by which cell-surface interacts, as illustrated in Table 1. While Figure 5 illustrates the surface modification methods involved in creating cell adhesion surfaces over the biomaterial surface. Figure 6 shows the various crosslinkers including natural and synthetic ones for bone tissue scaffolds. Table 4 shows various strategies for the development of cell adhesive surfaces.
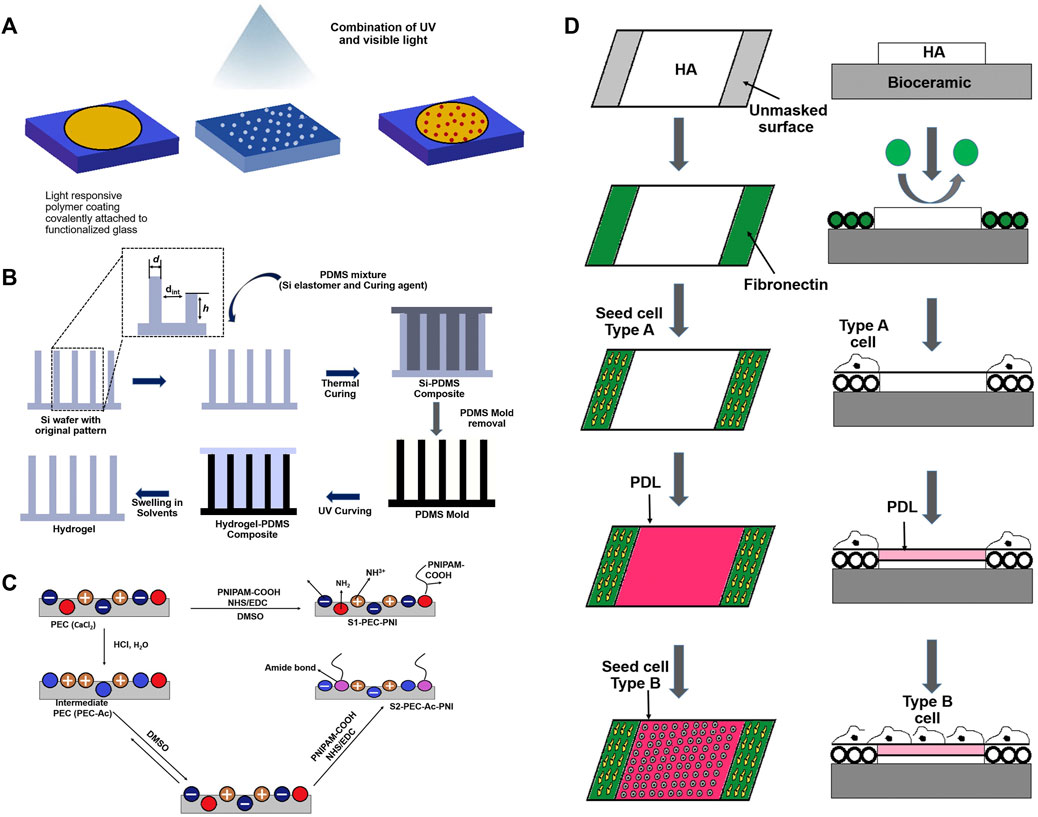
FIGURE 5. Different surface modification techniques of scaffolds for cell adhesion and proliferation: (A) Mask illumination; (B); Soft lithography; (C) EDC-NHS Coupling; (D) Deposition of HA-PLL.
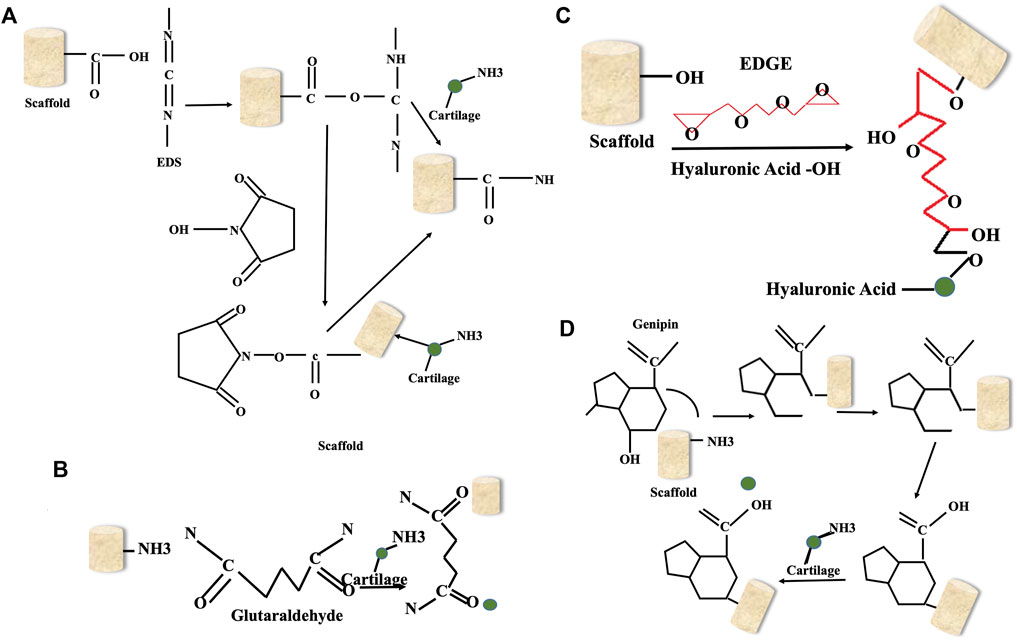
FIGURE 6. Different crosslinkers for bone tissue scaffolds: (A) EDC-NHS; (B); Glutaraldehyde; (C) EDGE; (D) Genipin.
8 Cellular adhesion materials
Every material has its own property, and it causes different reactions in cells. The biocompatibility of materials is the most significant quality on the surface of cell culture. For example, Silicon is widely applied in bone tissue engineering due to its excellent cell adhesion and biological activity. But since silicon is also very unstable, metallic materials have been applied that offer higher strength, hardness, resistance to fatigue, and simplicity in processing and formation of stents in interventional treatments of bone. Likewise, Bioceramics are a class of materials offering low toxicity and biocompatibility. Therefore they are used in dental restoration, artificial hips, other bones, tooth roots, joints, bolts, etc. However, bioceramics possess biocompatible nature yet have a lower toughness value, leading to their application in combination with polymers with biodegradable and biocompatible nature for repairing and transplanting organs. These materials have exceptional and remarkable abilities in the field of tissue engineering; therefore, they are widely used by researchers and clinicians for bone tissue regenerative applications. Table 5 shows the different methods for modification of the scaffold’s surface.
9 Tests applied to “composite matrix and multifunctional polymeric scaffolds” for the determination of bioactivity
Bioactivity is the degree to which a material can influence its biological environment. In recent years, researchers have begun to explore the potential of 3D scaffolds for tissue regeneration. They have developed a number of applications for these scaffolds, with researchers hypothesising that scaffolds could potentially provide structural stability and an environment for cellular regeneration, mimicking the functionality of natural tissue. Since then, 3D scaffolds have been tested for a variety of applications, including bone regeneration, nerve regeneration, muscle regeneration, tendon and ligament regeneration and many others. In order to determine the bioactivity of such multifunctional 3D scaffolds and composites in-vitro bioactivity and in-vivo bioactivity of the scaffolds are assessed.
Presently only two popular techniques are being utilised to evaluate the in-vitro bioactivity of bioceramics. One approach is to assess the bioceramics’ capacity to generate apatite in the simulated bodily fluids (Kokubo and Takadama, 2006). This can be performed via X-Ray diffraction method and using the ICP-OES technique (Pham Minh et al., 2013). Investigating how in-vitro bone cells react to bioceramics is the second alternative method for in-vitro bioactivity assessment (Valerio et al., 2004; Sun et al., 2006; Wu et al., 2008). Singh and colleagues developed a scaffold to look at the apatite formation when placed inside SBF in order to assess the apatite formation (Singh et al., 2020b). Prior to doing in-vivo bone bioactivity investigations, this technique is helpful and can greatly minimise the number of animals required for in-vivo evaluation. The scientific world as a whole has accepted the concept that in-vitro cell testing can be used to examine the in-vitro bioactivity of bioceramics. This method is known as the cell experiment method (Wu and Xiao, 2009b). This technique is frequently used to evaluate the bioactivity of bioceramics. Numerous instances, however, suggest that employing cell tests to assess the in-vitro bioactivity of bioceramics is insufficient (Webster et al., 1999; Manicone et al., 2007a; Manicone et al., 2007b; Popat et al., 2007). Therefore in-vivo bioactivity of the biomaterials is determined within the animal model. Three different steps are adopted for the determination of in-vivo bioactivity which includes blood sample collection for further biochemical analysis after third and sixth week. Followed by histological preparation in which the newly developed bone is isolated surgically and hematoxylin and eosin staining are performed for examination under a light microscope. Lastly, the biochemical analysis of the isolated serum is performed to determine the total alkaline phosphatase activity and test the other biomarkers like osteocalcin (Al-Rashidy et al., 2020). All the above tests are widely applied by researchers in the field of bone tissue engineering to determine the bioactivity of developed biopolymeric scaffolds.
10 Multifunctionality of polymeric scaffolds
Fabrication of biomimetic biomaterials with high porosity and specific morphology is of great interest to the scientific community as it can lead to improved properties. The biomimetic approach is a novel concept in materials science that can tailor material properties such as mechanical strength and stiffness while maintaining beneficial biological functions. Functionalisation of polymeric scaffolds is a common method to make them more suitable for cell growth. While there are many different techniques, most functionalisation methods depend on the material being used and some of these techniques are more effective than others. For example, surface modification method that is widely applied to incorporate the molecules necessary for cellular proliferation. It is done using various polymeric materials to form the desirable structural 3D scaffold. Copolymerisation with the same monomers has been used to functionalise polymer scaffolds in terms of promoting cellular adhesion in order to overcome the parent polymer’s limitations. Fabrication technique also plays an essential role in the functionality of the scaffolds like 3D printing, electrospinning, blowspinning, etc. They help in the development of unique morphologies of microparticles and hydrogels that can possess different mechanical properties, degradation rates and cellular adhesion. Relatively newer techniques for making 3D scaffolds include decellularization and 3D printing methods. Decellularization is a method of producing and functionalising natural 3D scaffolds by extracting an organ from an animal, removing all cells using detergents, and then reimplanting stem cells from a potential host organ. Growth factors can be added to the decellularized scaffold to accelerate cellular differentiation. More recently, bioactive scaffolds have been developed for tissue regeneration through the use of 3D printing. Several organs have been revitalised and cellularized using donor stem cells. A well-known example of this process is a re-cellularized functional heart organ, exhibiting the successful restoration of functionality (Taylor et al., 2020). In addition to the heart, several other organs, such as the lungs (O’Neill et al., 2013) and bladder (Moreno-Manzano et al., 2020), have also been recellularized in-vitro. Among these techniques, however, 3D printing has the advantage of enabling precise scaffold dimensions on the nanoscale compared to other traditional manufacturing methods. Countless bioactive materials have been 3D printed into scaffolds over the years. Even hydrogels have been 3D printed to create specialised 3D scaffolds. In this context, biofunctional polymeric materials with significant mechanical strength can serve as a support matrix for cell proliferation, adhesion and osteogenic differentiation with embedded materials for bone tissue regeneration. Table 6 discusses the recent research on the development of multifunctional polymeric scaffolds along with their applications.
11 Doping and its advantages
Biomedical sciences (medicine and biology) and materials science have collaborated in recent years to create biomaterials for application in tissue reconstruction and replacement. For this phenomenon, biomaterials need to be physically and chemically stable and possess certain mechanical qualities. Various bio-ceramic materials and BGs are among the most commonly employed materials for bone tissue healing and prosthesis concealing due to their biocompatibility, biodegradability, bioactivity, and osteoconductivity (Cai et al., 2019). nBGs are some of the most thoroughly researched nanomaterials for hard tissue reconstruction and replacement (Philippart et al., 2017). These include calcium phosphates (e.g., hydroxyapatite, tricalcium phosphate and calcium silicates e.g., wollastonite (CaSiO3) and larnite (-Ca2O4). Bone-bonding materials can establish stable chemical contact with the surrounding structures by generating apatite, similar to bone, on the surface. Apatite production in-vitro, when exposed to an aqueous media with a chemical composition that mimics physiological liquids, can be used as a predictor of apatite formation in-vivo. The bioceramic materials and nBGs of current generations have indeed been developed because of their capacity to induce bone regeneration and resemble biological tissue, eliciting a response from the body similar to that seen in the presence of natural tissue. Materials that can both anchor the implant in place and attract the cells that regulate their breakdown rate have become increasingly important. Bone-forming capability can be achieved by manipulating the release of certain ions during dissolution in-vivo. Because of the elevated solubilization rate, the released ions are swayed from the transplantation site by body fluid, which may interrupt the bone tissue healing process during new bone ingrowth. Also, the degradation kinetic growth factors presented by calcium phosphate ceramics are typically unsatisfactory, and the materials lack intrinsic osteoinductivity. The low mechanical strength and fracture toughness of BGs also makes it challenging to implant them in weight-bearing areas (Baino, 2018). Figure 7 displays the various doping techniques like EDC-NHS coupling, layer-by-layer assembly, plasma treatment, etc.
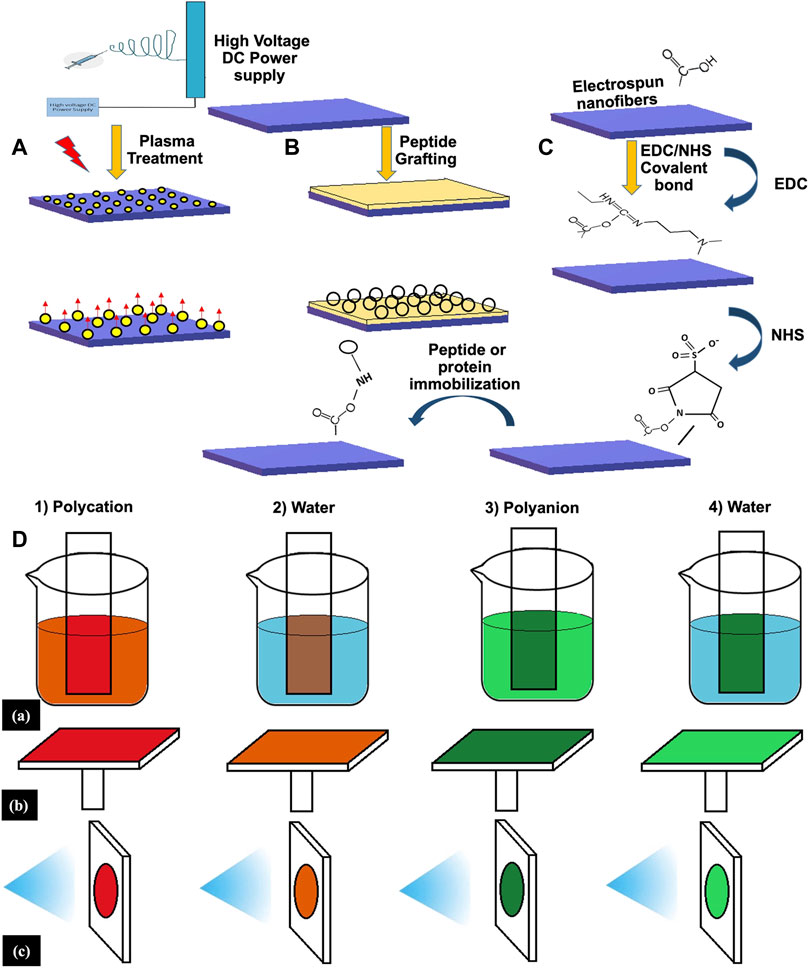
FIGURE 7. Different doping techniques of scaffold surface: (A) Plasma treatment; (B); Peptide Grafting; (C) EDC-NHS Coupling; (D) Layer-by-layer assembly.
The addition of a trace amount of another element into the bulk material is known as doping. It has been found that an increase in the concentration of dopant ions plays a crucial role in facilitating the formation of new crystalline phases. This means that the conditions in which the thermal treatment is carried out can affect pristine material’s qualities (Vahabzadeh and Bose, 2017; Sikder et al., 2020). Bioceramics and BGs are now integrated with certain metal ions that possess therapeutic benefits like Sr2+, Cu2+, and Zn2+ into their chemical structure to mitigate the drawbacks of virgin materials and promote their utilization in a variety of biomedical settings. Past research has shown that minerals, including cations Mg2+, Cu2+, Zn2+, Sr2+, Fe3+/Fe2+, Co2+/3+, and Mn2+, are also essential for bone growth, development, and maintenance. Therefore incorporating them into biomaterials has been shown to increase angiogenesis and osteogenesis (Ryan et al., 2019; Yuan et al., 2019; Zhuang et al., 2019). Considering the importance of cations in governing metabolic pathways, doping with Ag+ and Cu2+ in the structure of bioceramics and nBGs has been extensively researched and shielded against microbial infections in a wide range of biological contexts (El-Rashidy et al., 2018; Predoi et al., 2019; Ryan et al., 2019; Singh et al., 2020c; Hui et al., 2020; Sikder et al., 2020). Some researchers have taken a different track, looking at these materials as drug delivery systems. Targeted nano-carriers or scaffolding components, such as doped-bioceramics, are successfully functionalized with the drug or bioactive molecules to achieve this goal (Morsi and Abd Elhamid, 2019; Singh et al., 2020c).
Researchers investigated the use of rare Earth metals as dopants in bioceramics and nBGs, resulting in the creation of a novel good potential class of luminescent optical nanostructured materials. These materials could substitute organic fluorophores and quantum dots in bio-imaging application fields that range from cancer diagnosis to intra-operative supervision and postsurgical assessment (Neacsu et al., 2019). However, some challenges must be overcome before suitable biocompatibility and biodegradability nanoparticle-imaging sensors can be employed in healthcare situations. Different crystallinity, shape, and stoichiometry in the end products of metal-doped bioceramics and BGs have been achieved by the use of several synthesis techniques (Srinivasan et al., 2019). Methods such as sol-gel (El-Rashidy et al., 2018; Predoi et al., 2019; Zhuang et al., 2019), chemical precipitation (Luo et al., 2018; Gallo et al., 2019; Srinivasan et al., 2019; Chen et al., 2022), the melt-quenching approach (Deng et al., 2019), magnetron sputtering (Vranceanu et al., 2019), and pulsed laser deposition (Duta et al., 2019) are only a few of the many options. Once bioceramics and BGs have proven to be acceptable as coating materials (Predoi et al., 2019; Vranceanu et al., 2019), cements (Li et al., 2018; Zhang and Williams, 2019), scaffolds (Zhao et al., 2014; Yin et al., 2018; Luo et al., 2019; Yuan et al., 2019; Zhuang et al., 2019), and nanoparticles (El-Rashidy et al., 2018; Chen et al., 2019; Morsi and Abd Elhamid, 2019; Singh et al., 2020c), the optimum synthesis process will depend on the application. Over the past decade, researchers have analyzed the literature to determine the effects of metal ions in various inorganic matrices for application in a wide variety of diseases. About metal ion doping of inorganic matrices, they describe, summarise, and evaluate. Several studies using ion-doped inorganic matrices for bone tissue engineering have risen in the past years, which shows the growing interest in these materials among scientists. As a result, silicon, sodium, and phosphorus, the primary chemical components of silicate-based BGs and bioceramics, have attracted a major group of scientists and clinicians. Physicochemical, structural, and biological behaviour within in-vitro and in-vivo environment are discussed in this article showing the effectiveness of ions like magnesium, silver, copper, strontium, lithium, and cobalt. Table 7 discusses the latest research done on the doping of materials and their application in BTE.
12 Future perspective
There have been significant efforts to replace, image, or regenerate bone using cutting-edge nanotechnology as a consequence of a developing understanding of how bone tissues’ nanoscale characteristics contribute to their distinctive capabilities. Novel discoveries in the understanding and engineering of bone tissue are the consequence of these efforts. Several challenges still exist, though.
Creating vascular and innervated bone tissue is among the most challenging problems that current nanofabrication techniques have yet to solve. Nanoparticles and their technologies must be integrated with upgraded materials and processes. Manufacturing methods like multi-material bioprinting should be used in order to imitate the multiple-scale “organized chaos” found in bone tissues, including the cellular hierarchy and the ECM. Such pairings run into difficulties because of the mechanical differences between hydrogels, which are often used in bioprinting, and relatively hard materials ideal for bone ECM modelling.
It is predicted that the development of innovative bioinks and high-resolution printing methods would significantly impact future breakthroughs in hierarchical osseous tissue bioprinting. The advent of medicine will increasingly focus on the patient and be more customized; it is already commonly accepted. To employ powerful algorithms to influence advertising and subsequent purchase decisions, online firms already collect large volumes of data about consumer populations; nevertheless, assessments of medical treatment are based on surprisingly little data. Combining breakthroughs in nanotechnology with fields like big data research, genomics, and proteomics, among others, may have unanticipated implications for the TE sector.
In immunology, for instance, a patient’s particular biology cannot be avoided, yet illnesses, not individuals, are the focus of small molecule medication development. An initial move toward individualized care is the utilization of patient-explicit cells for bone TE, like MSCs and, at this point, unstudied iPSCs. Bone TE strategies will get redone as additional patient information is received and dissected at lower costs.
It may also be necessary to use pico-technology or engineering at sizes smaller than 10−9 m by changing the electrical environment of atoms and molecules to figure out how to arrange collagen and mineral phases to replicate the intricate natural structure of bone. Recent evidence suggests that the shape and handedness of calcium carbonate crystals may be changed by the addition of chiral amino acids, much like the helical forms that naturally occur in bone.
The insertion of small molecules with varying electron distributions is an early form of constructing nanostructures from “below.” The ability to customize medications and use pico-technology will undoubtedly improve the adequacy of nanomedicine and the likely harmfulness of nanomaterials. For example, NPs’ organic personality and potential as medication conveyance stages will be emphatically affected by the normally happening protein crown that encompasses them. NPs that advance the adsorption of supplement proteins will be obliterated before arriving at the designated tissues, as opposed to NPs that have some level of secrecy abilities in view of their surface properties, like electron dispersion.
The most recent finding that is; patient and illness type-dependent variations in NP corona composition exist emphasizes the complexity that nanomedicine researchers are being forced to accept more and more. The availability of additional patient-specific data and a greater comprehension of NP properties at the smallest scales will influence future nanomedicine initiatives. Finally, it has been demonstrated that decellularized bone matrices are more appealing than bottom-up scaffold design. This is because, despite their best efforts, TE scaffolds for bone have yet to imitate the unique physical properties of bone that derive from its multiscale architecture.
It will be important to acquire a more profound comprehension of the scattering of nanoparticles in a solid stage, and the interfacial connections between the two gradually ease that leading to drive transmission to make tissues that can imitate the innate durability and strength of bone from the base (nanoscale) up, potentially with the guide of pico-technology, refined virtual experiences, and information science.
Enhanced assessment procedures are needed to verify the suitability and effectiveness of nanoengineered systems for bone engineering. The possibility of therapies working in-vivo will undoubtedly rise with more accurate testing under in-vivo settings, including interstitial flow, various cell types organized in three dimensions, and mixtures of bodily fluids, including growth factors and serum proteins.
Additive manufacturing based techniques, notably three-dimensional printing and bioprinting, demonstrated tremendous promise in applications involving tissue engineering owing to their ability to customise (including both temporal and spatial control) in the fabrication of grafts or structures made from tissue that is specific to the patient. By leveraging tissue-specific printed structures made with a range of AM-based technologies, both in-vivo and in-vitro research has made major advancements in the regeneration of hard tissues.
Despite several research initiatives to improve the desirable properties of printable structures made of HAp-based substances, the conception, development, and treatment of HAp-based bioinks to obtain desired properties, notably for implants that bear load, in accordance with a particularly tough type of tissue and/or organ to achieve desirable qualities in the native hard tissue environment remains a substantial obstacle. Proper bioink design and development are necessary to enable tissue regeneration resembling natural tissue with bioprinted implants (i.e., implant integration, progressive remodelling, development and vascularization). This entails considering concerns linked to printing in terms of the components, cells, processing configurations (pre/post printing), and so forth.
This necessitates a deep grasp of biomaterials, cells, techniques of printing, and the in-vivo biological milieu. Throughout the printing process, additional significant obstacles include the possibility of nozzle obstruction and the creation of mechanically stable designs. In addition, printing more advanced 3D designs that make use of a variety of materials, cell types, and printing procedures to produce cellular diversity and functionality in-vivo is a significant challenge.
In the field of tissue engineering, it is also extremely difficult to bring 3D-printed biomaterials into the clinic. To investigate structural, chemical, physicomechanical, rheological, biological, and immunological issues using a multidisciplinary approach, significant and crucial tissue engineering research efforts are required.
13 Conclusion
This review illustrates the interface research on the physiology of bone and the various bone repair strategies for restoring and replacing damaged or defective bone. All the results that have been investigated in recent studies suggested useful information for creating new biomaterial-based products. However, it is imperative to look at the outcomes of each research trial. Bone graft replacements, implantable materials and scaffolds, tailored 3D structures, and preferred surface qualities are only a few of the several bone restoration techniques. These techniques are inclusive of the factors involved in the development of such biomaterials that can restore and repair damaged bone tissue. Like surface properties of the scaffold material that has a major role in cell adhesion and tissue formation. This article gives insight into how surface modification can enhance bone tissue regeneration. Similarly, pore size and pore morphology which have an adequate response towards cell adhesion and proliferation can also be modified by altering the material choice and fabrication method. It has been observed in past studies that as the size of the bone lesions increases, the role of the bioactive components enhances. Therefore these days, at the pre-clinical stage, signalling factors, polypeptides, and small biomolecules are evaluated for this purpose. By combining these bioactive compounds with cutting-edge carriers, it may be possible to build a delivery system (scaffold) that delivers the active molecules in a consistent and beneficial quantity. However, cell-based methods can also be used to treat significant and complex bone abnormalities, more controlling phases are necessary for future advancements in order to ensure effective clinical treatment.
Author contributions
PS, SK, AM, and BS conceived and outlined the review. All authors including wrote and edited the manuscript and designed the schematics.
Funding
This research was funded by MHRD, SPARC, IUSSTF and SERB-GOI.
Acknowledgments
Authors would like to thank IIT BHU, Varanasi for providing infrastructure support for the completion of the studies. Authors would like to thank MHRD, the Government of India for providing research support through SPARC funding (SPARC/2018-19/28/SL(IN)). Authors would also like to thank SERB, GOI for providing support through SRG funding (SRG/2021/001686).
Conflict of interest
The authors declare that the research was conducted in the absence of any commercial or financial relationships that could be construed as a potential conflict of interest.
Publisher’s note
All claims expressed in this article are solely those of the authors and do not necessarily represent those of their affiliated organizations, or those of the publisher, the editors and the reviewers. Any product that may be evaluated in this article, or claim that may be made by its manufacturer, is not guaranteed or endorsed by the publisher.
References
Abarrategi, A., Lópiz-Morales, Y., Ramos, V., Civantos, A., López-Durán, L., Marco, F., et al. (2010). Chitosan scaffolds for osteochondral tissue regeneration. J. Biomed. Mat. Res. A 95 (4), 1132–1141. doi:10.1002/jbm.a.32912
Abhinandan, R., Adithya, S. P., Sidharthan, D. S., Balagangadharan, K., and Selvamurugan, N. (2021). Synthesis and characterization of magnesium diboride nanosheets in alginate/polyvinyl alcohol scaffolds for bone tissue engineering. Colloids Surfaces B Biointerfaces 203, 111771. doi:10.1016/j.colsurfb.2021.111771
al Kayal, T., Losi, P., Pierozzi, S., and Soldani, G. (2020). A new method for fibrin-based electrospun/sprayed scaffold fabrication. Sci. Rep. 10 (1), 1–4.doi:10.1038/s41598-020-61933-z
Al-Rashidy, Z. M., Omar, A. E., El-Aziz, T. H. A., and Farag, M. M. (2020). In vivo bioactivity assessment of strontium-containing soda-lime-borate glass implanted in femoral defect of rat. J. Inorg. Organomet. Polym. Mat. 30 (10), 3953–3964. doi:10.1007/s10904-020-01535-4
Alicka, M., Sobierajska, P., Kornicka, K., Wiglusz, R. J., and Marycz, K. (2019). Lithium ions (Li+) and nanohydroxyapatite (nHAp) doped with Li+ enhance expression of late osteogenic markers in adipose-derived stem cells. Potential theranostic application of nHAp doped with Li+ and co-doped with europium (III) and samarium (III) ions. Mater. Sci. Eng. C 99, 1257–1273. doi:10.1016/j.msec.2019.02.073
Alvarez Echazú, M., Renou, S., Alvarez, G., Desimone, M., and Olmedo, D. (2022). A collagen-silica-based biocomposite for potential application in bone tissue engineering. J. Biomed. Mat. Res. A 110 (2), 331–340. doi:10.1002/jbm.a.37291
Amani, H., Kazerooni, H., Hassanpoor, H., Akbarzadeh, A., and Pazoki-Toroudi, H. (2019). Tailoring synthetic polymeric biomaterials towards nerve tissue engineering: A review. Artif. Cells, Nanomedicine, Biotechnol. 47 (1), 3524–3539. doi:10.1080/21691401.2019.1639723
Amini, A. R., Laurencin, C. T., and Nukavarapu, S. P. (2012). Bone tissue engineering: Recent advances and challenges. Crit. Rev. Biomed. Eng. 40 (5), 363–408. doi:10.1615/critrevbiomedeng.v40.i5.10
Amornkitbamrung, U., Hong, M.-H., and Shin, H. (2020). On the crystallization of hydroxyapatite under hydrothermal conditions: Role of sebacic acid as an additive. ACS Omega 5 (42), 27204–27210. doi:10.1021/acsomega.0c03297
Arif, Z. U., Khalid, M. Y., Sheikh, M. F., Zolfagharian, A., and Bodaghi, M. (2022). Biopolymeric sustainable materials and their emerging applications. J. Environ. Chem. Eng. 10, 108159. doi:10.1016/j.jece.2022.108159
Babilotte, J., Martin, B., Guduric, V., Bareille, R., Agniel, R., Roques, S., et al. (2021). Development and characterization of a PLGA-HA composite material to fabricate 3D-printed scaffolds for bone tissue engineering. Mater. Sci. Eng. C 118, 111334. doi:10.1016/j.msec.2020.111334
Bai, J., Wang, H., Gao, W., Liang, F., Wang, Z., Zhou, Y., et al. (2020). Melt electrohydrodynamic 3D printed poly (ε-caprolactone)/polyethylene glycol/roxithromycin scaffold as a potential anti-infective implant in bone repair. Int. J. Pharm. 576, 118941. doi:10.1016/j.ijpharm.2019.118941
Baino, F. (2018). Bioactive glasses–when glass science and technology meet regenerative medicine. Ceram. Int. 44 (13), 14953–14966. doi:10.1016/j.ceramint.2018.05.180
Baker, M. I., Walsh, S. P., Schwartz, Z., and Boyan, B. D. (2012). A review of polyvinyl alcohol and its uses in cartilage and orthopedic applications. J. Biomed. Mat. Res. 100 (5), 1451–1457. doi:10.1002/jbm.b.32694
Baptista, R., and Guedes, M. (2021). Morphological and mechanical characterization of 3D printed PLA scaffolds with controlled porosity for trabecular bone tissue replacement. Mater. Sci. Eng. C 118, 111528. doi:10.1016/j.msec.2020.111528
Basha, R. Y., Ts, S. K., and Doble, M. (2015). Design of biocomposite materials for bone tissue regeneration. Mater. Sci. Eng. C 57, 452–463. doi:10.1016/j.msec.2015.07.016
Bayraktar, A., Saraçoğlu, B., Gölgelioğlu, Ç., and Tuncel, A. (2012). Click-chemistry for surface modification of monodisperse-macroporous particles. J. Colloid Interface Sci. 365 (1), 63–71. doi:10.1016/j.jcis.2011.08.071
Berendsen, A. D., and Olsen, B. R. (2015). Bone development. Bone Dev. Bone 80, 14–18. doi:10.1016/j.bone.2015.04.035
Bishop, J. A., Palanca, A. A., Bellino, M. J., and Lowenberg, D. W. (2012). Assessment of compromised fracture healing. J. Am. Acad. Orthop. Surg. 20 (5), 273–282. doi:10.5435/jaaos-20-05-273
Biswal, T. (2021). Biopolymers for tissue engineering applications: A review. Mater. Today Proc. 41, 397–402. doi:10.1016/j.matpr.2020.09.628
Bonnans, C., Chou, J., and Werb, Z. (2014). Remodelling the extracellular matrix in development and disease. Nat. Rev. Mol. Cell Biol. 15 (12), 786–801. doi:10.1038/nrm3904
Budak, K., Sogut, O., and Aydemir Sezer, U. (2020). A review on synthesis and biomedical applications of polyglycolic acid. J. Polym. Res. 27 (8), 208–219. doi:10.1007/s10965-020-02187-1
Cai, Z., Wan, Y., Becker, M. L., Long, Y.-Z., and Dean, D. (2019). Poly (propylene fumarate)-based materials: Synthesis, functionalization, properties, device fabrication and biomedical applications. Biomaterials 208, 45–71. doi:10.1016/j.biomaterials.2019.03.038
Castillo-Dalí, G., Velázquez-Cayón, R., Serrera-Figallo, M. A., Rodríguez-González-Elipe, A., Gutierrez-Pérez, J.-L., and Torres-Lagares, D. (2015). Importance of poly (lactic-co-glycolic acid) in scaffolds for guided bone regeneration: A focused review. J. Oral Implant. 41 (4), e152–e157. doi:10.1563/aaid-joi-d-13-00225
Cg, K. S. B. W. N. R. S., Washburn, N., Simonjr, C., and Amis, E. (2006). Combinatorial screen of the effect of surface energy on fibronectin-mediated osteoblast adhesion, spreading and proliferation☆. Biomaterials 27, 3817–3824. doi:10.1016/j.biomaterials.2006.02.044
Chandran, L., and Am, B. (2021). Apatite matrix substituted with biologically essential rare Earth elements as an artificial hard tissue substitute: Systematic physicochemical and biological evaluation. J. Biomed. Mat. Res. A 109 (6), 821–828. doi:10.1002/jbm.a.37069
Chen, J., Wang, H., Wu, Y., Liu, Y., Shi, Y., Chen, C., et al. (2022). Biocompatible octacalcium phosphate/sodium alginate/silk fibroin composite scaffolds for bone regeneration. Mater. Today Commun. 31, 103312. doi:10.1016/j.mtcomm.2022.103312
Chen, S., Shi, Y., Luo, Y., and Ma, J. (2019). Layer-by-layer coated porous 3D printed hydroxyapatite composite scaffolds for controlled drug delivery. Colloids Surfaces B Biointerfaces 179, 121–127. doi:10.1016/j.colsurfb.2019.03.063
Chocholata, P., Kulda, V., and Babuska, V. (2019). Fabrication of scaffolds for bone-tissue regeneration. Materials 12 (4), 568. doi:10.3390/ma12040568
Christy, P. N., Basha, S. K., and Kumari, V. S. (2022). Nano zinc oxide and nano bioactive glass reinforced chitosan/poly (vinyl alcohol) scaffolds for bone tissue engineering application. Mater. Today Commun. 31, 103429. doi:10.1016/j.mtcomm.2022.103429
Chu, P. K., Chen, J. Y., Wang, L. P., and Huang, N. (2002). Plasma-surface modification of biomaterials. Mater. Sci. Eng. R Rep. 36 (5–6), 143–206. doi:10.1016/s0927-796x(02)00004-9
Cm, M., and O'Brien, F. J. (2010). The effect of mean pore size on cell attachment, proliferation and migration in collagen–glycosaminoglycan scaffolds for bone tissue engineering. Biomaterials 31, 461–466. doi:10.1016/j.biomaterials.2009.09.063
Cooke, M. E., Ramirez-GarciaLuna, J. L., Rangel-Berridi, K., Park, H., Nazhat, S. N., Weber, M. H., et al. (2020). 3D printed polyurethane scaffolds for the repair of bone defects. Front. Bioeng. Biotechnol. 8, 557215. doi:10.3389/fbioe.2020.557215
Coulson-Thomas, Y. M., Coulson-Thomas, V. J., Norton, A. L., Gesteira, T. F., Cavalheiro, R. P., Meneghetti, M. C. Z., et al. (2015). The identification of proteoglycans and glycosaminoglycans in archaeological human bones and teeth. PLoS One 10 (6), e0131105. doi:10.1371/journal.pone.0131105
de Souza, R. F. B., and Moraes, Â. M. (2022). Hybrid bilayered chitosan-xanthan/PCL scaffolds as artificial periosteum substitutes for bone tissue regeneration.J Mater Sci 57, 2924–2940. doi:10.1007/s10853-021-06800-6
Delpino, G. P., Borges, R., Zambanini, T., Joca, J. F. S., Gaubeur, I., de Souza, A. C. S., et al. (2021). Sol-gel-derived 58S bioactive glass containing holmium aiming brachytherapy applications: A dissolution, bioactivity, and cytotoxicity study. Mater. Sci. Eng. C 119, 111595. doi:10.1016/j.msec.2020.111595
Deng, Z., Lin, B., Jiang, Z., Huang, W., Li, J., Zeng, X., et al. (2019). Hypoxia-mimicking cobalt-doped borosilicate bioactive glass scaffolds with enhanced angiogenic and osteogenic capacity for bone regeneration. Int. J. Biol. Sci. 15 (6), 1113–1124. doi:10.7150/ijbs.32358
Ding, Y., Hao, Y., Yuan, Z., Tao, B., Chen, M., Lin, C., et al. (2020). A dual-functional implant with an enzyme-responsive effect for bacterial infection therapy and tissue regeneration. Biomater. Sci. 8 (7), 1840–1854. doi:10.1039/c9bm01924c
Do, A., Khorsand, B., Geary, S. M., and Salem, A. K. (2015). 3D printing of scaffolds for tissue regeneration applications. Adv. Healthc. Mat. 4 (12), 1742–1762. doi:10.1002/adhm.201500168
Donnaloja, F., Jacchetti, E., Soncini, M., and Raimondi, M. T. (2020). Natural and synthetic polymers for bone scaffolds optimization. Polymers 12 (4), 905. doi:10.3390/polym12040905
Du, J., Gan, S., Bian, Q., Fu, D., Wei, Y., Wang, K., et al. (2018). Preparation and characterization of porous hydroxyapatite/β-cyclodextrin-based polyurethane composite scaffolds for bone tissue engineering. J. Biomater. Appl. 33 (3), 402–409. doi:10.1177/0885328218797545
Duan, K., and Wang, R. (2006). Surface modifications of bone implants through wet chemistry. J. Mat. Chem. 16 (24), 2309–2321. doi:10.1039/b517634d
Dubok, V. A. (2000). Bioceramics―yesterday, today, tomorrow. Powder Metallurgy Metal Ceram. 39 (7), 381–394. doi:10.1023/a:1026617607548
Duta, L., Chifiriuc, M. C., Popescu-Pelin, G., Bleotu, C., Gradisteanu, G., Anastasescu, M., et al. (2019). Pulsed laser deposited biocompatible lithium-doped hydroxyapatite coatings with antimicrobial activity. Coatings 9 (1), 54. doi:10.3390/coatings9010054
Dwivedi, R., Kumar, S., Pandey, R., Mahajan, A., Nandana, D., Katti, D. S., et al. (2020). Polycaprolactone as biomaterial for bone scaffolds: Review of literature. J. Oral Biol. Craniofacial Res. 10 (1), 381–388. doi:10.1016/j.jobcr.2019.10.003
Einhorn, T. A. (2005). The science of fracture healing. J. Orthop. Trauma 19 (10), S4–S6. doi:10.1097/00005131-200511101-00002
El-Rashidy, A. A., Waly, G., Gad, A., Hashem, A. A., Balasubramanian, P., Kaya, S., et al. (2018). Preparation and in vitro characterization of silver-doped bioactive glass nanoparticles fabricated using a sol-gel process and modified Stöber method. J. Non-Crystalline Solids 483, 26–36. doi:10.1016/j.jnoncrysol.2017.12.044
Ensoylu, M., Deliormanlı, A. M., and Atmaca, H. (2021). Tungsten disulfide nanoparticle-containing PCL and PLGA-coated bioactive glass composite scaffolds for bone tissue engineering applications. J. Mat. Sci. 56 (33), 18650–18667. doi:10.1007/s10853-021-06494-w
Fabbri, P., and Messori, M. (2017). “Surface modification of polymers: Chemical, physical, and biological routes,” in Modification of polymer properties (Elsevier), 109–130.Amsterdam, Netherlands
Fang, J., Li, P., Lu, X., Fang, L., Lü, X., and Ren, F. (2019). A strong, tough, and osteoconductive hydroxyapatite mineralized polyacrylamide/dextran hydrogel for bone tissue regeneration. Acta Biomater. 88, 503–513. doi:10.1016/j.actbio.2019.02.019
Farzamfar, S., Naseri-Nosar, M., Sahrapeyma, H., Ehterami, A., Goodarzi, A., Rahmati, M., et al. (2019). Tetracycline hydrochloride-containing poly (ε-caprolactone)/poly lactic acid scaffold for bone tissue engineering application: In vitro and in vivo study. Int. J. Polym. Mater. Polym. Biomaterials 68 (8), 472–479. doi:10.1080/00914037.2018.1466133
Fernandes, M. M., Correia, D. M., Ribeiro, C., Castro, N., Correia, V., and Lanceros-Mendez, S. (2019). Bioinspired three-dimensional magnetoactive scaffolds for bone tissue engineering. ACS Appl. Mat. Interfaces 11 (48), 45265–45275. doi:10.1021/acsami.9b14001
Filippi, M., Born, G., Chaaban, M., and Scherberich, A. (2020). Natural polymeric scaffolds in bone regeneration. Front. Bioeng. Biotechnol. 8, 474. doi:10.3389/fbioe.2020.00474
Fonseca, H., Moreira-Gonçalves, D., Coriolano, H.-J. A., and Duarte, J. A. (2014). Bone quality: The determinants of bone strength and fragility. Sports Med. 44 (1), 37–53. doi:10.1007/s40279-013-0100-7
Frantz, C., Stewart, K. M., and Weaver, V. M. (2010). The extracellular matrix at a glance. J. Cell Sci. 123 (24), 4195–4200. doi:10.1242/jcs.023820
Frost, H. M. (1989). The biology of fracture healing. An overview for clinicians. Part I. Clin. Orthop. Relat. Res. 248, 283–293. doi:10.1097/00003086-198911000-00045
Fu, Q., Li, Z., Fu, F., Chen, X., Song, J., and Yang, H. (2021). Stimuli-responsive plasmonic assemblies and their biomedical applications. Nano Today 36, 101014. doi:10.1016/j.nantod.2020.101014
Gallo, M., Santoni, B. L. G., Douillard, T., Zhang, F., Gremillard, L., Dolder, S., et al. (2019). Effect of grain orientation and magnesium doping on β-tricalcium phosphate resorption behavior. Acta Biomater. 89, 391–402. doi:10.1016/j.actbio.2019.02.045
Garnero, P. (2015). The role of collagen organization on the properties of bone. Calcif. Tissue Int. 97 (3), 229–240. doi:10.1007/s00223-015-9996-2
Gautam, S., Sharma, C., Purohit, S. D., Singh, H., Dinda, A. K., Potdar, P. D., et al. (2021). Gelatin-polycaprolactone-nanohydroxyapatite electrospun nanocomposite scaffold for bone tissue engineering. Mater. Sci. Eng. C 119, 111588. doi:10.1016/j.msec.2020.111588
Ghassemi, T., Shahroodi, A., Ebrahimzadeh, M. H., Mousavian, A., Movaffagh, J., and Moradi, A. (2018). Current concepts in scaffolding for bone tissue engineering. Arch. Bone Jt. Surg. 6 (2), 90–99.
Gholizadeh, S., Moztarzadeh, F., Haghighipour, N., Ghazizadeh, L., Baghbani, F., Shokrgozar, M. A., et al. (2017). Preparation and characterization of novel functionalized multiwalled carbon nanotubes/chitosan/β-Glycerophosphate scaffolds for bone tissue engineering. Int. J. Biol. Macromol. 97, 365–372. doi:10.1016/j.ijbiomac.2016.12.086
Ghorai, S. K., Roy, T., Maji, S., Ray, P. G., Sarkar, K., Dutta, A., et al. (2022). A judicious approach of exploiting polyurethane-urea based electrospun nanofibrous scaffold for stimulated bone tissue regeneration through functionally nobbled nanohydroxyapatite. Chem. Eng. J. 429, 132179. doi:10.1016/j.cej.2021.132179
Ghorbani, F., Ghalandari, B., Sahranavard, M., Zamanian, A., and Collins, M. N. (2021). Tuning the biomimetic behavior of hybrid scaffolds for bone tissue engineering through surface modifications and drug immobilization. Mater. Sci. Eng. C 130, 112434. doi:10.1016/j.msec.2021.112434
Gilarska, A., Lewandowska-Łańcucka, J., Guzdek-Zając, K., Karewicz, A., Horak, W., Lach, R., et al. (2020). Bioactive yet antimicrobial structurally stable collagen/chitosan/lysine functionalized hyaluronic acid–based injectable hydrogels for potential bone tissue engineering applications. Int. J. Biol. Macromol. 155, 938–950. doi:10.1016/j.ijbiomac.2019.11.052
Goonoo, N., Bhaw-Luximon, A., Bowlin, G. L., and Jhurry, D. (2013). An assessment of biopolymer-and synthetic polymer-based scaffolds for bone and vascular tissue engineering. Polym. Int. 62 (4), 523–533. doi:10.1002/pi.4474
Govindharajulu, J. P., Chen, X., Li, Y., Rodriguez-Cabello, J. C., Battacharya, M., and Aparicio, C. (2017). Chitosan-recombinamer layer-by-layer coatings for multifunctional implants. Int. J. Mol. Sci. 18 (2), 369. doi:10.3390/ijms18020369
Guduric, V., Metz, C., Siadous, R., Bareille, R., Levato, R., Engel, E., et al. (2017). Layer-by-layer bioassembly of cellularized polylactic acid porous membranes for bone tissue engineering. J. Mat. Sci. Mat. Med. 28 (5), 78–11. doi:10.1007/s10856-017-5887-6
Gunatillake, P. A., Adhikari, R., and Gadegaard, N. (2003). Biodegradable synthetic polymers for tissue engineering. Eur. Cell. Mat. 5 (1), 1–16. doi:10.22203/ecm.v005a01
Guo, B., and Ma, P. X. (2018). Conducting polymers for tissue engineering. Biomacromolecules 19 (6), 1764–1782. doi:10.1021/acs.biomac.8b00276
Guo, L., Liang, Z., Yang, L., Du, W., Yu, T., Tang, H., et al. (2021). The role of natural polymers in bone tissue engineering. J. Control. Release 338, 571–582. doi:10.1016/j.jconrel.2021.08.055
Guo, S., Zhu, X., Li, M., Shi, L., Ong, J. L. T., Jańczewski, D., et al. (2016). Parallel control over surface charge and wettability using polyelectrolyte architecture: Effect on protein adsorption and cell adhesion. ACS Appl. Mat. Interfaces 8 (44), 30552–30563. doi:10.1021/acsami.6b09481
Habibovic, P., Gbureck, U., Doillon, C. J., Bassett, D. C., van Blitterswijk, C. A., and Barralet, J. E. (2008). Osteoconduction and osteoinduction of low-temperature 3D printed bioceramic implants. Biomaterials 29 (7), 944–953. doi:10.1016/j.biomaterials.2007.10.023
Han, L., Wang, M., Sun, H., Li, P., Wang, K., Ren, F., et al. (2017). Porous titanium scaffolds with self-assembled micro/nano-hierarchical structure for dual functions of bone regeneration and anti-infection. J. Biomed. Mat. Res. A 105 (12), 3482–3492. doi:10.1002/jbm.a.36178
Hickey, R. J., and Pelling, A. E. (2019). Cellulose biomaterials for tissue engineering. Front. Bioeng. Biotechnol. 7, 45. doi:10.3389/fbioe.2019.00045
Hines, D. J., and Kaplan, D. L. (2013). Poly (lactic-co-glycolic) acid− controlled-release systems: Experimental and modeling insights. Crit. Rev. Ther. Drug Carr. Syst. 30 (3), 257–276. doi:10.1615/critrevtherdrugcarriersyst.2013006475
Hu, M., Xiao, F., Ke, Q.-F., Li, Y., Chen, X.-D., and Guo, Y.-P. (2019). Cerium-doped whitlockite nanohybrid scaffolds promote new bone regeneration via SMAD signaling pathway. Chem. Eng. J. 359, 1–12. doi:10.1016/j.cej.2018.11.116
Hu, Z., Ma, C., Rong, X., Zou, S., and Liu, X. (2018). Immunomodulatory ECM-like microspheres for accelerated bone regeneration in diabetes mellitus. ACS Appl. Mat. Interfaces 10 (3), 2377–2390. doi:10.1021/acsami.7b18458
Hubbe, M. A., Rojas, O. J., and Lucia, L. A. (2015). Green modification of surface characteristics of cellulosic materials at the molecular or nano scale: A review. BioResources 10 (3), 6095–6206. doi:10.15376/biores.10.3.hubbe
Hui, Y., Dong, Z., Wenkun, P., Yao, D., Huichang, G., and Tongxiang, L. (2020). Facile synthesis of copper doping hierarchical hollow porous hydroxyapatite beads by rapid gelling strategy. Mater. Sci. Eng. C 109, 110531. doi:10.1016/j.msec.2019.110531
Idumah, C. I., Nwabanne, J. T., and Tanjung, F. A. (2021). Novel trends in poly (lactic) acid hybrid bionanocomposites. Clean. Mater. 2, 100022. doi:10.1016/j.clema.2021.100022
Iglesias-Mejuto, A., and García-González, C. A. (2021). 3D-printed alginate-hydroxyapatite aerogel scaffolds for bone tissue engineering. Mater. Sci. Eng. C 131, 112525. doi:10.1016/j.msec.2021.112525
Ikeda, J., Zhao, C., Sun, Y.-L., An, K.-N., and Amadio, P. C. (2010). Carbodiimide-derivatized hyaluronic acid surface modification of lyophilized flexor tendon: A biomechanical study in a canine in vitro model. J. Bone Jt. Surg. 92 (2), 388–395. doi:10.2106/jbjs.h.01641
Ikono, R., Li, N., Pratama, N. H., Vibriani, A., Yuniarni, D. R., Luthfansyah, M., et al. (2019). Enhanced bone regeneration capability of chitosan sponge coated with TiO2 nanoparticles. Biotechnol. Rep. 24, e00350. doi:10.1016/j.btre.2019.e00350
Janmohammadi, M., Nazemi, Z., Salehi, A. O. M., Seyfoori, A., John, J. v., Nourbakhsh, M. S., et al. (2023). Cellulose-based composite scaffolds for bone tissue engineering and localized drug delivery. Bioact. Mater. 20, 137–163. doi:10.1016/j.bioactmat.2022.05.018
Jasso-Gastinel, C. F., and Kenny, J. M. (2016). Modification of polymer properties. Italy: University of Perugia.
Jeon, H., Koo, S., Reese, W. M., Loskill, P., Grigoropoulos, C. P., and Healy, K. E. (2015). Directing cell migration and organization via nanocrater-patterned cell-repellent interfaces. Nat. Mat. 14 (9), 918–923. doi:10.1038/nmat4342
Jia, T., Wang, Y., Dou, Y., Li, Y., Jung de Andrade, M., Wang, R., et al. (2019). Moisture sensitive smart yarns and textiles from self-balanced silk fiber muscles. Adv. Funct. Mat. 29 (18), 1808241. doi:10.1002/adfm.201808241
Kalamajski, S., Aspberg, A., Lindblom, K., Heinegård, D., and Oldberg, Å. (2009). Asporin competes with decorin for collagen binding, binds calcium and promotes osteoblast collagen mineralization. Biochem. J. 423 (1), 53–59. doi:10.1042/bj20090542
Kanwar, S., and Vijayavenkataraman, S. (2021). Design of 3D printed scaffolds for bone tissue engineering: A review. Bioprinting 24, e00167. doi:10.1016/j.bprint.2021.e00167
Kao, W.-L., Chang, H.-Y., Lin, K.-Y., Lee, Y.-W., and Shyue, J.-J. (2018). Assessment of the effects of surface potential on the kinetics of HEK293T cell adhesion behavior using a quartz crystal microbalance with dissipation monitoring. J. Phys. Chem. C 122 (1), 694–704. doi:10.1021/acs.jpcc.7b11072
Kareem, M. M., Hodgkinson, T., Sanchez, M. S., Dalby, M. J., and Tanner, K. E. (2019). Hybrid core–shell scaffolds for bone tissue engineering. Biomed. Mat. 14 (2), 025008. doi:10.1088/1748-605x/aafbf1
Karpouzos, A., Diamantis, E., Farmaki, P., Savvanis, S., and Troupis, T. (2017). Nutritional aspects of bone health and fracture healing. J. Osteoporos. 2017, 1–10. doi:10.1155/2017/4218472
Karpov, T. E., Muslimov, A. R., Zyuzin, M. v., Peltek, O. O., Sergeev, I. S., Goncharenko, A. A., et al. (2020). Multifunctional scaffolds based on piezoelectric electrospun fibers modified with biocompatible drug carriers for regenerative medicine. J. Phys. Conf. Ser. 1461 (1), 012060. doi:10.1088/1742-6596/1461/1/012060
Katti, D. S., Vasita, R., and Shanmugam, K. (2008). Improved biomaterials for tissue engineering applications: Surface modification of polymers. Curr. Top. Med. Chem. 8 (4), 341–353. doi:10.2174/156802608783790893
Khan, M. U. A., Al-Arjan, W. S., Ashammakhi, N., Haider, S., Amin, R., and Hasan, A. (2022). Multifunctional bioactive scaffolds from ARX-g-(Zn@ rGO)-HAp for bone tissue engineering: In vitro antibacterial, antitumor, and biocompatibility evaluations. ACS Appl. Bio Mat. doi:10.1021/acsabm.2c00777
Kim, B. N., Ko, Y.-G., Yeo, T., Kim, E. J., Kwon, O. K., and Kwon, O. H. (2019). Guided regeneration of rabbit calvarial defects using silk fibroin nanofiber–poly (glycolic acid) hybrid scaffolds. ACS Biomater. Sci. Eng. 5 (10), 5266–5272. doi:10.1021/acsbiomaterials.9b00678
Kim, D., Thangavelu, M., Cheolui, S., Kim, H. S., Choi, M. J., Song, J. E., et al. (2019). Effect of different concentration of demineralized bone powder with gellan gum porous scaffold for the application of bone tissue regeneration. Int. J. Biol. Macromol. 134, 749–758. doi:10.1016/j.ijbiomac.2019.04.184
Kim, H. D., Lee, E. A., An, Y.-H., Kim, S. L., Lee, S. S., Yu, S. J., et al. (2017). Chondroitin sulfate-based biomineralizing surface hydrogels for bone tissue engineering. ACS Appl. Mat. Interfaces 9 (26), 21639–21650. doi:10.1021/acsami.7b04114
Kirby, D. J., and Young, M. F. (2018)., 143. Elsevier, 281–296.Isolation, production, and analysis of small leucine-rich proteoglycans in bone Methods Cell Biol. doi:10.1016/bs.mcb.2017.08.016
Ko, Y.-M., Choi, D.-Y., Jung, S.-C., and Kim, B.-H. (2015). Characteristics of plasma treated electrospun polycaprolactone (PCL) nanofiber scaffold for bone tissue engineering. J. Nanosci. Nanotechnol. 15 (1), 192–195. doi:10.1166/jnn.2015.8372
Kohli, N., Sharma, V., Orera, A., Sawadkar, P., Owji, N., Frost, O. G., et al. (2021). Pro-angiogenic and osteogenic composite scaffolds of fibrin, alginate and calcium phosphate for bone tissue engineering. J. Tissue Eng. 12, 204173142110056. doi:10.1177/20417314211005610
Kokubo, T., and Takadama, H. (2006). How useful is SBF in predicting in vivo bone bioactivity? Biomaterials 27 (15), 2907–2915. doi:10.1016/j.biomaterials.2006.01.017
Kong, X., Tang, Q., Chen, X., Tu, Y., Sun, S., and Sun, Z. (2017). Polyethylene glycol as a promising synthetic material for repair of spinal cord injury. Neural Regen. Res. 12 (6), 1003. doi:10.4103/1673-5374.208597
Koons, G. L., Diba, M., and Mikos, A. G. (2020). Materials design for bone-tissue engineering. Nat. Rev. Mat. 5 (8), 584–603. doi:10.1038/s41578-020-0204-2
Kostenuik, P., and Mirza, F. M. (2017). Fracture healing physiology and the quest for therapies for delayed healing and nonunion. J. Orthop. Res. 35 (2), 213–223. doi:10.1002/jor.23460
Kretlow, J. D., Hacker, M. C., Klouda, L., Ma, B. B., and Mikos, A. G. (2010). Synthesis and characterization of dual stimuli responsive macromers based on poly (N-isopropylacrylamide) and poly (vinylphosphonic acid). Biomacromolecules 11 (3), 797–805. doi:10.1021/bm9014182
Kumar, A., and Han, S. S. (2017). PVA-based hydrogels for tissue engineering: A review. Int. J. Polym. Mater. Polym. Biomaterials 66 (4), 159–182. doi:10.1080/00914037.2016.1190930
Kwon, H. J., and Han, Y. (2016). Chondroitin sulfate-based biomaterials for tissue engineering. Turk. J. Biol. 40 (2), 290–299. doi:10.3906/biy-1507-16
Lei, B., Guo, B., Rambhia, K. J., and Ma, P. X. (2019). Hybrid polymer biomaterials for bone tissue regeneration. Front. Med. 13 (2), 189–201. doi:10.1007/s11684-018-0664-6
Li, F., Zhu, Y., and Wang, Y. (2014). Dual-responsive drug delivery system with real time tunable release behavior. Microporous Mesoporous Mater. 200, 46–51. doi:10.1016/j.micromeso.2014.07.060
Li, G., Zhang, N., Zhao, S., Zhang, K., Li, X., Jing, A., et al. (2018). Fe-doped brushite bone cements with antibacterial property. Mater. Lett. 215, 27–30. doi:10.1016/j.matlet.2017.12.054
Li, H., Qi, Z., Zheng, S., Chang, Y., Kong, W., Fu, C., et al. (2019). The application of hyaluronic acid-based hydrogels in bone and cartilage tissue engineering. Adv. Mater. Sci. Eng. 2019, 1–12. doi:10.1155/2019/3027303
Li, X., and Chen, H. (2016). Yb3+/Ho3+ co-doped apatite upconversion nanoparticles to distinguish implanted material from bone tissue. ACS Appl. Mat. Interfaces 8 (41), 27458–27464. doi:10.1021/acsami.6b05514
Li, Y., Liu, X., Gaihre, B., Li, L., Rezaei, A., Miller, A. L., et al. (2022). Zinc-doped hydroxyapatite and poly (propylene fumarate) nanocomposite scaffold for bone tissue engineering. J. Mat. Sci. 57 (10), 5998–6012. doi:10.1007/s10853-022-06966-7
Li, Z., Li, S., Yang, J., Ha, Y., Zhang, Q., Zhou, X., et al. (2022). 3D bioprinted gelatin/gellan gum-based scaffold with double-crosslinking network for vascularized bone regeneration. Carbohydr. Polym. 290, 119469. doi:10.1016/j.carbpol.2022.119469
Lin, K., Zhang, D., Macedo, M. H., Cui, W., Sarmento, B., and Shen, G. (2019). Advanced collagen-based biomaterials for regenerative biomedicine. Adv. Funct. Mat. 29 (3), 1804943. doi:10.1002/adfm.201804943
Lin, K., Zhang, D., Macedo, M. H., Cui, W., Sarmento, B., and Shen, G. (2019). Advanced collagen-based biomaterials for regenerative biomedicine. Adv. Funct. Mat. 29 (3), 1804943. doi:10.1002/adfm.201804943
Lin, S., Huang, H., Zeng, Y., Zhang, L., and Hou, L. (2016). Facile surface modification by aldehydes to enhance chlorine resistance of polyamide thin film composite membranes. J. Membr. Sci. 518, 40–49. doi:10.1016/j.memsci.2016.06.032
Liu, M., Shu, M., Xu, W., Liu, X., Hou, Z., Xing, B., et al. (2019). BMP-2-Loaded HAp: Ln3+ (Ln= Yb, Er, Gd) nanorods with dual-mode imaging for efficient MC3t3-E1 cell differentiation regulation. Langmuir 35 (47), 15287–15294. doi:10.1021/acs.langmuir.9b02824
Liu, Q., Feng, L., Chen, Z., Lan, Y., Liu, Y., Li, D., et al. (2020). Ultrasmall superparamagnetic iron oxide labeled silk fibroin/hydroxyapatite multifunctional scaffold loaded with bone marrow-derived mesenchymal stem cells for bone regeneration. Front. Bioeng. Biotechnol. 8, 697. doi:10.3389/fbioe.2020.00697
Liu, X., and Ma, P. X. (2004). Polymeric scaffolds for bone tissue engineering. Ann. Biomed. Eng. 32 (3), 477–486. doi:10.1023/b:abme.0000017544.36001.8e
Liu, X., Zhao, K., Gong, T., Song, J., Bao, C., Luo, E., et al. (2014). Delivery of growth factors using a smart porous nanocomposite scaffold to repair a mandibular bone defect. Biomacromolecules 15 (3), 1019–1030. doi:10.1021/bm401911p
LogithKumar, R., KeshavNarayan, A., Dhivya, S., Chawla, A., Saravanan, S., and Selvamurugan, N. (2016). A review of chitosan and its derivatives in bone tissue engineering. Carbohydr. Polym. 151, 172–188. doi:10.1016/j.carbpol.2016.05.049
Lu, X., Liu, L., Feng, S., Pan, J., Li, C., and Zheng, Y. (2022). Preparation and biological properties of ZnO/hydroxyapatite/chitosan-polyethylene oxide@ gelatin biomimetic composite scaffolds for bone tissue engineering. J. Biomater. Appl. 37, 238–248. doi:10.1177/08853282221087110
Luo, Y., Li, D., Xie, X., and Kang, P. (2019). Porous, lithium-doped calcium polyphosphate composite scaffolds containing vascular endothelial growth factor (VEGF)-loaded gelatin microspheres for treating glucocorticoid-induced osteonecrosis of the femoral head. Biomed. Mat. 14 (3), 035013. doi:10.1088/1748-605x/ab0a55
Luo, Y., Li, D., Zhao, J., Yang, Z., and Kang, P. (2018). In vivo evaluation of porous lithium-doped hydroxyapatite scaffolds for the treatment of bone defect. Biomed. Mat. Eng. 29 (6), 699–721. doi:10.3233/bme-181018
Ma, D., Wang, Y., and Dai, W. (2018). Silk fibroin-based biomaterials for musculoskeletal tissue engineering. Mater. Sci. Eng. C 89, 456–469. doi:10.1016/j.msec.2018.04.062
Ma, L., Feng, X., Liang, H., Wang, K., Song, Y., Tan, L., et al. (2020). A novel photothermally controlled multifunctional scaffold for clinical treatment of osteosarcoma and tissue regeneration. Mater. Today 36, 48–62. doi:10.1016/j.mattod.2019.12.005
Ma, P. X. (2008). Biomimetic materials for tissue engineering. Adv. Drug Deliv. Rev. 60 (2), 184–198. doi:10.1016/j.addr.2007.08.041
Maharjan, B., Park, J., Kaliannagounder, V. K., Awasthi, G. P., Joshi, M. K., Park, C. H., et al. (2021). Regenerated cellulose nanofiber reinforced chitosan hydrogel scaffolds for bone tissue engineering. Carbohydr. Polym. 251, 117023. doi:10.1016/j.carbpol.2020.117023
Mallakpour, S., Tabesh, F., and Hussain, C. M. (2022). A new trend of using poly (vinyl alcohol) in 3D and 4D printing technologies: Process and applications. Adv. Colloid Interface Sci. 301, 102605. doi:10.1016/j.cis.2022.102605
Manicone, P. F., Iommetti, P. R., and Raffaelli, L. (2007). An overview of zirconia ceramics: Basic properties and clinical applications. J. Dent. 35 (11), 819–826. doi:10.1016/j.jdent.2007.07.008
Manicone, P. F., Iommetti, P. R., Raffaelli, L., Paolantonio, M., Rossi, G., Berardi, D., et al. (2007). Biological considerations on the use of zirconia for dental devices. Int. J. Immunopathol. Pharmacol. 20 (1), 9–12. doi:10.1177/039463200702001s03
Mao, D., Li, Q., Bai, N., Dong, H., and Li, D. (2018). Porous stable poly (lactic acid)/ethyl cellulose/hydroxyapatite composite scaffolds prepared by a combined method for bone regeneration. Carbohydr. Polym. 180, 104–111. doi:10.1016/j.carbpol.2017.10.031
Mao, Z., Fan, B., Wang, X., Huang, X., Guan, J., Sun, Z., et al. (2021). A systematic review of tissue engineering scaffold in tendon bone healing in vivo. Front. Bioeng. Biotechnol. 9, 621483. doi:10.3389/fbioe.2021.621483
Marsell, R., and Einhorn, T. A. (2011). The biology of fracture healing. Injury 42 (6), 551–555. doi:10.1016/j.injury.2011.03.031
Martins, C., Sousa, F., Araujo, F., and Sarmento, B. (2018). Functionalizing PLGA and PLGA derivatives for drug delivery and tissue regeneration applications. Adv. Healthc. Mat. 7 (1), 1701035. doi:10.1002/adhm.201701035
Miculescu, F., Maidaniuc, A., Voicu, S. I., Thakur, V. K., Stan, G. E., and Ciocan, L. T. (2017). Progress in hydroxyapatite–starch based sustainable biomaterials for biomedical bone substitution applications. ACS Sustain. Chem. Eng. 5 (10), 8491–8512. doi:10.1021/acssuschemeng.7b02314
Mishra, R., Bishop, T., Valerio, I. L., Fisher, J. P., and Dean, D. (2016). The potential impact of bone tissue engineering in the clinic. Regen. Med. 11 (6), 571–587. doi:10.2217/rme-2016-0042
Mittal, A., Negi, P., Garkhal, K., Verma, S., and Kumar, N. (2010). Integration of porosity and bio-functionalization to form a 3D scaffold: Cell culture studies and in vitro degradation. Biomed. Mat. 5 (4), 045001. doi:10.1088/1748-6041/5/4/045001
Miyagaki, A., Kamaya, Y., Matsumoto, T., Honda, K., Shibahara, M., Hongo, C., et al. (2019). Surface modification of poly (ether ether ketone) through friedel–crafts reaction for high adhesion strength. Langmuir 35 (30), 9761–9768. doi:10.1021/acs.langmuir.9b00641
Montoya, C., Du, Y., Gianforcaro, A. L., Orrego, S., Yang, M., and Lelkes, P. I. (2021). On the road to smart biomaterials for bone research: Definitions, concepts, advances, and outlook. Bone Res. 9 (1), 12–16. doi:10.1038/s41413-020-00131-z
Montoya, C., Du, Y., Gianforcaro, A. L., Orrego, S., Yang, M., and Lelkes, P. I. (2021). On the road to smart biomaterials for bone research: Definitions, concepts, advances, and outlook. Bone Res. 9 (1), 12–16. doi:10.1038/s41413-020-00131-z
Moorehead, C., Prudnikova, K., and Marcolongo, M. (2019). The regulatory effects of proteoglycans on collagen fibrillogenesis and morphology investigated using biomimetic proteoglycans. J. Struct. Biol. 206 (2), 204–215. doi:10.1016/j.jsb.2019.03.005
Moreno-Manzano, V., Mellado-López, M., Morera-Esteve, M. J., Alastrue-Agudo, A., Bisbal-Velasco, V., Forteza-Vila, J., et al. (2020). Human adipose-derived mesenchymal stem cells accelerate decellularized neobladder regeneration. Regen. Biomater. 7 (2), 161–169. doi:10.1093/rb/rbz049
Morsi, M. A., and Abd Elhamid, M. H. (2019). Effect of iron doped hydroxyapatite nanoparticles on the structural, morphological, mechanical and magnetic properties of polylactic acid polymer. J. Mater. Res. Technol. 8 (2), 2098–2106. doi:10.1016/j.jmrt.2019.01.017
Mouw, J. K., Ou, G., and Weaver, V. M. (2014). Extracellular matrix assembly: A multiscale deconstruction. Nat. Rev. Mol. Cell Biol. 15 (12), 771–785. doi:10.1038/nrm3902
Moztarzadeh, F., Farokhi, M., Mehrizi, A. A., Basiri, H., and Mohseni, S. S. (2021). Preparation of microfluidic-based pectin microparticles loaded carbon dots conjugated with BMP-2 embedded in gelatin-elastin-hyaluronic acid hydrogel scaffold for bone tissue engineering application. Int. J. Biol. Macromol. 184, 29–41. doi:10.1016/j.ijbiomac.2021.05.148
Narayanan, G., Vernekar, V. N., Kuyinu, E. L., and Laurencin, C. T. (2016). Poly (lactic acid)-based biomaterials for orthopaedic regenerative engineering. Adv. Drug Deliv. Rev. 107, 247–276. doi:10.1016/j.addr.2016.04.015
Neacsu, I. A., Stoica, A. E., Vasile, B. S., and Andronescu, E. (2019). Luminescent hydroxyapatite doped with rare Earth elements for biomedical applications. Nanomaterials 9 (2), 239. doi:10.3390/nano9020239
Nguyen, A. T., Sathe, S. R., and Yim, E. K. F. (2016). From nano to micro: Topographical scale and its impact on cell adhesion, morphology and contact guidance. J. Phys. Condens. Matter 28 (18), 183001. doi:10.1088/0953-8984/28/18/183001
Nikpour, P., Salimi-Kenari, H., Fahimipour, F., Rabiee, S. M., Imani, M., Dashtimoghadam, E., et al. (2018). Dextran hydrogels incorporated with bioactive glass-ceramic: Nanocomposite scaffolds for bone tissue engineering. Carbohydr. Polym. 190, 281–294. doi:10.1016/j.carbpol.2018.02.083
Noori, A., Ashrafi, S. J., Vaez-Ghaemi, R., Hatamian-Zaremi, A., and Webster, T. J. (2017). A review of fibrin and fibrin composites for bone tissue engineering. Int. J. Nanomedicine 12, 4937–4961. doi:10.2147/ijn.s124671
Nuss, K. M. R., and von Rechenberg, B. (2008). Biocompatibility issues with modern implants in bone-a review for clinical orthopedics. Open Orthop. J. 2, 66–78. doi:10.2174/1874325000802010066
O’Neill, J. D., Anfang, R., Anandappa, A., Costa, J., Javidfar, J., Wobma, H. M., et al. (2013). Decellularization of human and porcine lung tissues for pulmonary tissue engineering. Ann. Thorac. Surg. 96 (3), 1046–1056. doi:10.1016/j.athoracsur.2013.04.022
Padhi, A., and Nain, A. S. (2020). ECM in differentiation: A review of matrix structure, composition and mechanical properties. Ann. Biomed. Eng. 48 (3), 1071–1089. doi:10.1007/s10439-019-02337-7
Pathmanapan, S., Periyathambi, P., and Anandasadagopan, S. K. (2020). Fibrin hydrogel incorporated with graphene oxide functionalized nanocomposite scaffolds for bone repair—in vitro and in vivo study. Nanomedicine Nanotechnol. Biol. Med. 29, 102251. doi:10.1016/j.nano.2020.102251
Peng, X.-Y., Hu, M., Liao, F., Yang, F., Ke, Q.-F., Guo, Y.-P., et al. (2019). La-Doped mesoporous calcium silicate/chitosan scaffolds for bone tissue engineering. Biomater. Sci. 7 (4), 1565–1573. doi:10.1039/c8bm01498a
Pepla, E., Besharat, L. K., Palaia, G., Tenore, G., and Migliau, G. (2014). Nano-hydroxyapatite and its applications in preventive, restorative and regenerative dentistry: A review of literature. Ann. Stomatol. 5 (3), 108–114. doi:10.11138/ads/2014.5.3.108
Pereira, D. R., Canadas, R. F., Silva-Correia, J., da Silva Morais, A., Oliveira, M. B., Dias, I. R., et al. (2018). Injectable gellan-gum/hydroxyapatite-based bilayered hydrogel composites for osteochondral tissue regeneration. Appl. Mater. Today 12, 309–321. doi:10.1016/j.apmt.2018.06.005
Petretta, M., Gambardella, A., Desando, G., Cavallo, C., Bartolotti, I., Shelyakova, T., et al. (2021). Multifunctional 3D-printed magnetic polycaprolactone/hydroxyapatite scaffolds for bone tissue engineering. Polymers 13 (21), 3825. doi:10.3390/polym13213825
Pham Minh, D., Galera Martínez, M., Nzihou, A., and Sharrock, P. (2013). Thermal behavior of apatitic calcium phosphates synthesized from calcium carbonate and orthophosphoric acid or potassium dihydrogen orthophosphate. J. Therm. Anal. Calorim. 112 (3), 1145–1155. doi:10.1007/s10973-012-2695-6
Philippart, A., Gómez-Cerezo, N., Arcos, D., Salinas, A. J., Boccardi, E., Vallet-Regi, M., et al. (2017). Novel ion-doped mesoporous glasses for bone tissue engineering: Study of their structural characteristics influenced by the presence of phosphorous oxide. J. Non-Crystalline Solids 455, 90–97. doi:10.1016/j.jnoncrysol.2016.10.031
Pilipchuk, S. P., Plonka, A. B., Monje, A., Taut, A. D., Lanis, A., Kang, B., et al. (2015). Tissue engineering for bone regeneration and osseointegration in the oral cavity. Dent. Mater. 31 (4), 317–338. doi:10.1016/j.dental.2015.01.006
Popat, K. C., Chatvanichkul, K., Barnes, G. L., Latempa, T. J., Grimes, C. A., and Desai, T. A. (2007). Osteogenic differentiation of marrow stromal cells cultured on nanoporous alumina surfaces. J. Biomed. Mat. Res. A 80 (4), 955–964. doi:10.1002/jbm.a.31028
Prasad, A. (2021). State of art review on bioabsorbable polymeric scaffolds for bone tissue engineering. Mater. Today Proc. 44, 1391–1400. doi:10.1016/j.matpr.2020.11.622
Predoi, D., Iconaru, S. L., Predoi, M. V., Buton, N., and Motelica-Heino, M. (2019). Zinc doped hydroxyapatite thin films prepared by sol–gel spin coating procedure. Coatings 9 (3), 156. doi:10.3390/coatings9030156
Qasim, M., Chae, D. S., and Lee, N. Y. (2019). <p>Advancements and frontiers in nano-based 3D and 4D scaffolds for bone and cartilage tissue engineering</p>. Int. J. Nanomedicine 14, 4333–4351. doi:10.2147/ijn.s209431
Qasim, M., Chae, D. S., and Lee, N. Y. (2019). <p>Advancements and frontiers in nano-based 3D and 4D scaffolds for bone and cartilage tissue engineering</p>. Int. J. Nanomedicine 14, 4333–4351. doi:10.2147/ijn.s209431
Raucci, M. G., D’Amora, U., Ronca, A., Demitri, C., and Ambrosio, L. (2019). Bioactivation routes of gelatin-based scaffolds to enhance at nanoscale level bone tissue regeneration. Front. Bioeng. Biotechnol. 7, 27. doi:10.3389/fbioe.2019.00027
Ravindran, S., Gao, Q., Kotecha, M., Magin, R. L., Karol, S., Bedran-Russo, A., et al. (2012). Biomimetic extracellular matrix-incorporated scaffold induces osteogenic gene expression in human marrow stromal cells. Tissue Eng. Part A 18 (3–4), 295–309. doi:10.1089/ten.tea.2011.0136
Ribeiro, N., Nunes, C. M. M., Rodrigues, A. F. M., Sousa, A., and Olhero, S. M. (2022). Toughening robocast chitosan/biphasic calcium phosphate composite scaffolds with silk fibroin: Tuning printable inks and scaffold structure for bone regeneration. Biomater. Adv. 134, 112690. doi:10.1016/j.msec.2022.112690
Ripamonti, U., Crooks, J., Khoali, L., and Roden, L. (2009). The induction of bone formation by coral-derived calcium carbonate/hydroxyapatite constructs. Biomaterials 30 (7), 1428–1439. doi:10.1016/j.biomaterials.2008.10.065
Ripamonti, U., Crooks, J., Khoali, L., and Roden, L. (2009). The induction of bone formation by coral-derived calcium carbonate/hydroxyapatite constructs. Biomaterials 30 (7), 1428–1439. doi:10.1016/j.biomaterials.2008.10.065
Rodrigues, A. I., Gomes, M. E., Leonor, I. B., and Reis, R. L. (2012). Bioactive starch-based scaffolds and human adipose stem cells are a good combination for bone tissue engineering. Acta Biomater. 8 (10), 3765–3776. doi:10.1016/j.actbio.2012.05.025
Roseti, L., Parisi, V., Petretta, M., Cavallo, C., Desando, G., Bartolotti, I., et al. (2017). Scaffolds for bone tissue engineering: State of the art and new perspectives. Mater. Sci. Eng. C 78, 1246–1262. doi:10.1016/j.msec.2017.05.017
Roseti, L., Parisi, V., Petretta, M., Cavallo, C., Desando, G., Bartolotti, I., et al. (2017). Scaffolds for bone tissue engineering: State of the art and new perspectives. Mater. Sci. Eng. C 78, 1246–1262. doi:10.1016/j.msec.2017.05.017
Ryan, E. J., Ryan, A. J., González-Vázquez, A., Philippart, A., Ciraldo, F. E., Hobbs, C., et al. (2019). Collagen scaffolds functionalised with copper-eluting bioactive glass reduce infection and enhance osteogenesis and angiogenesis both in vitro and in vivo. Biomaterials 197, 405–416. doi:10.1016/j.biomaterials.2019.01.031
Sahoo, D. R., and Biswal, T. (2021). Alginate and its application to tissue engineering. SN Appl. Sci. 3 (1), 30–19. doi:10.1007/s42452-020-04096-w
Saito, M., and Marumo, K. (2015). Effects of collagen crosslinking on bone material properties in health and disease. Calcif. Tissue Int. 97 (3), 242–261. doi:10.1007/s00223-015-9985-5
Saveleva, M. S., Ivanov, A. N., Chibrikova, J. A., Abalymov, A. A., Surmeneva, M. A., Surmenev, R. A., et al. (2021). Osteogenic capability of vaterite-coated nonwoven polycaprolactone scaffolds for in vivo bone tissue regeneration. Macromol. Biosci. 21 (12), 2100266. doi:10.1002/mabi.202100266
Schmitt, S., Hümmer, J., Kraus, S., Welle, A., Grosjean, S., Hanke-Roos, M., et al. (2016). Tuning the cell adhesion on biofunctionalized nanoporous organic frameworks. Adv. Funct. Mat. 26 (46), 8455–8462. doi:10.1002/adfm.201603054
Sharma, A., Gupta, S., Sampathkumar, T. S., and Verma, R. S. (2022). Modified graphene oxide nanoplates reinforced 3D printed multifunctional scaffold for bone tissue engineering. Biomater. Adv. 134, 112587. doi:10.1016/j.msec.2021.112587
Sharma, A., Gupta, S., Sampathkumar, T. S., and Verma, R. S. (2022). Modified graphene oxide nanoplates reinforced 3D printed multifunctional scaffold for bone tissue engineering. Biomater. Adv. 134, 112587. doi:10.1016/j.msec.2021.112587
Sharma, R., Kuche, K., Thakor, P., Bhavana, V., Srivastava, S., Mehra, N. K., et al. (2022). Chondroitin Sulfate: Emerging biomaterial for biopharmaceutical purpose and tissue engineering. Ludhiana: Punjab Agricultural University.
Sharmila, G., Muthukumaran, C., Kirthika, S., Keerthana, S., Kumar, N. M., and Jeyanthi, J. (2020). Fabrication and characterization of Spinacia oleracea extract incorporated alginate/carboxymethyl cellulose microporous scaffold for bone tissue engineering. Int. J. Biol. Macromol. 156, 430–437. doi:10.1016/j.ijbiomac.2020.04.059
Shegarfi, H., and Reikeras, O. (2009). Review article: Bone transplantation and immune response. J. Orthop. Surg. Hong. Kong. 17 (2), 206–211. doi:10.1177/230949900901700218
Sikder, P., Bhaduri, S. B., Ong, J. L., and Guda, T. (2020). Silver (Ag) doped magnesium phosphate microplatelets as next-generation antibacterial orthopedic biomaterials. J. Biomed. Mat. Res. 108 (3), 976–989. doi:10.1002/jbm.b.34450
Singh, B. N., Veeresh, V., Mallick, S. P., Jain, Y., Sinha, S., Rastogi, A., et al. (2019). Design and evaluation of chitosan/chondroitin sulfate/nano-bioglass based composite scaffold for bone tissue engineering. Int. J. Biol. Macromol. 133, 817–830. doi:10.1016/j.ijbiomac.2019.04.107
Singh, B. N., Veeresh, V., Mallick, S. P., Sinha, S., Rastogi, A., and Srivastava, P. (2020). Generation of scaffold incorporated with nanobioglass encapsulated in chitosan/chondroitin sulfate complex for bone tissue engineering. Int. J. Biol. Macromol. 153, 1–16. doi:10.1016/j.ijbiomac.2020.02.173
Singh, B. N., Veeresh, V., Mallick, S. P., Sinha, S., Rastogi, A., and Srivastava, P. (2020). Generation of scaffold incorporated with nanobioglass encapsulated in chitosan/chondroitin sulfate complex for bone tissue engineering. Int. J. Biol. Macromol. 153, 1–16. doi:10.1016/j.ijbiomac.2020.02.173
Singh, R. P., Singh, J. P., Pal, A., and Kaur, T. (2020). Encapsulation of vancomycin in copper doped hydroxyapatite mesoporous nanoparticles of different morphologies. J. Drug Deliv. Sci. Technol. 55, 101441. doi:10.1016/j.jddst.2019.101441
Sionkowska, A. (2014). UV light as a tool for surface modification of polymeric biomaterials. Key Eng. Mat. 583, 80–86. doi:10.4028/www.scientific.net/kem.583.80
Skorulska, A., Piszko, P., Rybak, Z., Szymonowicz, M., and Dobrzyński, M. (2021). Review on polymer, ceramic and composite materials for cad/cam indirect restorations in dentistry—application, mechanical characteristics and comparison. Materials 14 (7), 1592. doi:10.3390/ma14071592
Sreekumaran, S., Radhakrishnan, A., Rauf, A. A., and Kurup, G. M. (2021). Nanohydroxyapatite incorporated photocrosslinked gelatin methacryloyl/poly (ethylene glycol) diacrylate hydrogel for bone tissue engineering. Prog. Biomater. 10 (1), 43–51. doi:10.1007/s40204-021-00150-x
Srinivasan, B., Kolanthai, E., Eluppai Asthagiri Kumaraswamy, N., Jayapalan, R. R., Vavilapalli, D. S., Catalani, L. H., et al. (2019). Thermally modified iron-inserted calcium phosphate for magnetic hyperthermia in an acceptable alternating magnetic field. J. Phys. Chem. B 123 (26), 5506–5513. doi:10.1021/acs.jpcb.9b03015
Stratton, S., Shelke, N. B., Hoshino, K., Rudraiah, S., and Kumbar, S. G. (2016). Bioactive polymeric scaffolds for tissue engineering. Bioact. Mater. 1 (2), 93–108. doi:10.1016/j.bioactmat.2016.11.001
Sun, H., Wu, C., Dai, K., Chang, J., and Tang, T. (2006). Proliferation and osteoblastic differentiation of human bone marrow-derived stromal cells on akermanite-bioactive ceramics. Biomaterials 27 (33), 5651–5657. doi:10.1016/j.biomaterials.2006.07.027
Surmenev, R. A., Shkarina, S., Syromotina, D. S., Melnik, E. v., Shkarin, R., Selezneva, I. I., et al. (2019). Characterization of biomimetic silicate-and strontium-containing hydroxyapatite microparticles embedded in biodegradable electrospun polycaprolactone scaffolds for bone regeneration. Eur. Polym. J. 113, 67–77. doi:10.1016/j.eurpolymj.2019.01.042
Tabaei, P. S. E., Asadian, M., Ghobeira, R., Cools, P., Thukkaram, M., Derakhshandeh, P. G., et al. (2021). Combinatorial effects of coral addition and plasma treatment on the properties of chitosan/polyethylene oxide nanofibers intended for bone tissue engineering. Carbohydr. Polym. 253, 117211. doi:10.1016/j.carbpol.2020.117211
Taghipour, Y. D., Hokmabad, V. R., Bakhshayesh, D., Rahmani, A., Asadi, N., Salehi, R., et al. (2020). The application of hydrogels based on natural polymers for tissue engineering. Curr. Med. Chem. 27 (16), 2658–2680. doi:10.2174/0929867326666190711103956
Tang, Y., Wu, C., Wu, Z., Hu, L., Zhang, W., and Zhao, K. (2017). Fabrication and in vitro biological properties of piezoelectric bioceramics for bone regeneration. Sci. Rep. 7 (1), 43360–43412. doi:10.1038/srep43360
Taylor, D. A., Lee, P.-F., Barac, Y., Hochman-Mendez, C., and Sampaio, L. C. (2020). “Decellularization of whole hearts for cardiac regeneration,” in Emerging technologies for heart diseases (Elsevier), 291–310. Netherlands
Terada, A., Okuyama, K., Nishikawa, M., Tsuneda, S., and Hosomi, M. (2012). The effect of surface charge property on Escherichia coli initial adhesion and subsequent biofilm formation. Biotechnol. Bioeng. 109 (7), 1745–1754. doi:10.1002/bit.24429
Thangprasert, A., Tansakul, C., Thuaksubun, N., and Meesane, J. (2019). Mimicked hybrid hydrogel based on gelatin/PVA for tissue engineering in subchondral bone interface for osteoarthritis surgery. Mater. Des. 183, 108113. doi:10.1016/j.matdes.2019.108113
Thomas, A., and Bera, J. (2019). Preparation and characterization of gelatin-bioactive glass ceramic scaffolds for bone tissue engineering. J. Biomaterials Sci. Polym. Ed. 30 (7), 561–579. doi:10.1080/09205063.2019.1587697
Titorencu, I., Georgiana Albu, M., Nemecz, M., and v Jinga, V. (2017). Natural polymer-cell bioconstructs for bone tissue engineering. Curr. Stem Cell Res. Ther. 12 (2), 1–174. doi:10.2174/1574888x11666160613195411
Trachtenberg, J. E., Placone, J. K., Smith, B. T., Fisher, J. P., and Mikos, A. G. (2017). Extrusion-based 3D printing of poly (propylene fumarate) scaffolds with hydroxyapatite gradients. J. Biomaterials Sci. Polym. Ed. 28 (6), 532–554. doi:10.1080/09205063.2017.1286184
Turnbull, G., Clarke, J., Picard, F., Riches, P., Jia, L., Han, F., et al. (2018). 3D bioactive composite scaffolds for bone tissue engineering. Bioact. Mater. 3 (3), 278–314. doi:10.1016/j.bioactmat.2017.10.001
Udomluck, N., Lee, H., Hong, S., Lee, S.-H., and Park, H. (2020). Surface functionalization of dual growth factor on hydroxyapatite-coated nanofibers for bone tissue engineering. Appl. Surf. Sci. 520, 146311. doi:10.1016/j.apsusc.2020.146311
Vahabzadeh, S., and Bose, S. (2017). Effects of iron on physical and mechanical properties, and osteoblast cell interaction in β-tricalcium phosphate. Ann. Biomed. Eng. 45 (3), 819–828. doi:10.1007/s10439-016-1724-1
Valerio, P., Pereira, M. M., Goes, A. M., and Leite, M. F. (2004). The effect of ionic products from bioactive glass dissolution on osteoblast proliferation and collagen production. Biomaterials 25 (15), 2941–2948. doi:10.1016/j.biomaterials.2003.09.086
Varma, S., Orgel, J. P. R. O., and Schieber, J. D. (2016). Nanomechanics of type I collagen. Biophysical J. 111 (1), 50–56. doi:10.1016/j.bpj.2016.05.038
Vranceanu, D. M., Parau, A. C., Cotrut, C. M., Kiss, A. E., Constantin, L. R., Braic, V., et al. (2019). In vitro evaluation of Ag doped hydroxyapatite coatings in acellular media. Ceram. Int. 45 (8), 11050–11061. doi:10.1016/j.ceramint.2019.02.191
Walimbe, T., and Panitch, A. (2020). Proteoglycans in biomedicine: Resurgence of an underexploited class of ECM molecules. Front. Pharmacol. 10, 1661. doi:10.3389/fphar.2019.01661
Wang, C., Huang, W., Zhou, Y., He, L., He, Z., Chen, Z., et al. (2020). 3D printing of bone tissue engineering scaffolds. Bioact. Mater. 5 (1), 82–91. doi:10.1016/j.bioactmat.2020.01.004
Wang, C., and Wang, M. (2012). Dual-source dual-power electrospinning and characteristics of multifunctional scaffolds for bone tissue engineering. J. Mat. Sci. Mat. Med. 23 (10), 2381–2397. doi:10.1007/s10856-012-4669-4
Wang, S., Lu, L., and Yaszemski, M. J. (2006). Bone-tissue-engineering material poly (propylene fumarate): Correlation between molecular weight, chain dimensions, and physical properties. Biomacromolecules 7 (6), 1976–1982. doi:10.1021/bm060096a
Wang, X., Jiang, M., Zhou, Z., Gou, J., and Hui, D. (2017). 3D printing of polymer matrix composites: A review and prospective. Compos. Part B Eng. 110, 442–458. doi:10.1016/j.compositesb.2016.11.034
Wang, X., Wang, Y., Gou, W., Lu, Q., Peng, J., and Lu, S. (2013). Role of mesenchymal stem cells in bone regeneration and fracture repair: A review. Int. Orthop. 37 (12), 2491–2498. doi:10.1007/s00264-013-2059-2
Wang, X., Xie, X., Zhang, G., Chen, S., Yao, D., He, K., et al. (2013). Exogenous phytoestrogenic molecule icaritin incorporated into a porous scaffold for enhancing bone defect repair. J. Orthop. Res. 31 (1), 164–172. doi:10.1002/jor.22188
Webster, T. J., Siegel, R. W., and Bizios, R. (1999). Osteoblast adhesion on nanophase ceramics. Biomaterials 20 (13), 1221–1227. doi:10.1016/s0142-9612(99)00020-4
Wei, J., Yan, Y., Gao, J., Li, Y., Wang, R., Wang, J., et al. (2022). 3D-printed hydroxyapatite microspheres reinforced PLGA scaffolds for bone regeneration. Biomater. Adv. 133, 112618. doi:10.1016/j.msec.2021.112618
Wu, C., Ramaswamy, Y., Soeparto, A., and Zreiqat, H. (2008). Incorporation of titanium into calcium silicate improved their chemical stability and biological propertiesThe Japanese Society for Biomaterials. J. Biomed. Mat. Res. Aand Aust. Soc. Biomaterials Korean Soc. Biomaterials 86 (2), 402–410. doi:10.1002/jbm.a.31623
Wu, C., Xia, L., Han, P., Mao, L., Wang, J., Zhai, D., et al. (2016). Europium-containing mesoporous bioactive glass scaffolds for stimulating in vitro and in vivo osteogenesis. ACS Appl. Mat. Interfaces 8 (18), 11342–11354. doi:10.1021/acsami.6b03100
Wu, C., and Xiao, Y. (2009). Evaluation of the in vitro bioactivity of bioceramics. Bone Tissue Regen. Insights 2 (1), 25–29.
Wu, C., and Xiao, Y. (2009). Evaluation of the in vitro bioactivity of bioceramics. Bone Tissue Regen. Insights 2 (1), 25–29.
Wu, D., Samanta, A., Srivastava, R. K., and Hakkarainen, M. (2017). Starch-derived nanographene oxide paves the way for electrospinnable and bioactive starch scaffolds for bone tissue engineering. Biomacromolecules 18 (5), 1582–1591. doi:10.1021/acs.biomac.7b00195
Xing, F., Chi, Z., Yang, R., Xu, D., Cui, J., Huang, Y., et al. (2021). Chitin-hydroxyapatite-collagen composite scaffolds for bone regeneration. Int. J. Biol. Macromol. 184, 170–180. doi:10.1016/j.ijbiomac.2021.05.019
Xing, F., Zhou, C., Hui, D., Du, C., Wu, L., Wang, L., et al. (2020). Hyaluronic acid as a bioactive component for bone tissue regeneration: Fabrication, modification, properties, and biological functions. Nanotechnol. Rev. 9 (1), 1059–1079. doi:10.1515/ntrev-2020-0084
Xu, L., Ma, F., Leung, F. K. L., Qin, C., Lu, W. W., and Tang, B. (2021). Chitosan-strontium chondroitin sulfate scaffolds for reconstruction of bone defects in aged rats. Carbohydr. Polym. 273, 118532. doi:10.1016/j.carbpol.2021.118532
Yang, M., Zhang, Z.-C., Yuan, F., Chen, Y., Deng, R.-H., Zhang, Z.-N., et al. (2021). Function and mechanism of RGD in bone and cartilage tissue engineering. Front. Bioeng. Biotechnol. 9, 773636. doi:10.3389/fbioe.2021.773636
Yang, P., and Moloney, M. G. (2017). Surface modification using crosslinking of diamine and a bis (diarylcarbene): Synthesis, characterization, and antibacterial activity via binding hydrogen peroxide. RSC Adv. 7 (47), 29645–29655. doi:10.1039/c7ra05258h
Yang, Y., Wang, K., Gu, X., and Leong, K. W. (2017). Biophysical regulation of cell behavior—Cross talk between substrate stiffness and nanotopography. Engineering 3 (1), 36–54. doi:10.1016/j.eng.2017.01.014
Yao, Q., Fuglsby, K. E., Zheng, X., and Sun, H. (2020). Nanoclay-functionalized 3D nanofibrous scaffolds promote bone regeneration. J. Mat. Chem. B 8 (17), 3842–3851. doi:10.1039/c9tb02814e
Ye, G., Bao, F., Zhang, X., Song, Z., Liao, Y., Fei, Y., et al. (2020). Nanomaterial-based scaffolds for bone tissue engineering and regeneration. Nanomedicine 15 (20), 1995–2017. doi:10.2217/nnm-2020-0112
Yeo, T., Ko, Y.-G., Kim, E. J., Kwon, O. K., Chung, H. Y., and Kwon, O. H. (2021). Promoting bone regeneration by 3D-printed poly (glycolic acid)/hydroxyapatite composite scaffolds. J. Industrial Eng. Chem. 94, 343–351. doi:10.1016/j.jiec.2020.11.004
Yin, H., Yang, C., Gao, Y., Wang, C., Li, M., Guo, H., et al. (2018). Fabrication and characterization of strontium-doped borate-based bioactive glass scaffolds for bone tissue engineering. J. Alloys Compd. 743, 564–569. doi:10.1016/j.jallcom.2018.01.099
Yin, J., Yu, J., Ke, Q., Yang, Q., Zhu, D., Gao, Y., et al. (2019). La-doped biomimetic scaffolds facilitate bone remodelling by synchronizing osteointegration and phagocytic activity of macrophages. J. Mat. Chem. B 7 (19), 3066–3074. doi:10.1039/c8tb03244k
Yongabi, D., Khorshid, M., Gennaro, A., Jooken, S., Duwé, S., Deschaume, O., et al. (2020). QCM-D study of time-resolved cell adhesion and detachment: Effect of surface free energy on eukaryotes and prokaryotes. ACS Appl. Mat. Interfaces 12 (16), 18258–18272. doi:10.1021/acsami.0c00353
Yuan, H., Kurashina, K., de Bruijn, J. D., Li, Y., de Groot, K., and Zhang, X. (1999). A preliminary study on osteoinduction of two kinds of calcium phosphate ceramics. Biomaterials 20 (19), 1799–1806. doi:10.1016/s0142-9612(99)00075-7
Yuan, Y., Yuan, Q., Wu, C., Ding, Z., Wang, X., Li, G., et al. (2019). Enhanced osteoconductivity and osseointegration in calcium polyphosphate bioceramic scaffold via lithium doping for bone regeneration. ACS Biomater. Sci. Eng. 5 (11), 5872–5880. doi:10.1021/acsbiomaterials.9b00950
Zeng, Y., Hoque, J., and Varghese, S. (2019). Biomaterial-assisted local and systemic delivery of bioactive agents for bone repair. Acta Biomater. 93, 152–168. doi:10.1016/j.actbio.2019.01.060
Zhang, D., Wu, X., Chen, J., and Lin, K. (2018). The development of collagen based composite scaffolds for bone regeneration. Bioact. Mater. 3 (1), 129–138. doi:10.1016/j.bioactmat.2017.08.004
Zhang, H., Migneco, F., Lin, C.-Y., and Hollister, S. J. (2010). Chemically-conjugated bone morphogenetic protein-2 on three-dimensional polycaprolactone scaffolds stimulates osteogenic activity in bone marrow stromal cells. Tissue Eng. Part A 16 (11), 3441–3448. doi:10.1089/ten.tea.2010.0132
Zhang, K., Wang, S., Zhou, C., Cheng, L., Gao, X., Xie, X., et al. (2018). Advanced smart biomaterials and constructs for hard tissue engineering and regeneration. Bone Res. 6 (1), 31–15. doi:10.1038/s41413-018-0032-9
Zhang, L., Dong, Y., Xue, Y., Shi, J., Zhang, X., Liu, Y., et al. (2019). Multifunctional triple-layered composite scaffolds combining platelet-rich fibrin promote bone regeneration. ACS Biomater. Sci. Eng. 5 (12), 6691–6702. doi:10.1021/acsbiomaterials.9b01022
Zhang, N., and Kohn, D. H. (2012). Using polymeric materials to control stem cell behavior for tissue regeneration. Birth Defects Res. Part C Embryo Today Rev. 96 (1), 63–81. doi:10.1002/bdrc.21003
Zhang, P., Wu, H., Wu, H., Lu, Z., Deng, C., Hong, Z., et al. (2011). RGD-conjugated copolymer incorporated into composite of poly (lactide-co-glycotide) and poly (L-lactide)-grafted nanohydroxyapatite for bone tissue engineering. Biomacromolecules 12 (7), 2667–2680. doi:10.1021/bm2004725
Zhang, X., Chen, Y., Han, J., Mo, J., Dong, P., Zhuo, Y., et al. (2019). Biocompatiable silk fibroin/carboxymethyl chitosan/strontium substituted hydroxyapatite/cellulose nanocrystal composite scaffolds for bone tissue engineering. Int. J. Biol. Macromol. 136, 1247–1257. doi:10.1016/j.ijbiomac.2019.06.172
Zhang, X., Li, Y., Chen, Y. E., Chen, J., and Ma, P. X. (2016). Cell-free 3D scaffold with two-stage delivery of miRNA-26a to regenerate critical-sized bone defects. Nat. Commun. 7 (1), 10376–10415. doi:10.1038/ncomms10376
Zhang, X., Li, Y., Chen, Y. E., Chen, J., and Ma, P. X. (2016). Cell-free 3D scaffold with two-stage delivery of miRNA-26a to regenerate critical-sized bone defects. Nat. Commun. 7 (1), 10376–10415. doi:10.1038/ncomms10376
Zhang, X., and Williams, D. (2019). Definitions of biomaterials for the twenty-first century. Elsevier. Netherlands
Zhao, M. Y., Li, L. H., Li, B., and Zhou, C. R. (2014). LBL coating of type I collagen and hyaluronic acid on aminolyzed PLLA to enhance the cell-material interaction. Express Polym. Lett. 8 (5), 322–335. doi:10.3144/expresspolymlett.2014.36
Zhou, J., Zhang, X., Sun, J., Dang, Z., Li, J., Li, X., et al. (2018). The effects of surface topography of nanostructure arrays on cell adhesion. Phys. Chem. Chem. Phys. 20 (35), 22946–22951. doi:10.1039/c8cp03538e
Zhu, D.-Y., Lu, B., Yin, J.-H., Ke, Q.-F., Xu, H., Zhang, C.-Q., et al. (2019). <p>Gadolinium-doped bioglass scaffolds promote osteogenic differentiation of hBMSC via the Akt/GSK3&beta; pathway and facilitate bone repair in vivo</p>. Int. J. Nanomedicine 14, 1085–1100. doi:10.2147/ijn.s193576
Zhuang, H., Lin, R., Liu, Y., Zhang, M., Zhai, D., Huan, Z., et al. (2019). Three-dimensional-printed bioceramic scaffolds with osteogenic activity for simultaneous photo/magnetothermal therapy of bone tumors. ACS Biomater. Sci. Eng. 5 (12), 6725–6734. doi:10.1021/acsbiomaterials.9b01095
Ziminska, M., Chalanqui, M. J., Chambers, P., Acheson, J. G., McCarthy, H. O., Dunne, N. J., et al. (2019). Nanocomposite-coated porous templates for engineered bone scaffolds: A parametric study of layer-by-layer assembly conditions. Biomed. Mat. 14 (6), 065008. doi:10.1088/1748-605x/ab3b7b
Glossary
TE Tissue engineering
BTE Bone Tissue engineering
MSCs Mesenchymal stem cells
ECM Extracellular matrix
HAp Hydroxyapatite
TCP Tricalcium phosphate
3D Three dimensional
PLA Poly lactic acid
PVA Polyvinyl alcohol
PGA Polyglycolic acid
PMMA Polymer of acrylamide
Dex-U Urethacrylate dextran PLLA Poly(L-lactic acid)
PADH Polymer of acrylamide -Urethacrylate dextran
BG Bioactive glass
HNTs Halloysite nanotubes
PMPV Polyacrylamide/poly (vinyl alcohol)
AM Additive manufacturing
PVA/SA/HA Polyvinyl alcohol/sodium alginate/hydroxyapatite
MGO Magnetic graphene oxide
GAG Glycosaminoglycan
SLRPs Small leucine-rich proteoglycans
TNF-alpha Tumor necrosis factor-alpha
BMPs Bone morphogenetic proteins
VEGF Vascular endothelial growth factor
Col Collagen
Gel Gelatin
Ch Chitosan
HA Hyaluronic acid
PD-RGO Polydopamine-reduced graphene oxide
SF Silk fibroin
AA Amino acid
CS Chondroitin sulfate
Alg Alginate
SBF Simulated bodily fluids
rhBMP-2 Recombinant human bone morphogenetic protein
EDC 1-Ethyl-3-(3-dimethylaminopropyl)carbodiimide
NHS N-hydroxysuccinimide
CL Cellulose
PLGA Poly (lactic-co-glycolic acid)
GFs Growth factors
PEG Polyethylene glycol
PCL Poly ɛ-caprolactone
ICP-OES Inductively coupled plasma-optical emission spectrometry
HP Hyperbranched polymer
PU Polyurethane
PPF Poly(propylene fumarate)
QDs Quantum dots
rBMSC Recombinant Bone marrow stromal cells
SMAD Mothers against decapentaplegic
hASC Human adult stromal cells
SBMs Smart biomaterials
PVDF Polyvinylidene fluoride
SAMs Self assembled monolayers
CNTs Carbon nanotubes
f-MWCNT Multiwalled Carbon Nanotubes
ERMs Enzyme-responsive materials
Keywords: scaffold, bone tissue engineering, bioactivity, regenerative medicine, composite matrix
Citation: Kumari S, Katiyar S, Darshna , Anand A, Singh D, Singh BN, Mallick SP, Mishra A and Srivastava P (2022) Design strategies for composite matrix and multifunctional polymeric scaffolds with enhanced bioactivity for bone tissue engineering. Front. Chem. 10:1051678. doi: 10.3389/fchem.2022.1051678
Received: 25 September 2022; Accepted: 14 November 2022;
Published: 28 November 2022.
Edited by:
Jianhong Zhou, Baoji University of Arts and Sciences, ChinaCopyright © 2022 Kumari, Katiyar, Darshna, Anand, Singh, Singh, Mallick, Mishra and Srivastava. This is an open-access article distributed under the terms of the Creative Commons Attribution License (CC BY). The use, distribution or reproduction in other forums is permitted, provided the original author(s) and the copyright owner(s) are credited and that the original publication in this journal is cited, in accordance with accepted academic practice. No use, distribution or reproduction is permitted which does not comply with these terms.
*Correspondence: Pradeep Srivastava, cGtzcml2YXN0YXZhLmJjZUBpdGJodS5hYy5pbg==