- 1Enterprise Technology Center of Guizhou Province, Guizhou University, Guiyang, China
- 2Key Laboratory of Macrocyclic and Supramolecular Chemistry of Guizhou Province, Guizhou University, Guiyang, China
The semiconductors, such as TiO2, CdS, ZnO, BiVO4, graphene, produce good applications in photocatalytic water splitting for hydrogen production, and great progress have been made in the synthesis and modification of the materials. As a two-dimensional layered structure material, graphitic carbon nitride (g-C3N4), with the unique properties of high thermostability and chemical inertness, excellent semiconductive ability, affords good potential in photocatalytic hydrogen evolution. However, the related low efficiency of g-C3N4 with fast recombination rate of photogenerated charge carriers, limited visible-light absorption, and low surface area of prepared bulk g-C3N4, has called out the challenge issues to synthesize and modify novel g-C3N4-block photocatalyst. In this review, we have summarized several strategies to improve the photocatalytic performance of pristine g-C3N4 such as pH, morphology control, doping with metal or non-metal elements, metal deposition, constructing a heterojunction or homojunction, dye-sensitization, and so forth. The performances for photocatalytic hydrogen evolution and possible development of g-C3N4 materials are shared with the researchers interested in the relevant fields hereinto.
1 Introduction
With the development and progress of human society, environmental pollution and energy shortage have become two major problems that plague human beings. Hydrogen is considered as one of the best candidates for storing solar energy meeting the growing clean energy demand (Chen et al., 2016; Shen et al., 2016; Wang et al., 2016; Wu et al., 2017; Wang et al., 2018; Liu et al., 2019). Since Fujishima and Honda discovered the hydrogen evolution reaction activates by TiO2 under irradiation in 1972, photocatalytic water splitting is one of the promising means for hydrogen production (Fujishima and Honda., 1972). Without relying on fossil reserves, the photocatalytic hydrogen evolution from water with highly efficient utilization of solar irradiation is a desirable exploration for the solution of the energy issues (Maeda et al., 2006; Qi et al., 2022). Although great process in photocatalysts of water splitting have been made for H2 evolution under visible light, there are still challenging and concerns with semiconductors to promise hydrogen energy development methods (Zhong et al., 2015; Wang et al., 2016; Zhang et al., 2018; Qi et al., 2020).
Graphitic carbon nitride (g-C3N4) is considered as an ideal 2D material with the conjugated skeleton for photocatalytic water splitting with the activity of photoelectronic chemistry and high stability in the photochemical reaction (Ong et al., 2016). Compounds in rich carbon and nitrogen elements such as melamine, urea, cyanamide, dicyandiamide, cyanuric acid, etc. are usually subjected as the precursors. Graphitic carbon nitride materials were synthesized by methods including electro-chemical deposition, thermal shrinkage polymerization, solid phase synthesis, gas phase synthesis, solvothermal synthesis and electrochemical deposition (Thomas et al., 2008). Under light irradiation, electron-hole pairs were generated on the surface of g-C3N4 photocatalyst to provide the reaction sites. The water molecules adsorbed on the surface of g-C3N4 undergo the photocatalytic reduction for H2 evolution and oxidation for O2 release, respectively, with the efficacious charge carriers by the reactions (1–3):
Oxidation:
Reduction:
Overall reaction:
The first case of g-C3N4 as a polymeric photocatalyst for water splitting to produce H2 under visible-light irradiation was reported by Wang et al. (Wang et al., 2009) Figure 1 schematically described the photogeneration of H2 and O2 in water splitting reaction with the pristine g-C3N4. The obtained bulk form of g-C3N4 exhibited some drawbacks including limited visible light utilization efficiency, fast recombination rate of photogenerated electron-hole pairs, and low specific surface areas (<10 m2g−1), which still limited the photocatalytic performance of on its practical applications (Reza et al., 2015; Fu et al., 2018), and modification of g-C3N4 has been recognized to be the effective way to improve the photocatalytic performance of pristine g-C3N4.
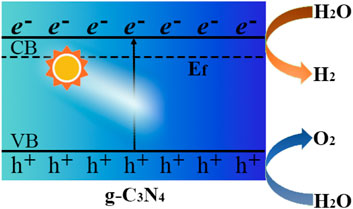
FIGURE 1. Schematic of generation of H2 and O2 from water with the catalysis of pristine g-C3N4 under light irradiation.
2 Modification of graphitic carbon nitride materials
Recently, the application of g-C3N4 with improved photocatalytic performance by developed several strategies, involving adjusting pH value, morphology control, doping by heteroatoms or metals, participation of co-catalyst, dye-sensitization, and construction of heterojunction. The hydrogen evolution performance of the modified g-C3N4-based materials are summarized in Table 1 to provide the development of the co-catalysts in the photolysis system.
2.1 pH
The pH value of solution was an important factor affecting the activity of g-C3N4, that is, Zeta potential values suggested the surface charge of g-C3N4 could be changes at different pH value for the diversity of functional groups on the surface (Wang et al., 2016). Wu et al. demonstrated that the alkaline environment was beneficial to the photocatalytic hydrogen evolution efficiency of g-C3N4 material as shown in Figure 2A (Wu et al., 2014). The experimental results show that pH and methanol have certain effects on the photocurrent amplification on g-C3N4 films. In the presence of methanol, the photoelectronic efficiency was improved to provide an increased photocurrent from 0.6 to 1.2 μA cm−2, which was further enhanced to offer a 4.2 μA cm−2 current upon adding base to bring the pH to 12.8. The results implied the transfer of photogenerated holes into solution was enhanced by the addition of methanol and alkali, which could root in the additive-induced decrease of the energy gap of the flat band and band-edge of g-C3N4 as description in Figure 2B, that is, methanol oxidation occurred in alkaline solution, but restrained in acidic condition with the amine-terminated g-C3N4 surface.
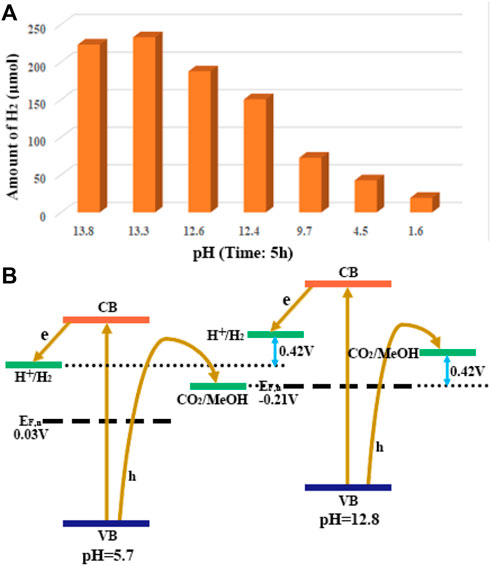
FIGURE 2. (A) the relationship of pH values to the efficiencies of H2 evolution with g-C3N4 photolysis; (B) energy diagrams of g-C3N4 in solution at pH 5.7 and 12.8.
2.2 Morphology control
The activity of g-C3N4 for H2 production via water splitting under visible-light irradiation could be determined by morphology of the material surface (Niu et al., 2012; Zhang et al., 2012; Han et al., 2015). The targets of controllable morphologies in preparation of well-defined g-C3N4 nanostructures to get larger specific surface area and more abundant reactive sites, reduced the recombination rate of photogenerated charge carriers. There were different nanostructures of g-C3N4 have been described in pioneering reports involving zero-dimensional (Wang et al., 2014) (0D), one-dimensional (Bai et al., 2013; Zhang et al., 2013; Wang et al., 2015; Mo et al., 2018; Bashir et al., 2019; Zhang et al., 2020) (1D), two-dimensional (Li et al., 2015; Zhao et al., 2018; Qi et al., 2019; Shi et al., 2022) (2D), three-dimensional (Li et al., 2016; Di et al., 2018; Chen et al., 2019) (3D) as shown in Figure 3, which built an ideal platform for collectively advanced photoredox processes for the enormous advantages in terms of physical and chemical characterization in following details.
The photocatalytic performance of 0D nanostructured materials are dependence on the natures including quantum size effect, small size effect, surface effect, macroscopic quantum effect and so on. Prof. Yu and co-workers prepared graphitic carbon nitride quantum dot structures directly from g-C3N4 with a thermochemical etching process, which produced unique upconversion properties and higher hydrogen production efficiency than original g-C3N4 in 2.87 times (Wang et al., 2014).
There was more explosion of active sites on the surface of 1D g-C3N4, which was reported as nanotubes (Mo et al., 2018; Guo et al., 2021), nanowires (Zhang et al., 2013; Wang et al., 2015), nanorods (Bai et al., 2013; Bashir et al., 2019) and so on, and efficient transfer of photogenerated electrons could be realized along one-dimensional paths with enhancement of visible light absorption and fast short-distance electron transport. Mo et al. developed g-C3N4 nanotubes with large number of nitrogen defects by a green-, acid- and base-free synthesis method, and the hydrogen production of 118.5 μmol h−1 was far superior to pristine g-C3N4 (Mo et al., 2018).
Compared with 1D structures, 2D photocatalysts have greater potential because of their larger specific surface area and thinner thickness, exposing more active sites and shortening the transport path of photogenerated carriers. Prof. Zhu’s group successfully fabricated g-C3N4 nanosheets with a single atomic layer structure of only 0.4 nm thickness, with a simple chemical exfoliation method. The single-atom-layer nanosheets offered better separation and transfer rates of photogenerated carriers, and exhibited higher performance than bulk g-C3N4 in photocatalytic splitting of water for hydrogen production and photocurrent generation. Chen’s group proposed that the precursors assembled into nanorods at low power level, while grew into nanoplates at high power level, which implied that the morphology of g-C3N4 was dependance upon on a kinetically driven process (Li et al., 2015). Zhao et al. treated supramolecular precursors under the action of glycerol and ethanol to obtain porous few-layer g-C3N4 (Zhao et al., 2018). The hydrogen evolution rate of thin-layer g-C3N4 was evaluated to be 159.8 μmol h−1, as the results of its large specific surface area, more active sites, and the abundant nitrogen vacancies in the framework, to accelerate the transfer of photogenerated electrons.
Compared with 2D g-C3N4 nanosheets, the porous 3D g-C3N4 material can provide a larger specific surface area. It also maximizes the use of incident photons through multiple reflections within the interconnected open frame (Di et al., 2018). In addition, the porous 3D g-C3N4 material acted as a support to prevent the agglomeration of ultrathin nanosheets and provided a pathway for electron transfer, thereby greatly enhancing the photocatalytic activity (Li et al., 2016). Zhang et al. utilized a simple bottom-up supramolecular self-assembly route to assemble a porous 3D g-C3N4 with high crystallinity and applied it to photocatalytic water splitting (Chen et al., 2019). In 2022, Guo et al. reported a facile template-free self-assembly method to synthesize three-dimensional porous g-C3N4 nanovesicles for achieving efficient and durable photocatalytic generation of H2, and the large-size vesicles exhibited the high H2 production rate of 10.3 mmol h−1 g−1 (Sun et al., 2022). And 3D onion-ring-like g-C3N4 was made from silica microsphere as a hard-template, which affored excellent properties such as large specific surface area, strong optical absorption, high dispersion, for the efficient water splitting with 5-fold higher than that of pristine g-C3N4 (Cui et al., 2018; Shi et al., 2022).
2.3 Doping
Graphitic carbon nitride, as a conjugated polymeric material with a band gap of about 2.7 eV, has a relatively narrow response to visible-light. Numerous research results suggested that the optical properties and some other physical properties of g-C3N4 could be well regulated by doping foreign elements (Chen et al., 2017; Zeng et al., 2018; Fang et al., 2019; Sun H.,R. et al., 2022a). Therefore, the photocatalytic activity of pure g-C3N4 could be improved by hybridization with a small amounts of non-metals or metals into the framework.
2.3.1 Non-Metal doping
Hybridization of non-metallic dopants such as B, S, O, P and I to realize the ingenious design of the electronic structure, was considered as an important method for the improvement of g-C3N4 performance (Qi et al., 2021). Non-metal doping refers to doping of some non-metal elements into the structural framework, which not only modified the electronic and textural properties of g-C3N4 photocatalyst, but also improved the separation efficiency of photogenerated charge carriers and finally boosted the photocatalytic activity. Fang and coworkers (Fang et al., 2019) reported P-doped g-C3N4 for photocatalytic water splitting, and 4-(diphenylphosphino)benzoic acid (4-DPPBA) was employed as the precursor of phosphorous. The combination of P-doping and thermal exfoliation was applied for the preparation of porous g-C3N4 with P hybridization, which afford excellent photocatalysis for hydrogen evolution high to 1,596 μmol h−1 g−1 under irradiation of visible light (Ran et al., 2015). As demonstrated by DFT and experimental studies, the empty intermediate bandgap state enhanced the photo sensitivity with P hybridization, and the mass transfer process and light trapping were improved on the macroporous structure. The intrinsic energy gap of g-C3N4 was decrease from 2.98 to 2.66 eV in the attendance of P dopant. On the other hand, Lin and co-workers discovered the B,F-doped g-C3N4 porous nanosheets were achieved by the self-polymerization of urea in the presence of ionic liquid [Bmim][BF4], which yielded photocatalytic hydrogen in 3.9 times higher than pristine g-C3N4 (Lin and Wang., 2014) (Zhang et al., 2014). successfully obtained iodine-doped carbon nitride (CN-I) with calcining dicyandiamide to significantly improve the hydrogen production performance (Zhang et al., 2014). The photocatalytic activity of iodine-doped g-C3N4 was occurred at the wavelength of 600 nm, while pristine g-C3N4 provided inactive catalysis at 500 nm. Guo et al. (Guo et al., 2016) prepared a phosphorus-doped hexagonal hollow tubular structure g-C3N4 by hydrothermal method and the special structure greatly increased the specific surface area of the catalyst, thereby increasing the number of active sites for hydrogen production. Carbon doping is also an important part of non-metal dopants. In 2021, Liu et al. reported the synthesis of C-doped g-C3N4 by one-step copolymerization using melamine and chitosan as the raw materials (Liu et al., 2021). The N atom in g-C3N4 matrix was replaced by C to form the delocalized big Π bonds. The prepared C-doped g-C3N4 exhibited an excellent photocatalytic H2 evolution activity of 1,224 mmol h−1 g−1, which was 4.5 times than the free g-C3N4.
2.3.2 Metal doping
In addition to the doping of non-metallic elements, the g-C3N4 framework was also doped with metallic elements to modify the electronic energy band structure, thereby improving the visible-light absorption, and enhancing the migration and separation of photogenerated carriers in the g-C3N4 photocatalyst (Chen et al., 2016). reported the Co-doped g-C3N4 synthesized by one-step thermal polymerization of cobalt phthalocyanine (CoPc) and melamine as the precursors (Chen et al., 2017). Yue et al. used a simple chemical method to dope metallic Zn into g-C3N4 (Yue et al., 2011). When the content of Zn was 10%, the visible-light-generated hydrogen production activity was 10 times higher than the pure g-C3N4. The proposed mechanism implied that doping of Zn increased the light absorption, improved the separation efficiency of electron-hole pairs, and enabled more electrons for water splitting. However, it is still a challenge to obtain nanoparticles with uniform size, regular shape, and high stability with common precursors such as polymers, carbon supports, ionic liquids, surfactants and microemulsions. In the recent report of our group, the coordination complex of cucurbit [6]uril and Co2+ was developed as the precursor, to produce cobalt nanoparticles with themolysis, for photocatalytic electrolysis of water by deposition on the surface of the g-C3N4 film. (Dai et al., 2022). The formed semiconductor-metal interface provided more reaction sites and electron transport channels for effective charge carriers to capture photons and excite electrons, thereby, promoting the photoelectrocatalytic reaction process. The discovery provided a new strategy for exploring macrocyclic/g-C3N4 materials with excellent photocatalytic activities.
2.4 Metal deposition (co-catalyst)
Various studies suggested that metal deposition on pure g-C3N4 was also one of the promising methods to enhance the photocatalytic activity. In theory, when metal nanoparticles are in contact with g-C3N4, a Schottky junction is formed at the interface of metal and g-C3N4 semiconductor due to the different work function, which changes the electron distribution on the semiconductor surface (Naseri et al., 2017; Caux et al., 2019; Qi et al., 2020; Zhao et al., 2021). The main function of metal is to accept the photogenerated electrons from the CB of g-C3N4 during the photocatalytic H2 production process. Various metals such as Pt (Ou et al., 2017; Zhu et al., 2019), Au (Samanta et al., 2014; Caux et al., 2019), Pd (Xiao et al., 2019), Ag (Nagajyothi et al., 2017; Deeksha et al., 2021) and Ni (Indra et al., 2016; Kong et al., 2016) were employed as co-catalyst for the efficient sensitization for photocatalysis with the surface plasmon resonance (SPR) effect, which improved the light absorption capacity of the catalyst. With an in situ photoreduction, Pt/g-C3N4 was subjected to be a visible light photocatalyst by Wang’s group, and the results indicated that the photocatalytic hydrogen evolution capability was gradually enhanced as the size decrease of the Pt co-catalyst (Zhu et al., 2019). The participation of Pt provided more active sites on the surface for reduction, which was favorable for accepting electrons from CB of g-C3N4, due to the formation of Schottky junctions at the interface of Pt and g-C3N4. The PL spectra and UV-vis/DRS spectra of g-C3N4 and Ptx-CN with different Pt content, demonstrated that Pt loading greatly improved charge separation and transfer in g-C3N4 photocatalysts, thereby reduced charge recombination, and enhanced photocatalytic activity, as well as provided the maximum utilization efficiency photocatalytic performance for H2 production. The Pt0.1-CN (with 0.1wt% Pt loading amount) sample displayed the highest photocatalytic activity with H2 evolution of 473.82 μmol mg−1 under visible-light irradiation.
Furthermore, Bi et al. reported a Ni cocatalyst for the enhancement of photocatalytic performance of g-C3N4 (Bi et al., 2015). A higher separation efficiency of photogenerated charge carriers was obtained as a result of a deeper band bending of g-C3N4 contacting with Ni, which contributed to enhanced photocatalytic H2 production performance. In addition, the heterojunction formed between the Ni nanoparticles and g-C3N4 acted as an electron collector, and impeded the recombination rate of photogenerated electron and holes as illumination in Figure 4. Ni/g-C3N4 catalyst exhibited high photocatalytic H2 evolution rate (8.314 μmol h−1) compared with pristine g-C3N4, in which rapid recombination between conduction band (CB) and valence band (VB) holes and the quick reversible reaction occurred.
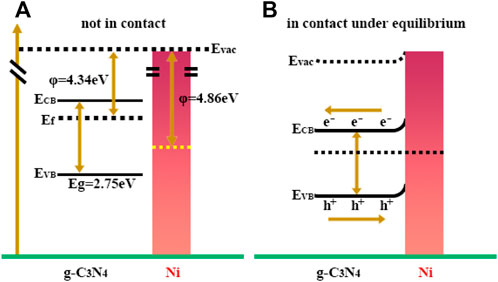
FIGURE 4. Schematic graph shows (A) the energy level diagram of g-C3N4 and Ni; (B) the interfacial electron transfer between g-C3N4 and Ni under irradiation.
2.5 Dye sensitization
To overcome the g-C3N4 absorption edge of a band gap of 2.7 eV, organic dyes were employed as a driver to improve the visible-light photoactivity (Bard and Fax., 1995; Kudo and Miseki., 2009; Kim et al., 2015), which were considered to dramatically extend the visible-light region of the band-gap of semiconductor (Zhuang et al., 2019). However, the researches about H2 production based on dye-sensitized carbon nitride were still insufficient, only few organic dyes such as metal-porphyrins (Yu et al., 2014; Chen et al., 2015; Zhang et al., 2015; Zhuang et al., 2019; Liu et al., 2020), poly (3-hexylthiophene) (Zhang et al., 2015), eosin Y (EY) (Min and Liu., 2012; Wang et al., 2018; Qi et al., 2019; Xu et al., 2019; Nagaraja et al., 2020; Zhao et al., 2021) and erythrosin B (ErB) (Wang et al., 2013; Zhang et al., 2017; Zhang et al., 2017) have been successfully applied to enhance the photocatalytic activity with improvement of the utilization efficiency of visible-light. In the process of H2 generation, the organic dyes were damaged in oxidation reactions, and its stabilization could be realized with a porous support, which accelerates the transfer of electrons from the excited dye molecule to the active site in definition of a cocatalyst, in general use of noble metals (especially Pt). The hybridization of Ag with g-C3N4 was applied for hydrogen evolution, and the photocatalysis was improved with the dye-sensitization under visible-light irradiation (Schwinghanmmer et al., 2013). Min et al. reported that g-C3N4 with modification of Eosin Y performed the light-drove H2 generation at about 600 nm, while the reaction occurred at less than 460 nm on the pristine g-C3N4 surface (Min and Liu., 2012).
2.6 Heterogeneous structure
The photocatalytic efficiency and application of pristine g-C3N4 were limited for high recombination rate of photogenerated charge carriers and narrow range of visible light response in a solar spectrum. Recently, g-C3N4-based heterojunctions were developed by enhancement of carrier separation efficiency and demonstrated excellent photocatalytic performance. Semiconductors were induced to form heterojunctions with g-C3N4 including carbon materials (graphene (Xiang et al., 2011), carbon nanotubes (Ge and Han., 2012), fullerenes (Chai et al., 2014)), metal oxides (TiO2(Chen and Liu, 2016), SnO2(Zada et al., 2019), ZnO(Sun et al., 2012), NiFe2O4(Liu et al., 2022), Fe2O3(Theerthagiri et al., 2014)), metal sulfides (CdS(Chen et al., 2016), ZnS(Shi et al., 2014), MoS2(Li et al., 2014)), bismuth-based compounds (BiPO4(Zou et al., 2015), BiVO4(Li et al., 2014), Bi2WO6(Li et al., 2018)), silver-based compounds (Ag2O(Liang et al., 2019), Ag3PO4(Liu et al., 2016), Ag3VO4(Zhu et al., 2015)), multi-element rare Earth oxides (Zn2GeO4(Sun et al., 2014), SrTiO3(Xu et al., 2011)), etc. The principle was executed in design of the heterojunction, that is, the recombination of g-C3N4 and the band-matched semiconductor promoted the transfer of charge carriers and suppressed the recombination of charges.
Based on different photogenerated carrier transfer mechanisms, the heterojunctions were formed when g-C3N4 coupled with other materials (Reza et al., 2015; Patnaik et al., 2016; Fu et al., 2018). In the heterojunction structures, Type-Ⅰ constructure refers to that the position of CB of semiconductor-1 is higher than that of semiconductor-2, while the VB position of semiconductor-1 is lower than that of semiconductor-2, as shown in Figure 5A. Under the excitation of visible light, electrons and holes of the Type-Ⅰ heterojunction photocatalyst are more inclined to migrate to the semiconductor-2 with a smaller band gap and undergo a redox reaction, and the separation efficiency of carriers is not significantly improved, resulting in the low rate of photocatalytic redox reaction. In the Type-Ⅱ structure, the positions of both CB and VB of semiconductor-2 are lower than those of semiconductor-1, and therefore the photogenerated electrons and holes transferr into different sides of the heterostructure, as shown in Figure 5B. The carrier transport mode of the Type-Ⅱ heterojunction greatly improve the photocatalytic activity of the composite photocatalyst. In 2021, Roy’s team reported the TiO2/ultrathin g-C3N4 (U-g-CN) heterostructure photocatalyst using a unique in situ thermal exfoliation process, and the presence of U-g-CN produced a redshift (∼0.13eV) in the absorption edge of heterostructures compared to that of bare TiO2, which extended the light absorption capability. Combined with the morphological characteristics of g-C3N4, Chen et al. prepared a novel 3D hierarchical hollow tubular g-C3N4/ZnIn2S4 nanosheets as the type-Ⅱ heterojunction photocatalyst (Chen et al., 2022). The optimum photocatalyst offered the H2 evolution rate up to 20,738 μmol h−1 g−1. In the case of the Type-Ⅲ heterojunction (Figure 5C), there are no any energy band intersection of semiconductor-1 and semiconductor-2, resulting in the inability of transport of photogenerated carriers between the semiconductors to greatly improve the photocatalytic efficiency.
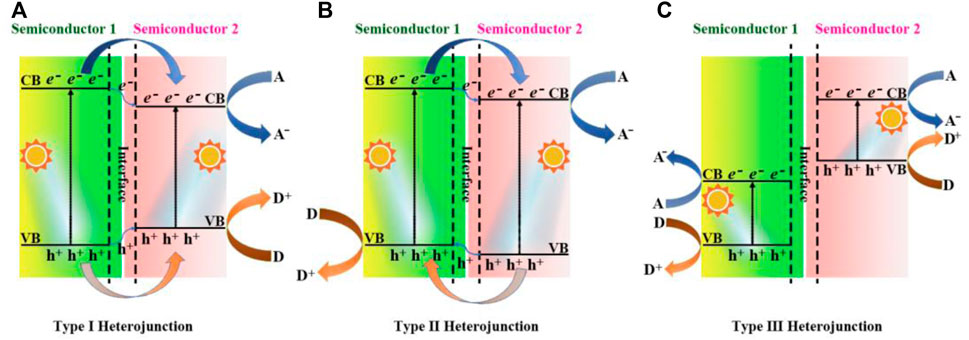
FIGURE 5. Schematic representation band structure of different heterojunctions: (A) Type Ⅰ heterojunction; (B) Type Ⅱ heterojunction; (C) Type Ⅲ heterojunction. A and D represent electron acceptor and electron donor, respectively.
Thus, a suitable semiconductor heterojunction is able to both enhance the ability to capture sunlight and significantly accelerate the separation and migration of photogenerated electron-hole pairs as description in Type II structure, but it is still insufficient in terms of photocatalytic oxidation ability. The Z-scheme heterojunction (Xu et al., 2022) was explored to overcome this disadvantage to a certain extent, which was mainly divided into binary and ternary structures, as shown in the Figure 6 (Maeda, 2013). The CB and VB potentials of semiconductor-2 were more positive than those of semiconductor-1 in binary Z-scheme (Figure 6A), thereby enhancing the reduction and oxidation capacity of e− and h+. Zhao et al. prepared the CeO2/g-C3N4 heterojunction photocatalysts, through a one-step in situ pyrolysis formation of 3D hollow CeO2 mesoporous nanospheres and 2D g-C3N4 nanosheets. The hydrogen evolution from water splitting experiment of the CeO2/g-C3N4-6 gave a maximum yield of 1,240.9 μmol g−1 h−1, which was about 5.2 times higher than that of CeO2 (Zhao et al., 2021). Figure 6B pictured out a conductor was employed as a charge bridge between the VB of semiconductor-1 and the CB of semiconductor-2 in the ternary Z-scheme heterojunction, which was played by metal particles, such as Cu, Au, Ag, etc. Hieu et al. synthesized the TiO2/Ti3C2/g-C3N4 (TTC) photocatalyst from g-C3N4 and Ti3C2 MXene via a calicination technique, and a high H2 production of 2,592 μmol g−1 was achieved (Hieu et al., 2021).
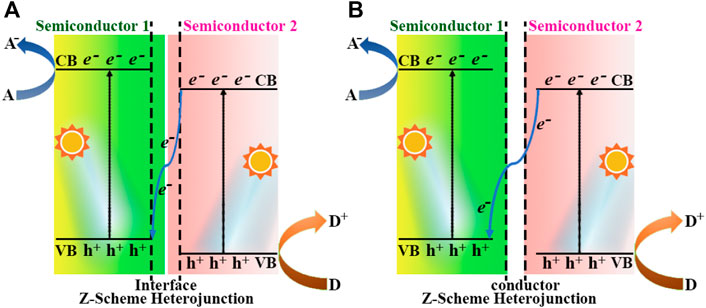
FIGURE 6. Schematic energy band diagram of two different types of Z-scheme heterojunction: (A) binary Z-scheme heterojunction, (B) ternary Z-scheme heterojunction. A and D denote electron acceptor and electron donor, respectively.
2.7 Homojunction structure
The g-C3N4 homojunctions are also recognized as the efficient photocatalysts. However, the type II structures and Z-schemes in the pioneering reports require deep optimization of the electron transport path in g-C3N4 homojunctions, since the redox potentials were depressed to inhibit the improvement of photocatalytic performance. The barrier could be overcome by the inspiration of S-scheme heterojunction proposed by Yu’s group (Xu et al., 2020), Guo et al. fabricated the S-scheme homojunctions with high-crystalline/amorphous g-C3N4 (HCCN/ACN) with solvothermal method, which was applied in photocatalytic H2 production with the evolution rates of 5.534 mmol h−1 g−1 in water and 3.147 mmol h−1 g−1 in seawater (Sun et al., 2022).
3 Conclusion
The excessive use and combustion of fossil fuels will inevitably bring some environmental problems. The value of hydrogen energy has been fully recognized, but its preparation technology still needs to be further explored. Photocatalytic technology is expected to realize sustainable energy production under the premise of making full use of solar energy, and has great potential in terms of energy and environment. The main factor limiting the photocatalytic activity of pristine g-C3N4 is its bulk structure, resulting in its small specific surface area and few active sites, which prolongs the transfer path of photogenerated electrons, thus accelerates the photogenerated charge carriers compound odds. The ability of photocatalytic hydrogen production performance of g-C3N4 could be improved by adjusting pH of the environment to induce the change of the surface charge of g-C3N4, controlling the morphology of g-C3N4 to increase active sites and shorten the transport path of carriers, and compositing co-catalysts or narrow-band semiconductors or dyes to enhance light absorption and reduce the recombination of photogenerated electrons and holes. So far, the strategies for exploration of stable hybridization structures to boost the photocatalytic efficiency could be the main concern in this filed, and more cases should be discovered to realize the dependence of the morphologies, structures, and species of dopants on the activities. This review is aimed at summarization of the recent progress of preparation and performance of g-C3N4-block photocatalysts to induce new ideas for the structural design with further improved efficiency by interdisciplinary researches across chemistry, physics, and material science.
Author contributions
R-HG, HC, and Y-QZ contributed to conception and design of the study. ML, QG, and NJ organized the database. R-HG wrote the first draft of the manuscript. All authors contributed to manuscript revision, read, and approved the submitted version.
Funding
This work was supported by Natural Science Foundation of Guizhou Province [No. ZK(2022)049], and National Natural Science Foundation of China (No. 22161011).
Conflict of interest
The authors declare that the research was conducted in the absence of any commercial or financial relationships that could be construed as a potential conflict of interest.
Publisher’s note
All claims expressed in this article are solely those of the authors and do not necessarily represent those of their affiliated organizations, or those of the publisher, the editors and the reviewers. Any product that may be evaluated in this article, or claim that may be made by its manufacturer, is not guaranteed or endorsed by the publisher.
Supplementary material
The Supplementary Material for this article can be found online at: https://www.frontiersin.org/articles/10.3389/fchem.2022.1048504/full#supplementary-material
References
Bai, X. J., Wang, L., Zong, R. L., and Zhu, Y. (2013). Photocatalytic activity enhanced via g-C3N4 nanoplates to nanorods. J. Phys. Chem. C 117 (19), 9952–9961. doi:10.1021/jp402062d
Bard, A. J., and Fox, M. A. (1995). Artificial photosynthesis: Solar splitting of water to hydrogen and oxygen. Acc. Chem. Res. 28 (3), 141–145. doi:10.1021/ar00051a007
Bashir, H., Yi, X. Y., Yuan, J. L., Yin, K., and Luo, S. (2019). Highly ordered TiO2 nanotube arrays embedded with g-C3N4 nanorods for enhanced photocatalytic activity. J. Photochem. Photobiol. A Chem. 382, 111930. doi:10.1016/j.jphotochem.2019.111930
Bi, L., Xu, D., Zhang, L., Lin, Y., Wang, D., and Xie, T. (2015). Metal Ni-loaded g-C3N4 for enhanced photocatalytic H2 evolution activity: The change in surface band bending. Phys. Chem. Chem. Phys. 17 (44), 29899–29905. doi:10.1039/c5cp05158d
Caux, M., Menard, H., AlSalik, Y. M., Irvine, J. T. S., and Idriss, H. (2019). Photo-catalytic hydrogen production over Au/g-C3N4: Effect of Gold Particle dispersion and morphology. Phys. Chem. Chem. Phys. 21 (29), 15974–15987. doi:10.1039/c9cp02241d
Chai, B., Liao, X., Song, F., and Zhou, H. (2014). Fullerene modified C3N4 composites with enhanced photocatalytic activity under visible light irradiation. Dalton Trans. 43 (3), 982–989. doi:10.1039/c3dt52454j
Chen, D., Wang, K., Hong, W., Zong, R., Yao, W., and Zhu, Y. (2015). Visible light photoactivity enhancement via CuTCPP hybridized g-C3N4 nanocomposite. Appl. Catal. B Environ. 166, 366–373. doi:10.1016/j.apcatb.2014.11.050
Chen, D., Wang, Z., Yue, D., Yang, G., Ren, T., and Ding, H. (2016). Synthesis and visible photodegradation enhancement of CdS/mpg-C3N4 photocatalyst. J. Nanosci. Nanotechnol. 16 (1), 471–479. doi:10.1166/jnn.2016.10661
Chen, H. B., and Liu, W. X. (2016). Cellulose-based photocatalytic paper with Ag2O nanoparticles loaded on graphite fibers. J. Bioresour. Bioprod. 1 (4), 192–198. doi:10.21967/jbb.v1i4.63
Chen, P. W., Li, K., Yu, Y. X., and Zhang, W. De. (2017). Cobalt-doped graphitic carbon nitride photocatalysts with high activity for hydrogen evolution. Appl. Surf. Sci. 392, 608–615. doi:10.1016/j.apsusc.2016.09.086
Chen, X., Shi, R., Chen, Q., Zhang, Z., Jiang, W., Zhu, Y., et al. (2019). Three-dimensional porous g-C3N4 for highly efficient photocatalytic overall water splitting. Nano Energy 59, 644–650. doi:10.1016/j.nanoen.2019.03.010
Chen, X., Wei, J., Hou, R., Liang, Y., Xie, Z., Zhu, Y., et al. (2016). Growth of g-C3N4 on mesoporous TiO2 spheres with high photocatalytic activity under visible light irradiation. Appl. Catal. B Environ. 188, 342–350. doi:10.1016/j.apcatb.2016.02.012
Chen, Z. H., Guo, F., Sun, H., Shi, Y. X., and Shi, W. L. (2022). Well-designed three-dimensional hierarchical hollow tubular g-C3N4/ZnIn2S4 nanosheets heterostructure for achieving efficient visible-light photocatalytic hydrogen evolution. J. Colloid Interface Sci. 607, 1391–1401. doi:10.1016/j.jcis.2021.09.095
Cui, L., Song, J., McGuire, A. F., Kang, S., Fang, X., Wang, J., et al. (2018). Constructing highly uniform onion-ring-like graphitic carbon nitride for efficient visible-light-driven photocatalytic hydrogen evolution. ACS Nano 12, 5551–5558. doi:10.1021/acsnano.8b01271
Dai, X., Jin, X. Y., Gao, R. H., Ge, Q., Chen, K., Jiang, N., et al. (2022). Controllable synthesis of Co nanoparticles with the assistance of cucurbit[6]uril and its efficient photoelectrochemical catalysis in water splitting on a g-C3N4 photoanode. New J. Chem. 46, 6738–6746. doi:10.1039/d2nj00036a
Deeksha, B., Sadanand, V., Hariram, N., and Rajulu, A. V. (2021). Preparation and properties of cellulose nanocomposite fabrics with in situ generated silver nanoparticles by bioreduction method. J. Bioresour. Bioprod. 6 (1), 75–81. doi:10.1016/j.jobab.2021.01.003
Di, J., Xiong, J., Li, H., and Liu, Z. (2018). Ultrathin 2D photocatalysts: Electronic-structure tailoring, hybridization, and applications. Adv. Mat. 30 (1), 1704548. doi:10.1002/adma.201704548
Fang, X. X., Ma, L. B., Liang, K., Zhao, S. J., Jiang, Y. F., Ling, C., et al. (2019). The Doping of phosphorus atoms into graphitic carbon nitride for highly enhanced photocatalytic hydrogen evolution. J. Mat. Chem. A Mat. 7 (18), 11506–11512. doi:10.1039/c9ta01646e
Fu, J., Yu, J., Jiang, C., and Cheng, B. (2018). g-C3N4-based heterostructured photocatalysts. Adv. Energy Mat. 8 (3), 1701503–1701531. doi:10.1002/aenm.201701503
Fujishima, A., and Honda, K. (1972). Electrochemical photolysis of water at a semiconductor electrode. Nature 238 (5358), 37–38. doi:10.1038/238037a0
Ge, L., and Han, C. (2012). Synthesis of MWNTs/g-C3N4 composite photocatalysts with efficient visible light photocatalytic hydrogen evolution activity. Appl. Catal. B Environ. 117, 268–274. doi:10.1016/j.apcatb.2012.01.021
Guo, F., Chen, Z., Huang, X., Cao, L., Cheng, X., Shi, W., et al. (2021). Cu3P nanoparticles decorated hollow tubular carbon nitride as a superior photocatalyst for photodegradation of tetracycline under visible light. Sep. Purif. Technol. 275 (15), 119223. doi:10.1016/j.seppur.2021.119223
Guo, S., Deng, Z., Li, M., Jiang, B., Tian, C., Pan, Q., et al. (2016). Phosphorus-doped carbon nitride tubes with a layered micro-nanostructure for enhanced visible-light photocatalytic hydrogen evolution. Angew. Chem. Int. Ed. 55 (5), 1830–1834. doi:10.1002/anie.201508505
Han, Q., Wang, B., Zhao, Y., Hu, C., and Qu, L. (2015). A graphitic-C3N4 “seaweed” architecture for enhanced hydrogen evolution. Angew. Chem. Int. Ed. 54 (39), 11433–11437. doi:10.1002/anie.201504985
Hieu, V. Q., Lam, T. C., Khan, A., Thi Vo, T., Nguyen, T. Q., Doan, V. D., et al. (2021). TiO2/Ti3C2/g-C3N4 ternary heterojunction for photocatalytic hydrogen evolution. Chemosphere 285, 131429. doi:10.1016/j.chemosphere.2021.131429
Indra, A., Menezes, P. W., Kailasam, K., Hollmann, D., Schroder, M., Thomas, A., et al. (2016). Nickel as a Co-catalyst for photocatalytic hydrogen evolution on graphitic-carbon nitride (sg-CN): What is the nature of the active species? Chem. Commun. 52 (1), 104–107. doi:10.1039/c5cc07936e
Kim, D., Sakimoto, K. K., Hong, D., and Yang, P. D. (2015). Artificial photosynthesis for sustainable fuel and chemical production. Angew. Chem. Int. Ed. 54 (11), 3259–3266. doi:10.1002/anie.201409116
Kong, L., Dong, Y., Jiang, P., Wang, G., Zhang, H., and Zhao, N. (2016). Light-assisted rapid preparation of a Ni/g-C3N4 magnetic composite for robust photocatalytic H2 evolution from water. J. Mat. Chem. A 4 (25), 9998–10007. doi:10.1039/c6ta03178a
Kudo, A., and Miseki, Y. (2009). Heterogeneous photocatalyst materials for water splitting. Chem. Soc. Rev. 38 (1), 253–278. doi:10.1039/b800489g
Li, C., Wang, S., Wang, T., Wei, Y., Zhang, P., and Gong, J. (2014). Monoclinic porous BiVO4 networks decorated by discrete g-C3N4 nano-islands with tunable coverage for highly efficient photocatalysis. Small 10 (14), 2783–2790. doi:10.1002/smll.201400506
Li, H. J., Qian, D. J., and Chen, M. (2015). Templateles infrared heating process for fabricating carbon nitride nanorods with efficient photocatalytic H2 evolution. ACS Appl. Mat. Interfaces 7 (45), 25162–25170. doi:10.1021/acsami.5b06627
Li, H., Li, N., Wang, M., Zhao, B., and Long, F. (2018). Synthesis of novel and stable g-C3N4-Bi2WO6 hybrid nanocomposites and their enhanced photocatalytic activity under visible light irradiation. R. Soc. open Sci. 5 (3), 171419. doi:10.1098/rsos.171419
Li, Q., Zhang, N., Yang, Y., Wang, G., and Ng, D. H. L. (2014). High efficiency photocatalysis for pollutant degradation with MoS2/C3N4 heterostructures. Langmuir 30 (29), 8965–8972. doi:10.1021/la502033t
Li, X., Yu, J., and Jaroniec, M. (2016). Hierarchical photocatalysts. Chem. Soc. Rev. 45 (9), 2603–2636. doi:10.1039/c5cs00838g
Liang, S., Zhang, D., Pu, X., Yao, X., Han, R., Yin, J., et al. (2019). A novel Ag2O/g-C3N4 p-n heterojunction photocatalysts with enhanced visible and near-infrared light activity. Sep. Purif. Technol. 210, 786–797. doi:10.1016/j.seppur.2018.09.008
Lin, Z., and Wang, X. (2014). Ionic liquid promoted synthesis of conjugated carbon nitride photocatalysts from urea. ChemSusChem 7 (6), 1547–1550. doi:10.1002/cssc.201400016
Liu, E., Lin, X., Hong, Y., Yang, L., Luo, B., Shi, W., et al. (2021). Rational copolymerization strategy engineered C self-doped g-C3N4 for efficient and robust solar photocatalytic H2 evolution. Renew. Energy 178, 757–765. doi:10.1016/j.renene.2021.06.066
Liu, L., Qi, Y., Lu, J., Lin, S., An, W., Liang, Y., et al. (2016). A stable Ag3PO4@g-C3N4 hybrid core@shell composite with enhanced visible light photocatalytic degradation. Appl. Catal. B Environ. 183, 133–141. doi:10.1016/j.apcatb.2015.10.035
Liu, Q., Li, N., Qiao, Z., Li, W., Wang, L., Zhu, S., et al. (2019). The multiple promotion effects of ammonium phosphate-modified Ag3PO4 on photocatalytic performance. Front. Chem. 7, 866. doi:10.3389/fchem.2019.00866
Liu, S., Zada, A., Yu, X., Liu, F., and Jin, G. (2022). NiFe2O4/g-C3N4 heterostructure with an enhanced ability for photocatalytic degradation of tetracycline hydrochloride and antibacterial performance. Chemosphere 307, 135717. doi:10.1016/j.chemosphere.2022.135717
Liu, Y., Kang, S., Cui, L., and Ma, Z. (2020). Boosting near-infrared-driven photocatalytic H2 evolution using protoporphyrin-sensitized g-C3N4. J. Photochem. Photobiol. A Chem. 396, 112517–112526. doi:10.1016/j.jphotochem.2020.112517
Maeda, K., Teramura, K., Lu, D., Takata, T., Saito, N., Inoue, Y., et al. (2006). Photocatalyst releasing hydrogen from water. Nature 440 (7082), 295. doi:10.1038/440295a
Maeda, K. (2013). Z-scheme water splitting using two different semiconductor photocatalysts. ACS Catal. 3 (7), 1486–1503. doi:10.1021/cs4002089
Min, S., and Lu, G. (2012). Enhanced electron transfer from the excited eosin Y to mpg-C3N4 for highly efficient hydrogen evolution under 550 nm irradiation. J. Phys. Chem. C 116 (37), 19644–19652. doi:10.1021/jp304022f
Mo, Z., Xu, H., Chen, Z. G., She, X., Song, Y., Wu, J., et al. (2018). Self-assembled synthesis of defect-engineered graphitic carbon nitride nanotubes for efficient conversion of solar energy. Appl. Catal. B Environ. 225, 154–161. doi:10.1016/j.apcatb.2017.11.041
Nagajyothi, P. C., Pandurangan, M., Vattikuti, S. V. P., Tettey, C. O., Sreekanth, T. V. M., and Shim, J. (2017). Enhanced photocatalytic activity of Ag/g-C3N4 composite. Sep. Purif. Technol. 188, 228–237. doi:10.1016/j.seppur.2017.07.026
Nagaraja, C. M., Kaur, M., and Dhingra, S. (2020). Enhanced visible-light-assisted photocatalytic hydrogen generation by MoS2/g-C3N4 nanocomposites. Int. J. Hydrogen Energy 45 (15), 8497–8506. doi:10.1016/j.ijhydene.2020.01.042
Naseri, A., Samadi, M., Pourjavadi, A., Moshfegh, A. Z., and Ramakrishma, S. (2017). Graphitic carbon nitride (g-C3N4)-based photocatalysts for solar hydrogen generation: Recent advances and future development directions. J. Mat. Chem. A Mat. 5 (45), 23406–23433. doi:10.1039/c7ta05131j
Niu, P., Zhang, L., Liu, G., and Cheng, H. M. (2012). Graphene-like carbon nitride nanosheets for improved photocatalytic activities. Adv. Funct. Mat. 22 (22), 4763–4770. doi:10.1002/adfm.201200922
Ong, W. J., Tan, L. L., Ng, Y. H., Yong, S. T., and Chai, S. P. (2016). Graphitic carbon nitride (g-C3N4)-based photocatalysts for artificial photosynthesis and environmental remediation: Are we a step closer to achieving sustainability? Chem. Rev. 116 (12), 7159–7329. doi:10.1021/acs.chemrev.6b00075
Ou, M., Wan, S., Zhong, Q., Zhang, S., and Wang, Y. (2017). Single Pt atoms deposition on g-C3N4 nanosheets for photocatalytic H2 evolution or NO oxidation under visible light. Int. J. Hydrogen Energy 42 (44), 27043–27054. doi:10.1016/j.ijhydene.2017.09.047
Patnaik, S., Martha, S., and Parida, K. M. (2016). An overview of the structural, textural and morphological modulations of g-C3N4 towards photocatalytic hydrogen production. RSC Adv. 6 (52), 46929–46951. doi:10.1039/c5ra26702a
Qi, K., Cui, N., Zhang, M., Ma, Y., Wang, G., Zhao, Z., et al. (2021). Ionic liquid-assisted synthesis of porous boron-doped graphitic carbon nitride for photocatalytic hydrogen production. Chemosphere 272, 129953. doi:10.1016/j.chemosphere.2021.129953
Qi, K., Li, Y., Xie, Y., Liu, S., Zheng, K., Chen, Z., et al. (2019). Ag loading enhanced photocatalytic activity of g-C3N4 porous nanosheets for decomposition of organic pollutants. Front. Chem. 7, 91. doi:10.3389/fchem.2019.00091
Qi, K., Liu, S., and Zada, A. (2020). Graphitic carbon nitride, a polymer photocatalyst. J. Taiwan Inst. Chem. Eng. 109, 111–123. doi:10.1016/j.jtice.2020.02.012
Qi, K., Lv, W., Khan, I., and Liu, S. (2020). Photocatalytic H2 generation via CoP quantum-dot-modified g-C3N4 synthesized by electroless plating. Chin. J. Catal. 41 (1), 114–121. doi:10.1016/s1872-2067(19)63459-5
Qi, K., Zhang, C., Zhang, M., Gholami, P., and Khataee, A. (2022). Sonochemical synthesis of photocatalysts and their applications. J. Mat. Sci. Technol. 123, 243–256. doi:10.1016/j.jmst.2022.02.019
Qi, Y., Xu, J., Zhang, M., Lin, H., and Wang, L. (2019). In situ metal-organic framework-derived C-doped Ni3S4/Ni2P hybrid co-catalysts for photocatalytic H2 production over g-C3N4 via dye sensitization. Int. J. Hydrogen Energy 44 (31), 16336–16347. doi:10.1016/j.ijhydene.2019.04.276
Ran, J., Ma, T. Y., Gao, G., Du, X. W., and Qiao, S. Z. (2015). Porous P-doped graphitic carbon nitride nanosheets for synergistically enhanced visible-light photocatalytic H2 production. Energy Environ. Sci. 8 (12), 3708–3717. doi:10.1039/c5ee02650d
Reza, G. M., Dinh, C. T., Beland, F., and Do, T. O. (2015). Nanocomposite heterojunctions as sunlight-driven photocatalysts for hydrogen production from water splitting. Nanoscale 7 (18), 8187–8208. doi:10.1039/c4nr07224c
Samanta, S., Martha, S., and Parida, K. (2014). Facile synthesis of Au/g-C3N4 nanocomposites: An inorganic/organic hybrid plasmonic photocatalyst with enhanced hydrogen gas evolution under visible-light irradiation. ChemCatChem 6 (5), 1453–1462. doi:10.1002/cctc.201300949
Schwinghanmmer, K., Tuffy, B., Mesch, M. B., Wirnhier, E., Martineau, C., Taulelle, F., et al. (2013). Triazine-based carbon nitrides for visible-light-driven hydrogen evolution. Angew. Chem. Int. Ed. 52 (9), 2435–2439. doi:10.1002/anie.201206817
Shen, X. C., Tang, Y. J., Zhou, D. D., Zhang, J. H., Guo, D. L., and Friederichs, G. (2016). Improving the electroconductivity and mechanical properties of cellulosic paper with multi-walled carbon nanotube/polyaniline nanocomposites. J. Bioresour. Bioprod. 1 (1), 48–54. doi:10.21967/jbb.v1i1.40
Shi, W. L., Sun, W., Liu, Y., Li, X. Y., Lin, X., Guo, F., et al. (2022). Onion -ring-like g-C3N4 modified with Bi3TaO7 quantum dots: A novel 0D/3D S-scheme heterojunction for enhanced photocatalytic hydrogen production under visible light irradiation. Renew. Energy 182, 958–968. doi:10.1016/j.renene.2021.11.030
Shi, Y., Jiang, S., Zhou, K., Wang, B., Wang, B., Gui, Z., et al. (2014). Facile Preparation of ZnS/g-C3N4 nanohybrids for enhanced optical properties. RSC Adv. 4 (6), 2609–2613. doi:10.1039/c3ra44256j
Shi, Y. X., Li, L. L., Sun, H. R., Xu, Z., Cai, Y., Shi, W. L., et al. (2022). Engineering ultrathin oxygen-doped g-C3N4 nanosheet for boosted photoredox catalytic activity based on a facile thermal gas-shocking exfoliation effect. Sep. Purif. Technol. 292, 121038. doi:10.1016/j.seppur.2022.121038
Sun, H., R., Wang, L. J., Guo, F., Shi, Y. X., Li, L. L., Xu, Z., et al. (2022a). Fe-doped g-C3N4 derived from biowaste material with Fe-N bonds for enhanced synergistic effect between photocatalysis and Fenton degradation activity in a broad pH range. J. Alloys Compd. 900, 163410. doi:10.1016/j.jallcom.2021.163410
Sun, H. R., Shi, Y. X., Shi, W. L., and Guo, F. (2022b). High-crystalline/amorphous g-C3N4 S-scheme homojunction for boosted photocatalytic H2 production in water/simulated seawater: Interfacial charge transfer and mechanism insight. Appl. Surf. Sci. 593, 153281. doi:10.1016/j.apsusc.2022.153281
Sun, J. X., Yuan, Y. P., Qiu, L. G., Jiang, X., Xie, A. J., Shen, Y. H., et al. (2012). Fabrication of composite photocatalyst g-C3N4-ZnO and enhancement of photocatalytic activity under visible light. Dalton Trans. 41 (22), 6756–6763. doi:10.1039/c2dt12474b
Sun, L., Qi, Y., Jia, C. J., Jin, Z., and Fan, W. (2014). Enhanced visible-light photocatalytic activity of g-C3N4/Zn2GeO4 heterojunctions with effective interfaces based on band match. Nanoscale 6 (5), 2649–2659. doi:10.1039/c3nr06104c
Sun, X. H., Shi, Y. X., Lu, J. L., Shi, W. L., and Guo, F. (2022). Template-free self-assembly of three-dimensional porous graphitic carbon nitride nanovesicles with size-dependent photocatalytic activity for hydrogen evolution. Appl. Surf. Sci. 606, 154841. doi:10.1016/j.apsusc.2022.154841
Theerthagiri, J., Senthil, R. A., Priya, A., Madhavan, J., Michael, R. J. V., and Ashokkumar, M. (2014). Photocatalytic and photoelectrochemical studies of visible-light active alpha-Fe2O3-g-C3N4 nanocomposites. RSC Adv. 4 (72), 38222–38229. doi:10.1039/c4ra04266b
Thomas, A., Fischer, A., Goettmann, F., Antonietti, M., Muller, J. O., Schlogl, R., et al. (2008). Graphitic carbon nitride materials: Variation of structure and morphology and their use as metal-free catalysts. J. Mat. Chem. 18 (41), 4893–4908. doi:10.1039/b800274f
Wang, B., Wang, Y., Lei, Y., Wu, N., Gou, Y., Han, C., et al. (2016). Mesoporous silicon carbide nanofibers with in situ embedded carbon for Co-catalyst free photocatalytic hydrogen production. Nano Res. 9 (3), 886–898. doi:10.1007/s12274-015-0971-z
Wang, L. X., Zhao, F., Han, Q., Hu, C., Lv, L., Chen, N., et al. (2015). Spontaneous formation of Cu2O-g-C3N4 core-shell nanowires for photocurrent and humidity responses. Nanoscale 7 (21), 9694–9702. doi:10.1039/c5nr01521a
Wang, P., Guan, Z., Li, Q., and Yang, J. (2018). Efficient visible-light-driven photocatalytic hydrogen production from water by using Eosin Y-sensitized novel g-C3N4/Pt/GO composites. J. Mat. Sci. 53 (1), 774–786. doi:10.1007/s10853-017-1540-5
Wang, Q., Hisatomi, T., Jia, Q., Tokudome, H., Zhao, M., Wang, C., et al. (2016). Scalable water splitting on particulate photocatalyst sheets with a solar-to-hydrogen energy conversion efficiency exceeding 1%. Nat. Mat. 15 (6), 611–615. doi:10.1038/nmat4589
Wang, W., Yu, J. C., Shen, Z., Chan, D. K. L., and Gu, T. (2014). g-C3N4 quantum dots: direct synthesis, upconversion properties and photocatalytic application. Chem. Commun. 50 (70), 10148–10150. doi:10.1039/c4cc02543a
Wang, X., Maeda, K., Thomas, A., Takanabe, K., Xin, G., Carlsson, J. M., et al. (2009). A metal-free polymeric photocatalyst for hydrogen production from water under visible light. Nat. Mat. 8 (1), 76–80. doi:10.1038/nmat2317
Wang, Y., Hong, J., Zhang, W., and Xu, R. (2013). Carbon nitride nanosheets for photocatalytic hydrogen evolution: Remarkably enhanced activity by dye sensitization. Catal. Sci. Technol. 3 (7), 1703–1711. doi:10.1039/c3cy20836b
Wang, Z., Xu, X., Si, Z., Liu, L., He, Y., Ran, R., et al. (2018). In situ synthesized MoS2/Ag dots/Ag3PO4 Z-scheme photocatalysts with ultrahigh activity for oxygen evolution under visible light irradiation. Appl. Surf. Sci. 450, 441–450. doi:10.1016/j.apsusc.2018.04.149
Wu, P., Wang, J., Zhao, J., Guo, L., and Osterloh, F. E. (2014). High alkalinity boosts visible light driven H2 evolution activity of g-C3N4 in aqueous methanol. Chem. Commun. 50 (98), 15521–15524. doi:10.1039/c4cc08063g
Wu, X., Zhu, C., Wang, L., Guo, S., Zhang, Y., Li, H., et al. (2017). Control strategy on two-/four-electron pathway of water splitting by multidoped carbon based catalysts. ACS Catal. 7 (3), 1637–1645. doi:10.1021/acscatal.6b03244
Xiang, Q., Yu, J., and Jaroniec, M. (2011). Preparation and enhanced visible-light photocatalytic H2 production activity of graphene/C3N4 composites. J. Phys. Chem. C 115 (15), 7355–7363. doi:10.1021/jp200953k
Xiao, N., Li, Y., Li, X., Gao, Y., Ge, L., Lu, G., et al. (2019). In-situ synthesis of PdAg/g-C3N4 composite photocatalyst for highly efficient photocatalytic H2 generation under visible light irradiation. Int. J. Hydrogen Energy 44 (36), 19929–19941. doi:10.1016/j.ijhydene.2019.05.236
Xu, J., Qi, Y., Wang, W., and Wang, L. (2019). Montmorillonite-hybridized g-C3N4 composite modified by NiCoP cocatalyst for efficient visible-light-driven photocatalytic hydrogen evolution by dye-sensitization. Int. J. Hydrogen Energy 44 (8), 4114–4122. doi:10.1016/j.ijhydene.2018.12.167
Xu, Q., Zhang, L., Cheng, B., Fan, J., and Yu, J. (2020). S-scheme heterojunction photocatalyst. Chem 6 (7), 1543–1559. doi:10.1016/j.chempr.2020.06.010
Xu, X., Liu, G., Randorn, C., and Irvine, J. T. S. (2011). g-C3N4 coated SrTiO3 as an efficient photocatalyst for H2 production in aqueous solution under visible light irradiation. Int. J. Hydrogen Energy 36 (21), 13501–13507. doi:10.1016/j.ijhydene.2011.08.052
Xu, Z., Shi, Y. X., Li, L. L., Sun, H., R., Amin, M. D. S., Guo, F., et al. (2022). Fabrication of 2D/2D Z-scheme highly crystalline carbon nitride/δ-Bi2O3 heterojunction photocatalyst with enhanced photocatalytic degradation of tetracycline. J. Alloys Compd. 895, 162667. doi:10.1016/j.jallcom.2021.162667
Yu, L., Zhang, X., Zhuang, C., Lin, L., Li, R., and Peng, T. (2014). Syntheses of asymmetric zinc phthalocyanines as sensitizer of Pt-loaded graphitic carbon nitride for efficient visible/near-IR-light-driven H2 production. Phys. Chem. Chem. Phys. 16 (9), 4106–4114. doi:10.1039/c3cp54316a
Yue, B., Li, Q., Iwai, H., Kako, T., and Ye, J. (2011). Hydrogen production using zinc-doped carbon nitride catalyst irradiated with visible light. Sci. Technol. Adv. Mat. 12 (3), 034401034401–034401/7. doi:10.1088/1468-6996/12/3/034401
Zada, A., Khan, M., Qureshi, M. N., Liu, S. Y., and Wang, R. D. (2019). Accelerating photocatalytic hydrogen production and pollutant degradation by functionalizing g-C3N4 with SnO2. Front. Chem. 7, 941. doi:10.3389/fchem.2019.00941
Zeng, Y., Liu, X., Liu, C., Wang, L., Xia, Y., Zhang, S., et al. (2018). Scalable one-step production of porous oxygen-doped g-C3N4 nanorods with effective electron separation for excellent visible-light photocatalytic activity. Appl. Catal. B Environ. 224, 1–9. doi:10.1016/j.apcatb.2017.10.042
Zhang, G., Zhang, M., Ye, X., Qiu, X., Lin, S., and Wang, X. (2014). Iodine modified carbon nitride semiconductors as visible light photocatalysts for hydrogen evolution. Adv. Mat. 26 (5), 805–809. doi:10.1002/adma.201303611
Zhang, J. Y., Wang, Y. H., Jin, J., Lin, Z., Huang, F., Yu, J. G., et al. (2013). Efficient visible-light photocatalytic hydrogen evolution and enhanced photostability of core/shell CdS/g-C3N4 nanowires. ACS Appl. Mat. Interfaces 5 (20), 10317–10324. doi:10.1021/am403327g
Zhang, P., Song, T., Wang, T., and Zeng, H. (2017). Effectively extending visible light absorption with a broad spectrum sensitizer for improving the H2 evolution of in-situ Gu/g-C3N4 nanocomponents. Int. J. Hydrogen Energy 42 (21), 14511–14521. doi:10.1016/j.ijhydene.2017.04.234
Zhang, P., Wang, T., and Zeng, H. (2017). Design of Cu-Cu2O/g-C3N4 nanocomponent photocatalysts for hydrogen evolution under visible light irradiation using water-soluble Erythrosin B dye sensitization. Appl. Surf. Sci. 391, 404–414. doi:10.1016/j.apsusc.2016.05.162
Zhang, S., Khan, I., Qin, X., Qi, K., Liu, Y., and Bai, S. (2020). Construction of 1D Ag-AgBr/AlOOH plasmonic photocatalyst for degradation of tetracycline hydrochloride. Front. Chem. 8, 117. doi:10.3389/fchem.2020.00117
Zhang, X., Peng, B., Zhang, S., and Peng, T. (2015). Robust wide visible-light-responsive photoactivity for H2 production over a polymer/polymer heterojunction photocatalyst: The significance of sacrificial reagent. ACS Sustain. Chem. Eng. 3 (7), 1501–1509. doi:10.1021/acssuschemeng.5b00211
Zhang, X., Peng, T., Yu, L., Li, R., Li, Q., and Li, Z. (2015). Visible/near-infrared-light-induced H2 production over g-C3N4 Co-sensitized by organic dye and zinc phthalocyanine derivative. ACS Catal. 5 (2), 504–510. doi:10.1021/cs5016468
Zhang, X. W., Zhu, Y. F., Chen, X. Q., Shen, W. H., and Lutes, R. (2018). How does binuclear zinc amidohydrolase FwdA work in the initial step of methanogenesis: From formate to formyl-methanofuran. J. Inorg. Biochem. 3 (2), 71–79. doi:10.1016/j.jinorgbio.2018.05.004
Zhang, X., Yu, L., Zhuang, C., Peng, T., Li, R., and Li, X. (2014). Highly asymmetric phthalocyanine as a sensitizer of graphitic carbon nitride for extremely efficient photocatalytic H2 production under near-infrared light. ACS Catal. 4 (1), 162–170. doi:10.1021/cs400863c
Zhang, Y., Liu, J., Wu, G., and Chen, W. (2012). Porous graphitic carbon nitride synthesized via direct polymerization of urea for efficient sunlight-driven photocatalytic hydrogen production. Nanoscale 4 (17), 5300–5303. doi:10.1039/c2nr30948c
Zhao, S., Xu, J., Mao, M., Li, L., and Li, X. (2021). Protonated g-C3N4 cooperated with Co-MOF doped with Sm to construct 2D/2D heterojunction for integrated dye-sensitized photocatalytic H2 evolution. J. Colloid Interface Sci. 583, 435–447. doi:10.1016/j.jcis.2020.09.063
Zhao, S., Zhang, Y. W., Zhou, Y. M., Wang, Y., Qiu, K., Zhang, C., et al. (2018). Facile one-step synthesis of hollow mesoporous g-C3N4 spheres with ultrathin nanosheets for photoredox water splitting. Carbon 126, 247–256. doi:10.1016/j.carbon.2017.10.033
Zhao, W., She, T., Zhang, J., Wang, G., Zhang, S., Wei, W., et al. (2021). A novel z-scheme CeO2/g-C3N4 heterojunction photocatalyst for degradation of bisphenol A and hydrogen evolution and insight of the photocatalysis mechanism. J. Mater. Sci. Technol. 85, 18–29. doi:10.1016/j.jmst.2020.12.064
Zhao, Y., Zada, A., Yang, Y., Pan, J., Wang, Y., Yan, Z., et al. (2021). Photocatalytic removal of antibiotics on g-C3N4 using amorphous CuO as cocatalysts. Front. Chem. 9, 797738. doi:10.3389/fchem.2021.797738
Zhong, X., Wang, G., Papandrea, B., Li, M., Xu, Y., Chen, Y., et al. (2015). Reduced graphene oxide/silicon nanowire heterostructures with enhanced photoactivity and superior photoelectrochemical stability. Nano Res. 8 (9), 2850–2858. doi:10.1007/s12274-015-0790-2
Zhu, T., Song, Y., Ji, H., Xu, Y., Song, Y., Xia, J., et al. (2015). Synthesis of g-C3N4/Ag3VO4 composites with enhanced photocatalytic activity under visible light irradiation. Chem. Eng. J. 271, 96–105. doi:10.1016/j.cej.2015.02.018
Zhu, Y., Wang, T., Xu, T., Li, Y., and Wang, C. (2019). Size effect of Pt co-catalyst on photocatalytic efficiency of g-C3N4 for hydrogen evolution. Appl. Surf. Sci. 464, 36–42. doi:10.1016/j.apsusc.2018.09.061
Zhuang, J., Zhang, J., Pang, J., Wang, A., Wang, X., and Zhu, W. (2019). Fabrication of pyrimidine/g-C3N4 nanocomposites for efficient photocatalytic activity under visible-light illumination. Dyes Pigments 163, 634–640. doi:10.1016/j.dyepig.2018.12.046
Keywords: g-C3N4, photocatalysis, hydrogen evolution, energy materials, semiconductor
Citation: Gao R-H, Ge Q, Jiang N, Cong H, Liu M and Zhang Y-Q (2022) Graphitic carbon nitride (g-C3N4)-based photocatalytic materials for hydrogen evolution. Front. Chem. 10:1048504. doi: 10.3389/fchem.2022.1048504
Received: 19 September 2022; Accepted: 14 October 2022;
Published: 25 October 2022.
Edited by:
Kezhen Qi, Shenyang Normal University, ChinaReviewed by:
Xin Ying Kong, Nanyang Technological University, SingaporeFeng Guo, Jiangsu University of Science and Technology, China
Copyright © 2022 Gao, Ge, Jiang, Cong, Liu and Zhang. This is an open-access article distributed under the terms of the Creative Commons Attribution License (CC BY). The use, distribution or reproduction in other forums is permitted, provided the original author(s) and the copyright owner(s) are credited and that the original publication in this journal is cited, in accordance with accepted academic practice. No use, distribution or reproduction is permitted which does not comply with these terms.
*Correspondence: Hang Cong, ZWNudWNAMTYzLmNvbQ==; Yun-Qian Zhang, c2NpLnlxemhhbmdAZ3p1LmVkdS5jbg==