- 1Institute for Nuclear Waste Disposal, Karlsruhe Institute of Technology, Karlsruhe, Germany
- 2Research Centre for Radwaste Disposal and Williamson Research Centre for Molecular Environmental Science, Department of Earth and Environmental Sciences, The University of Manchester, Manchester, United Kingdom
The impact of temperature on a freshly precipitated ThO2(am, hyd) solid phase was investigated using a combination of undersaturation solubility experiments and a multi-method approach for the characterization of the solid phase. XRD and EXAFS confirm that ageing of ThO2(am, hyd) at T = 80°C promotes a significant increase of the particle size and crystallinity. TG-DTA and XPS support that the ageing process is accompanied by an important decrease in the number of hydration waters/hydroxide groups in the original amorphous Th(IV) hydrous oxide. However, while clear differences between the structure of freshly precipitated ThO2(am, hyd) and aged samples were observed, the characterization methods used in this work are unable to resolve clear differences between solid phases aged for different time periods or at different pH values. Solubility experiments conducted at T = 22°C with fresh and aged Th(IV) solid phases show a systematic decrease in the solubility of the solid phases aged at T = 80°C. In contrast to the observations gained by solid phase characterization, the ageing time and ageing pH significantly affect the solubility measured at T = 22°C. These observations can be consistently explained considering a solubility control by the outermost surface of the ThO2(s, hyd) solid, which cannot be properly probed by any of the techniques considered in this work. Solubility data are used to derive the thermodynamic properties (log *K°s,0, ΔfG°m) of the investigated solid phases, and discussed in terms of particle size using the Schindler equation. These results provide new insights on the interlink between solubility, structure, surface and thermodynamics in the ThO2(s, hyd)–H2O(l) system, with special emphasis on the transformation of the amorphous hydrous/hydroxide solid phases into the thermodynamically stable crystalline oxides.
1 Introduction
In the case of early canister failure, actinide chemistry in the near-field of a repository for the disposal of high-level radioactive waste (HLW) will be affected by elevated temperatures of up to 200°C, depending upon host-rock system and repository concept. The corrosion of iron occurring after the closure of the repository and possible water access will promote reducing conditions, for which the oxidation states + III and +IV are expected to control the solution chemistry of the actinides, An (Grenthe et al., 2020). In aqueous systems, An (IV) behavior is dominated by the formation of sparingly soluble, nanoparticulate and amorphous hydrous oxides, AnO2 (am, hyd), and by a strong tendency for hydrolysis (Rand et al., 2009; Grenthe et al., 2020). The transition of these amorphous oxy-hydroxides into the thermodynamically stable crystalline oxides AnO2 (cr), is kinetically hindered due to their low solubility, and is generally not observed in aqueous systems. However, ageing processes induced by time or temperature may facilitate this transition with the consequent decrease of the overall solubility.
The solubility and hydrolysis of thorium have been extensively investigated in the literature, although solubility studies involving a concurrent, thorough solid phase characterization are sparse. Hietanen and co-workers conducted potentiometric and coulometric experiments with Th(IV) in 3 M NaCl solutions at 25°C (Hietanen and Sillen, 1968). Based on their own experimental data and the reinterpretation of previous studies (Hietanen, 1954; Kraus and Holmberg, 1954; Baes et al., 1965), the authors derived a speciation model involving the predominance of the polynuclear species Th2(OH)26+, Th2(OH)35+ and Th6(OH)1410+. This chemical model was updated in the review work by Baes and Mesmer, who reported the hydrolysis scheme and corresponding equilibrium constants in the reference state (I = 0, T = 25°C) including the hydrolysis species ThOH3+, Th(OH)22+, Th(OH)4 (aq), Th2(OH)26+, Th4(OH)88+ and Th6(OH)159+ (Baes and Mesmer, 1976). In 2001, Neck and Kim published the most comprehensive study on An (IV) solubility and hydrolysis, which included the critical review of previously reported studies and estimations of hydrolysis constants based on a semi-empirical electrostatic model. For Th(IV), the authors selected a hydrolysis scheme including the species ThOH3+, Th(OH)22+, Th(OH)3+, Th(OH)4 (aq), Th4(OH)124+ and Th6(OH)159+, and reported the solubility product log K°s,0 (Th(OH)4, am) = –47.0 (Neck and Kim, 2001). Neck and co-workers reported also solubility experiments combined with laser-induced breakdown detection (LIBD) and X-ray absorption fine structure (XAFS) (Neck et al., 2002). The solubility product was determined to be log K°s,0 = –47.8, whereas XAFS and LIBD confirmed the presence of large amounts of small Th(IV) colloids within 3.5 < pH < 5. Altmaier et al. investigated the solubility and the colloid formation of Th(IV) in 0.5 M and 5.0 M NaCl as well as in 0.25, 2.5 and 4.5 M MgCl2 solutions (Altmaier et al., 2004). The authors emphasized the relevant role of intrinsic colloids in the aquatic chemistry of thorium in neutral and alkaline solutions. Kobayashi et al. conducted solubility experiments and investigated the differences on solid phases and solubility after aging a freshly precipitated Th(IV) hydroxide at 363 K for 3–6 weeks (Kobayashi et al., 2016). The authors observed a significant decrease on the solubility correlating with a slight growth of the particle size in the aged solid, for which a log K°s,0 = –51.6 was reported. Nishikawa et al. investigated the solubility of Th(IV) in 0.5 M NaClO4 and HClO4 solutions with 2.0 < pH < 8.0 (Nishikawa et al., 2018). The starting material Th(OH)4(am) was aged at 298 K, 313 K and 333 K for up to 40 weeks. Solubility measurements conducted with the aged solid phases were also conducted at 298, 313 and 333 K, and showed a systematic decrease of solubility with increase of the ageing temperature. The expected increase of crystallinity at elevated temperatures was confirmed by XRD measurements.
Most of the available solubility studies were conducted using amorphous hydrous oxides. The nomenclature used to define such solid phases includes Th(OH)4(am), ThO2⋅xH2O(am), ThO2(am, hyd), ThO2(am), among others, thus highlighting the ill-defined character of the solid phase controlling the solubility in these studies (Dzimitrowicz et al., 1985; Rai et al., 1997; Neck and Kim, 2001; Altmaier et al., 2004; Rand et al., 2009). Although the crystalline oxides AnO2(cr) are the thermodynamically stable An(IV) end-members (Rand et al., 2009; Grenthe et al., 2020), the amorphous hydrous oxides are actually controlling An(IV) solubility in aqueous systems and thus are considered to estimate solubility upper limits required in the safety assessment of repositories for nuclear waste disposal. The transition of An(IV) amorphous solid/colloidal phases into the thermodynamically stable AnO2(cr) has been correlated with the increase of the particle-size (Neck et al., 2007). However, no attempts have been made so far to link the loss of hydroxide groups/water in the transformation of AnO2(am, hyd) into AnO2(cr), or to understand how this affects the stability of the corresponding solid phases, as well as the interlink with surface area and particle size.
The structures of ThO2(am, hyd) nanoparticles and precursors, and AnO2(am, hyd) more generally, have been extensively investigated using extended X-ray absorption fine structure (EXAFS) analysis. Th coordination environments found varied from aqueous Th4+-like coordination for early hydrolysis products, with Th coordinated by 10–13 O backscatterers (likely H2O and OH−) at 2.44–2.51 Å and few or no Th backscatterers (Neck et al., 2002; Rothe et al., 2002), to more crystalline ThO2-like systems for heat treated nanoparticles, with seven to nine O backscatterers at 2.41 Å and a Th shell fit at 3.9–4.0 Å with up to 12 Th backscatterers (Bonato et al., 2020; Manaud et al., 2020). Rothe et al. analysed Th particles formed via hydrolysis and precipitation of colloids at pH 1.3–3.6 (Rothe et al., 2002). The resulting EXAFS fitting for the majority of samples indicated a coordination environment similar to that of aqueous Th, with only the highest pH sample and the precipitated ‘fresh’ ThO2(am,hyd) showing evidence of Th-Th backscatterers and long-range order. This is consistent with the findings of Neck et al. who, using a combination of EXAFS and laser-induced breakdown detection (LIBD), showed the structure of Th colloids formed after titration of acidic solution had high Th-O and low Th-Th coordination numbers relative to crystalline ThO2 (Neck et al., 2002). More recently, EXAFS studies into the structure of nanoparticulate ThO2 and PuO2 have shown that the nearest neighbor O shell alters significantly for very small particles (<10 nm) (Bonato et al., 2020). This trend was quantified by the Debye-Waller factor of the first Th-O shell, which was observed to decrease with increasing particle size (attained by TEM) and attributed to structural disorder which was more prevalent at smaller nanoparticle sizes. There was also a direct correlation observed between particle size and Th-Th coordination number; the larger the particle size, the higher the Th-Th coordination. This trend increased most significantly at small particle sizes and appeared to approach full Th-Th coordination of ThO2 with particles >25 nm in size. Other investigations into thermally synthesized ThO2 nanoparticles treated at 220-250°C found that the nanoparticles were highly crystalline and there was little or no variation in particle structure at different formation temperatures (Manaud et al., 2020). An XRD and XAFS study into ThO2 nanoparticles of sizes from 2.5 to 33.8 nm found that, with decreasing particle size, there was a systematic shift to larger lattice parameters by 1.1%, which coincided with a decrease in Th-Th coordination number, consistent with other studies discussed here (Plakhova et al., 2019). Amidani and co-workers investigated ThO2 nanoparticles by means of HEXS and HERFD XANES (Amidani et al., 2021). The authors observed mixed thorium hexamer clusters with 1 nm nanoparticles in the initial steps of formation, which lead to more crystalline, thermodynamically stable nanoparticles when exposed to elevated temperatures (150–1,000 °C). Recently, Romanchuk et al. compiled a systematic study of AnO2 (An = Th, U, Pu) and CeO2 nanoparticles, illustrating the similarities between the systems and suggested that the reduced coordination numbers observed in EXAFS fitting was a result of the core-shell nature of AnO2 nanoparticles (Romanchuk et al., 2022).
In this context, this work aims at investigating the impact of temperature, ageing time and pH on the structure and solubility of a freshly precipitated ThO2(am, hyd) solid phase. A multi-method approach including XRD, SEM, XPS, TG-DTA and EXAFS is used to thoroughly characterize the resulting solid phases, with special focus on particle size, degree of hydration and surface properties. In combination with solubility data, this information is used to derive thermodynamic properties for the solid phases investigated, which are compared with thermodynamic data selected in the NEA-TDB reference database. The results contribute to the quantitative description of radionuclide aqueous systems of potential relevance in the context of high-level waste disposal. At a more fundamental level, the work provides new insights on the structural evolution of the ThO2(s, hyd) solid phases in the transition from amorphous to crystalline, with special focus on the role of water and surface effects.
2 Experimental
2.1 Chemicals
Thorium nitrate pentahydrate (Th(NO3)4∙5H2O), sodium chloride (NaCl), nitric acid (ultrapure), HCl Titrisol© and NaOH Titrisol© were purchased from Merck. Ethanol (99.9%) was obtained from VWR Chemicals. All solutions were prepared with Milli-Q water (Milli–Q academic, Millipore, 18.2 MΩ cm). Before use, Milli-Q water was purged with Ar for >1 h to remove traces of dissolved CO2(g). All samples were prepared and stored in an Ar-glove box (<1 ppm O2), either at T = 22 or 80°C.
2.2 pH measurements
pH values were measured with combination glass electrodes (Orion ROSS), calibrated against commercial pH buffer solutions (Merck, pH 2–10). In salt solutions of ionic strength I ≥ 0.1 mol kg−1, the measured pH value (pHexp) is an operational value related to [H+] by pHm = pHexp + Am (with [H+] in molal units). Am is an empirical parameter accounting for the liquid junction potential of the electrode and the activity coefficient of H+, which is given as a function of the background electrolyte concentration. Values of Am in NaCl solutions were taken from the literature (Altmaier et al., 2003).
2.3 Solid phase preparation and solubility experiments with ThO2(s, hyd)
A232Th(IV) stock solution was prepared by slow titration of a 0.15 M Th(NO3)4 solution to pH ≈ 10–11 using 1.0 M NaOH. The resulting solid, i.e. ThO2(am, hyd), was separated from the nitrate-rich supernatant by centrifugation at 4,000 g for 10–15 min. The solid phase was dissolved in 0.1 M HCl, and the procedure was repeated until nitrate was washed out (<10 ppm, determined with colorimetric test strips Merck MQuant®). Approximately 1.2 g of ThO2(am, hyd) solid phase was obtained in a final slow titration, which was divided into nine aliquots of approximately 130 mg each. Eight of the aliquots were contacted with either 0.1 M HCl–NaCl (pHm ≈ 3) or 0.1 M NaOH (pHm = 12.8) at T = (22 ± 2) °C, and aged at T = (80 ± 2)°C for 1, 2, 4.5 and 5.5 months. The remaining aliquot was used for the study of the freshly precipitated hydrous oxide. Due to the temperature-dependence of the pKw of water, the actual pHm of the 0.1 M NaOH suspensions was significantly lower at T = (80 ± 2) °C, i.e. pHm (T = 80°C) = 11.2.
Different series of undersaturation solubility experiments at T = 22°C were prepared using the fresh and aged solid phases described above. Each independent batch sample was prepared with 1.5–3 ml of the corresponding solid suspension (fresh precipitate or solid phases aged at T = 80°C). This aliquot was centrifuged for 5 min at 4,000 g, separated from the supernatant, and washed two times with the corresponding equilibration solution. After the last washing step, the solid phase was contacted with 5–20 ml (depending upon pHm) of the equilibration solution. Seven series of solubility experiments were prepared using a freshly precipitated ThO2(am, hyd), and ThO2(s, hyd) aged for 1, 2 and 4.5 months (pHm = 3 and 12.8) at T = (80 ± 2)°C. These solid phases were equilibrated at T = 22°C in 0.1 M HCl–NaCl solutions with 2.3 < pHm < 6.3. Concentration of Th and pH were monitored at regular time intervals until equilibrium conditions were attained (defined by constant [Th] and pHm readings). For the determination of thorium concentrations, an aliquot of the supernatant of each sample was centrifuged (12,000 g) with 10 kDa filters (NanoSep Merck Millipore, pore size ∼2 nm). A given volume of the resulting filtrate was diluted with 2% ultrapure HNO3, and Th concentration was quantified by ICP-MS (Perkin Elmer ELAN 6100).
2.4 Solid phase characterization
2.4.1 XRD, TG-DTA, SEM and XPS
X-ray powder diffraction (XRD) measurements were performed with a Bruker AXS D8 Advance X-Ray powder diffractometer (Cu-Kα radiation, LynxEye XE-T detector). An aliquot with approximately 1–2 mg of each solid phase was washed 3 times with 0.5 ml of ethanol to remove the matrix solutions. After the last washing step, the solid phase was re-suspended in ethanol and deposited as suspension on a spot prepared with vaseline on a XRD sample plate. The measurement angle was 2° < 2θ < 70° with incremental steps of 0.015° and a measurement time of 0.4 s for each step. Diffractograms collected were compared with reference data reported in the Joint Committee on Powder Diffraction Standard (JCPDS, 2001). Based on the full width at half maximum (FWHM) of the diffraction peaks, the crystallite size for a given solid was calculated using the Scherrer equation (Scherrer, 1918; Holzwarth and Gibson, 2011).
An aliquot of the washed solid was prepared on an indium foil, dried under Ar atmosphere, and subsequently analyzed by X-ray photoelectron spectroscopy (XPS) and scanning electron microscopy (SEM). XPS measurements were performed with a XPS system PHI 5000 VersaProbe II (ULVAC-PHI Inc.) equipped with a scanning microprobe X-ray source (monochromatic Al Kα, hν = 1,486.7 eV). Binding energies of elemental lines are charge referenced to the oxidic portion of the O 1s spectrum at 530.0 eV. A FEI Quanta 650 FEG environmental scanning electron microscope (now Thermo Fisher Scientific Inc.) was used to image the carbon coated sample surfaces. The primary electron beam energy was 30 keV. Relative atomic concentrations (H not detected) were calculated by areas of elemental lines of survey spectra, recorded at 187.85 eV pass energy of the analyzer, after subtraction of a local Shirley background and taking into account sensitivity factors and asymmetry parameters of elemental lines, and the transmission function of the analyzer (Moulder et al., 1995; Briggs and Grant, 2003). Relative error of semiquantitative atomic concentrations is typically within ± (10–20)%. Curve fits to narrow scans of elemental lines recorded at 23.5 eV pass energy were performed by Gaussian functions after Shirley background subtraction. Molar concentrations of elemental species are calculated by atomic concentrations and curve fit results. Data analysis was performed using ULVAC-PHI MultiPak program, version 9.9.
Thermogravimetric analysis (TG) with differential thermal analysis (DTA) were performed under Ar atmosphere using a Netzsch STA 449C equipment. Measurements were performed with 10–20 mg of dry solid material. Samples were heated up to 1,200 °C with a heating rate of 10 K min−1.
2.4.2 EXAFS measurements
Th L3 edge XAFS spectra were recorded at the INE beamline of the KIT Light Source (KARA storage ring), Karlsruhe, Germany (Rothe et al., 2019). Ge (4 2 2) crystals were used in the Lemonnier-type double crystal monochromator. The monochromated radiation was focused by a Rh-coated toroidal mirror resulting in a spot size of <1 mm at the sample position. EXAFS measurements were performed with selected solid samples, i.e. ThO2(am, hyd, fresh) and three aged solid phases ThO2(s, hyd, aged): t = 1 m at pHm = 3, t = 1 m at pHm = 12.8 and t = 5.5 m at pHm = 12.8. Approximately 10–15 mg of each solid phase was washed once with a weakly alkaline solution (pHm ≈ 9.2) and re-suspended in a small volume of the same solution. The resulting suspensions were placed into a sealed, liquid nitrogen stable sample holder. The plastic cells were contained under Ar atmosphere in a gas-tight cell and transported to the synchrotron source, where they were stored under Ar atmosphere until the EXAFS measurements. Samples were measured at 80 K in a liquid nitrogen cooled cryostat in fluorescence acquisition mode simultaneously using a 1-pixel and a 4-pixel silicon drift (Vortex) detector. The excitation energy scale was calibrated using a ThO2 reference standard (first inflection point calibrated to 16,300 eV). The resulting XAFS spectra were processed and analysed using Athena and Artemis software from the Demeter software package (FEFF 6) (Ravel and Newville, 2005). The spectra were fit using a ‘shell-by-shell’ approach using fixed coordination numbers to limit number of fit parameters, and the statistical validity of each shell was verified by way of an F-test (>95% validity) (Downward et al., 2007).
3 Results and discussion
3.1 Solid phase characterization
3.1.1 XRD
Figure 1 shows the powder diffractograms collected for the fresh and aged Th(IV) solid phases investigated within this study. The figure includes also the main diffraction lines reported for the ThO2(cr) reference (PDF 75-0052).
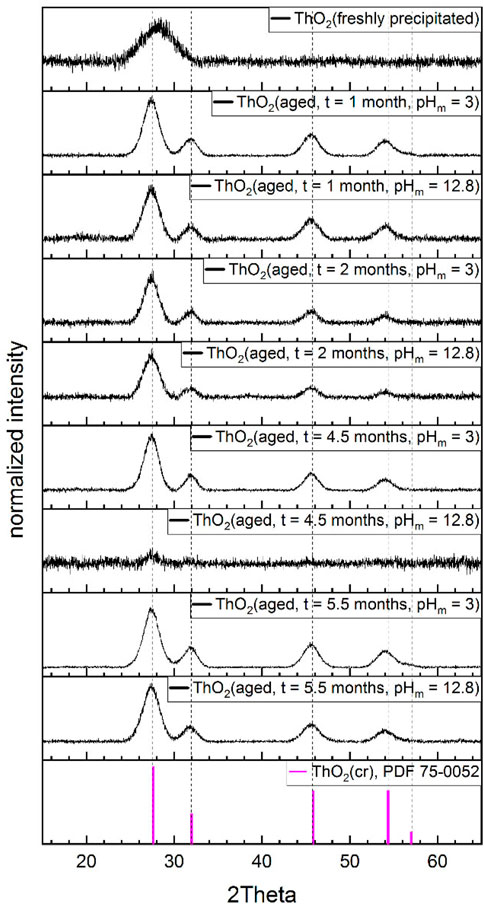
FIGURE 1. Diffractograms of the Th(IV) solid phases synthesized in this work and equilibrated at T = 80°C and pHm(25°C) = 3 and 12.8, except for the sample “ThO2(freshly precipitated)”, which was measured 2 days after precipitation. Vertical dashed lines refer to the ThO2(cr) reference (PDF 75-0052). The low quality of the diffractogramm obtained for the sample aged for 4.5 months at pHm = 12.8 was caused by the limited amount of solid phase available.
The diffractogram obtained for the freshly precipitated material shows a main broad feature at 2Θ ≈ 28.5°, which reflects the amorphous character of the solid phase. This is in line with previous observations reported by Kobayashi and co-workers for freshly precipitated Th(IV) hydrous oxide (Kobayashi et al., 2016). In all other cases, XRD patterns show narrower peaks with well-defined 2Θ positions, reflecting a higher degree of crystallinity for the Th(IV) aged samples. No significant differences are visually observed in the diffractograms of Th(IV) solid phases aged for different times or at different pHm values. The peak positions in the XRD of the aged samples are in excellent agreement with reference data reported for ThO2(cr) in the JCPDS database, with main reflections at 2Θ = 27.6, 32.0, 45.9, 54.4 and 57.0°. A good agreement is also obtained with the peak positions in the XRD reported by Kobayashi et al. for Th(IV) solid phases aged at T = 90°C for 3–6 weeks in 0.1–2.0 M NaClO4 and 0.1–3.0 M NaCl (Kobayashi et al., 2016). Plakhova and co-workers reported well-resolved XRD patterns for freshly precipitated ThO2(s, hyd) (Plakhova et al., 2019). However, we note that the drying process at T = 40 and 150°C followed by the authors may have increased the degree of crystallinity of the originally precipitated Th(IV) solid phase.
Table 1 shows the results of the Scherrer analysis based on the evaluation of the full width at half maximum (FWHM) intensity of the XRD peaks in Figure 1. Large differences are observed between the crystallite size of freshly precipitated and aged ThO2(s, hyd) solid phases, whereas very similar crystallite sizes are quantified for solid phases aged at T = 80°C for samples aged for different lengths of time. For the same ageing time, the Scherrer analysis hints towards the formation of slightly larger crystallites in the solid phases aged at pHm = 3 than for those aged at pHm = 12.8, although the values of the crystallite size overlap within their uncertainties. This observation could be rationalized by the higher solubility of Th(IV) at pHm = 3 (≈10−2 M, calculated at T = 25°C for ThO2(am, hyd, aged)) than at pHm = 12.8 (≈10−8 M), which may result in a faster particle growth through enhanced dissolution and precipitation reactions.
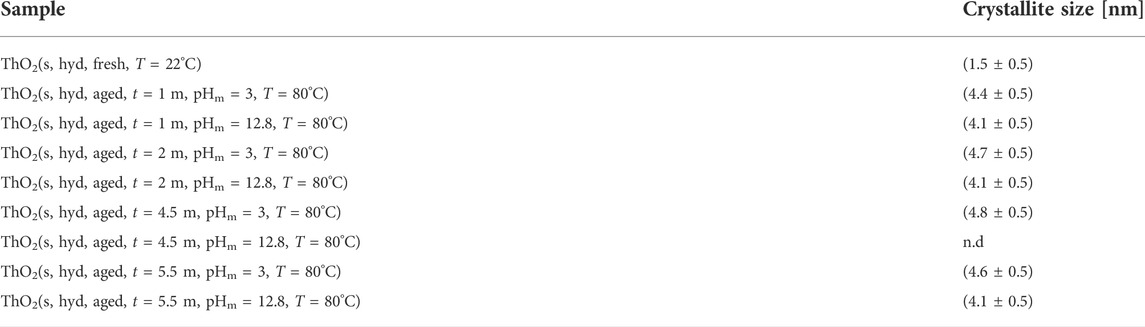
TABLE 1. Scherrer analysis of the ThO2(s, hyd, fresh) and ThO2(s, hyd, aged) solid phases investigated in this work. n.d. stands for not determined.
Kobayashi et al. reported crystallite sizes between 3.1 and 4 nm for selected Th(IV) solid phases aged at T = 90°C (Kobayashi et al., 2016), in moderate agreement with crystallite sizes determined in this work. Plakhova and co-workers reported crystallite sizes of ≈2 and ≈3.6 nm for ThO2(s, hyd) solid phases precipitated at room temperature (but dried at T = 40 and 150°C) in 3.0 M NH3⋅H2O and 3.0 M NaOH, respectively (Plakhova et al., 2019). The hydrothermal treatment of Th(IV) solid phases in H2O (T = 210°C, pH not specified) and 3.0 M NaOH (T = 180°C) resulted in particle sizes of ≈4.3 and ≈4.7 nm, respectively.
3.1.2 TG-DTA, SEM and XPS
In the process of particle growth through ageing (either at room or elevated temperatures), a decrease of the number of hydration waters in the solid ThO2(am, hyd)1 is expected. The number of hydration waters in An (IV) hydrous oxides has been often assumed as 2 (i.e. AnO2⋅2H2O(s) ≅ An(OH)4(s)), although a few previous studies report clearly lower values of hydration waters (0.6–1) for M(IV) solid phases aged for 1–3 years at room temperature (Yalcintas et al., 2016; Cevirim-Papaioannou, 2018). TG-DTA measurements were performed to determine the number of hydration waters of freshly precipitated ThO2(am, hyd) as well as of solid phases aged at T = 80°C for t = 1, 2, 4.5 and 5.5 months. The main quantitative outcome evaluated from the TG-DTA data is the total weight loss measured up to 1,200°C, which is assigned to the number of hydration waters in the investigated hydrous oxides (see Table 2). This evaluation approach is a simplification of the actual situation, where loosely bound water, sticking moisture, hydroxide groups and (less likely) crystal waters might be present. The results in the table clearly show a significantly larger amount of hydration waters in the freshly precipitated solid (n = 2.9 ± 0.1) compared to the aged phases (n = 1.4 ± 0.2). No clear trend is observed for the latter as a function of time or pH. The values of hydration water determined for the aged solid phases are in line with data reported by Cevirim-Papaioannou for a U(IV) hydrous oxide phase aged at T = 22°C for up to 798 days (n = 1.0 ± 0.5) (Cevirim-Papaioannou, 2018). Figure 2 shows the evolution of the number of hydration waters in M(IV)O2 (s, hyd) as a function of time, with M = Th (this work), U (Cevirim-Papaioannou, 2018) and Tc (Yalcintas et al., 2016; Grenthe et al., 2020). The figure shows a clear qualitative trend to decrease the number of hydration waters with ageing time, thus supporting the transformation of hydroxide/hydrated phases into the corresponding, thermodynamically stable, oxides.
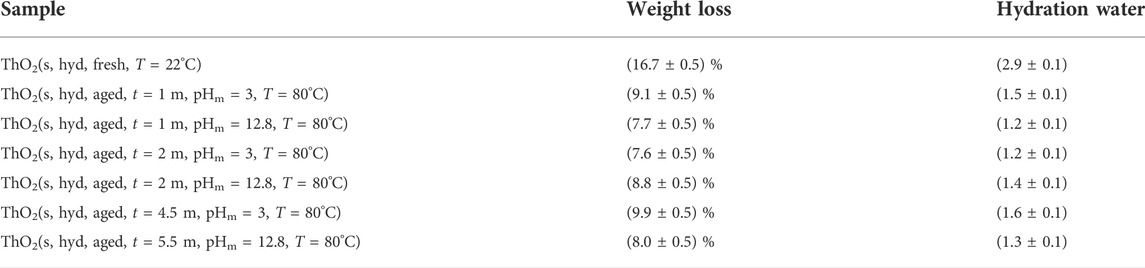
TABLE 2. Weight loss and calculated number of hydration waters as quantified by TG-DTA for the Th(IV) hydrous phases investigated in this work.
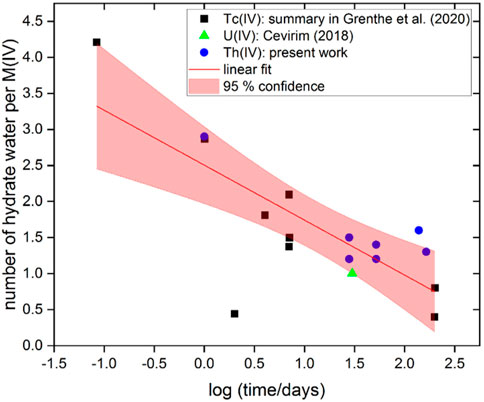
FIGURE 2. Evolution of the number of hydration waters in M(IV)O2 (s, hyd) with time as reported in this work for Th or reported in the literature for U (Cevirim-Papaioannou, 2018) and Tc (Yalcintas et al., 2016; Grenthe et al., 2020). Figure modified after (Grenthe et al., 2020) (Tc chapter, prepared by B. Grambow).
All SEM pictures show irregular aggregates of ≈20 to ≈100 nm (see Figure SI-1 in Supporting Information). No clear trend can be observed as function of the ageing time or ageing pH, which is likely related to the very small and similar particle size observed for all aged samples. Similar aggregates were previously reported for other An(IV)O2(am, hyd) systems (with M = Tc, U, Np, Pu) precipitated at room temperature (Fellhauer, 2013; Yalcintas, 2015; Cevirim-Papaioannou, 2018). Note that much more regular aggregates were obtained in recent studies investigating the size and local environment of Th(IV) nanoparticles exposed to much higher temperatures (400–1,000°C) (Amidani et al., 2019; Bonato et al., 2020).
Supplementary Figures SI-2A–C in the Supporting Information exemplarily shows the complete survey XP spectrum of the Th(IV) sample equilibrated at T = 80°C for 2 months at pHm = 3, as well as the narrow scans of the Th 4f and the O 1s lines. The narrow scan of the O 1s line includes also the fit with the hydrate, hydroxide and oxide contributions. The XP spectra of all investigated solids are shown together in Figures 3A,B. Table 3 summarizes the atom % of Th and O as well as the ratio [O]/[Th] quantified considering the intensities of the Th 4f and the O 1s lines. In all cases, the ratio O: Th is well above 2 (2.3–2.8), thus underpinning the significant presence of hydroxide and hydrate groups that enhance the ratio [O]/[Th] beyond the value of two present in the crystalline ThO2(cr). Table 3 summarizes also the speciation of oxygen (as hydrate, hydroxide or oxide) resulting from the fit of the O 1s line.
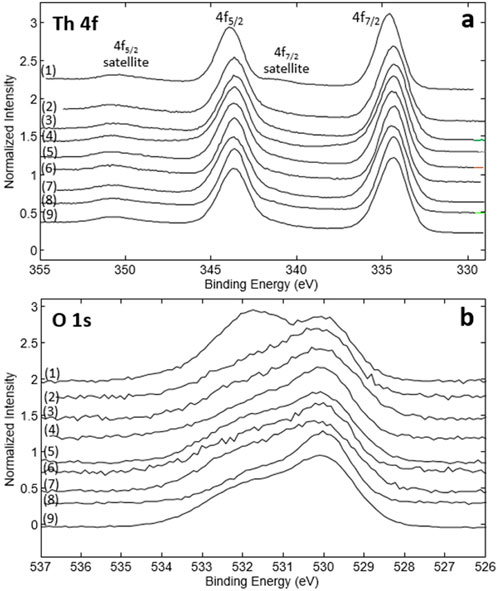
FIGURE 3. XPS narrow scans of Th(IV) hydrous oxides: (A) Th 4f lines; (B) O 1s lines. (1) freshly precipitated solid phase. Solids aged at T = 80°C: (2) 1 month at pHm = 3; (3) 1 month at pHm = 12.8; (4) 2 months at pHm = 3; (5) 2 months at pHm = 12.8; (6) 4.5 months at pHm = 3; (7) 4.5 months at pHm = 12.8; (8) 5.5 months at pHm = 3; (9) 5.5 months at pHm = 12.8.
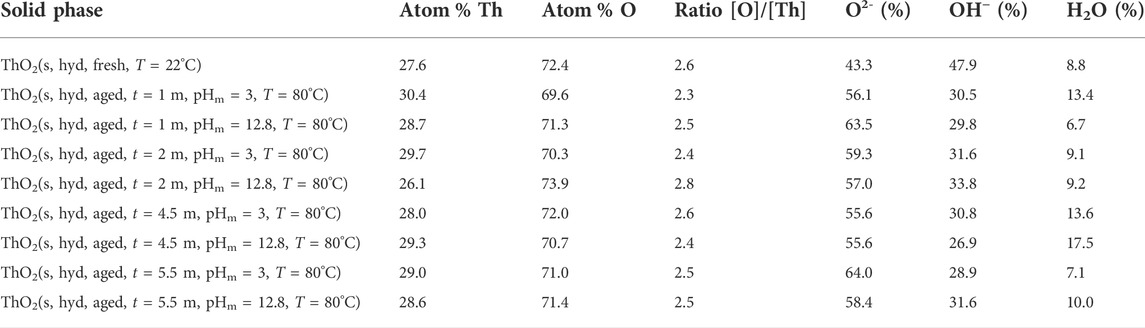
TABLE 3. Atomic % of Th and O, ratio [O]/[Th] and speciation of oxygen (as atomic percent of oxide, hydroxide and water) as calculated from the Th 4f and O 1s lines in the XPS measurements. Relative error of atomic concentrations is within ± (10–20) %.
Consistently with other solid phase characterization techniques considered in this work (XRD, TG-DTA), XPS provides clear evidence of the differences between the freshly precipitated Th(IV) hydrous oxide and the corresponding solids aged at T = 80°C. However, no clear trends are observed in the XPS analysis of solid phases aged for different times or at different pH values. The fraction of oxide calculated as average of all solid phases equilibrated at pHm = 3 (58.8 ± 5.8%) is virtually the same as the average obtained for solid phases equilibrated at pHm = 12.8 (58.6 ± 4.9%). However, the oxide content in all aged samples is clearly above the fraction of oxide quantified for the freshly precipitated Th(IV) hydrous oxide (43.3%).
Both bulk (XRD, TG-DTA) and surface-sensitive techniques (XPS) considered for the characterization of the solid phases show no clear effect of ageing time or pH on the properties of the investigated Th(IV) hydrous oxides. However, it is important to bear in mind that XPS provides an information depth of about 3 nm at the experimental conditions. Alterations affecting a monolayer at the Th(IV) oxide surface will most likely not be clearly identified by this technique.
3.1.3 EXAFS
Th L3 edge EXAFS data and fits are shown in Figure 4, and details of best fits are presented in Table 4. Freshly precipitated as well as solid phases aged at T = 80 °C for 1 month (pHm = 3 and 12.8) and 5.5 months (pH = 12.8) were analysed. All samples were fit with one or two O shells at 2.37–2.54 Å and a Th backscatterer at (3.96 ± 0.04) Å, with all three aged samples also including a distant O shell at (4.58 ± 0.04) Å. These distances are in general agreement with the structure of crystalline ThO2 (Wyckoff, 1963), however there are some deviations from the crystalline structure which are more prominent in the less aged samples. For the fresh and 1 month aged samples, at both pHm 3 and 12.8, best fits contain a split first shell coordination. Here, two O shells at (2.37 ± 0.03) and (2.53 ± 0.04) Å were fit, compared to one O shell at 2.43 Å that would be expected for crystalline ThO2. The two shells have coordination numbers between 4.8 and three each, with total coordination ranging from 7.8 to 8.7 for each fit, which is in close agreement with the coordination number of eight for the single O shell in crystalline ThO2. These findings are consistent with previous EXAFS fitting of ThO2(am,hyd) nanoparticles (Neck et al., 2002), and this is representative of a distortion of the crystal structure in the fresh and 1 month aged precipitates. However, this distortion does not appear to affect the longer distance backscatterers for all fits, with Th and O backscatterers fit at distances anticipated for a ThO2-like, fluorite-type structure. The main difference between the fits is in the fresh sample, where the best fit includes only 5.5 Th backscatterers, as opposed to 12 Th backscatterers anticipated for crystalline ThO2. This value is much closer for the sample aged 1 month at pH = 3 (11.6), 1 month at pH = 12.8 (12) and 5.5 months at pH = 12.8 (11.4) fits. These results are consistent with the fresh sample having significantly different structure to the other three samples, likely due to a much smaller crystallite size and/or much higher structural disorder resulting in reduced long-range coordination, seen as a reduced Th backscatterer coordination number.
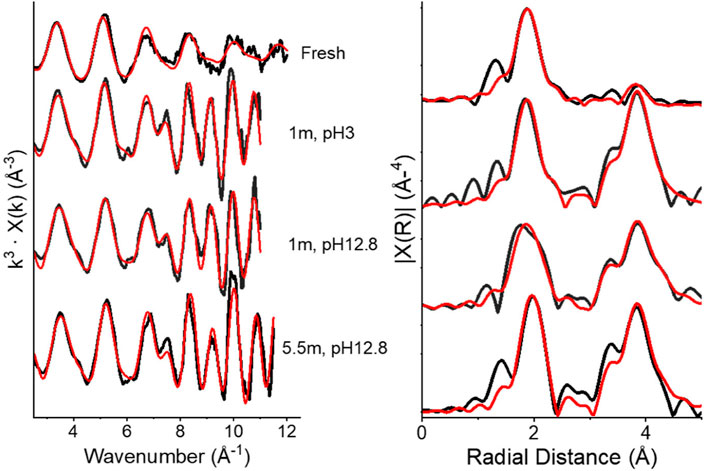
FIGURE 4. EXAFS (left) and Fourier-Transformed EXAFS (right) for (from top to bottom) ThO2(s, hyd) freshly precipitated, ThO2(s, hyd) aged, t = 1 m, pHm = 3, ThO2(s, hyd) aged, t = 1 m, pHm = 12.8 and ThO2(s, hyd) aged, t = 5.5 m, pHm = 12.8. Black lines are experimental data and red lines are fits.
Overall, EXAFS fitting suggests that the structure of these nanoparticles becomes more crystalline over time. The fresh sample has the lowest Th backscatterer coordination number and a split first O shell, the two 1 month aged samples retain this split O shell, but have Th backscatterer coordination numbers more closely matching ThO2 and also a distant O shell at (4.58 ± 0.04) Å. Finally, the 5.5 months aged sample has a single O shell with a Th-O distance identical to that of crystalline ThO2, as well as Th and distant O backscatterers present with coordination numbers similar to those in the crystalline structure (Table 1, see data reported by Rothe et al., 2002).
3.2 Solubility experiments
Figures 5A–D shows solubility of Th(IV) determined in this work for freshly precipitated ThO2(am, hyd, fresh) and ThO2(s, hyd, aged) aged at T = 80 °C for t = 1, 2, 4.5 m and pHm = 3, 12.8. The figure also shows the solubility curves corresponding to freshly precipitated, aged and crystalline ThO2 solid phases calculated using the solubility and hydrolysis constants selected in the NEA-TDB (Rand et al., 2009), as well as solubility curves calculated with the solubility constants derived in this work (see Section 3.3).
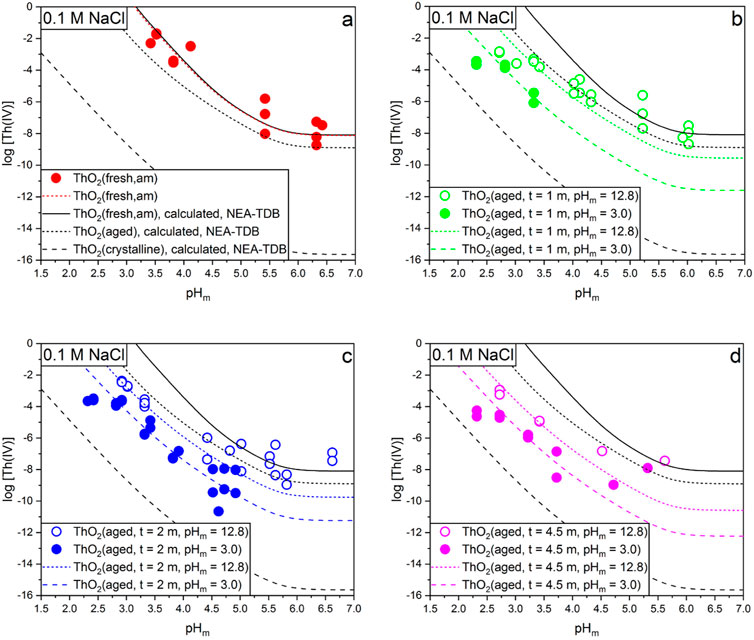
FIGURE 5. Experimental solubility data obtained in this work and model calculations using thermodynamic data derived in this work or reported in the NEA-TDB for Th(IV) hydrous oxide: (A) freshly precipitated; (B) aged for 1m at T = 80°C and pHm = 3 and 12.8; (C) aged for 2m at T = 80°C and pHm = 3 and 12.8; (D) aged for 4.5m at T = 80°C and pHm = 3 and 12.8.
The experimental data on the freshly precipitated Th(IV) hydrous oxide agrees well with the solubility calculated for ThO2(am, hyd, fresh) using the NEA-TDB thermodynamic selection (Figure 5A). Experimental data are also in moderate agreement with previous studies reporting the solubility of ThO2(am, hyd) at T = 25°C in 0.1 M NaCl or NaClO4 (Ryan and Rai, 1987; Rai et al., 2000). All aged Th(IV) solid phases show lower solubilities than ThO2(am, hyd, fresh), consistent with the increase in particle size/crystallinity observed by XRD. This observation supports also that aged solids are not a mixture of (nano-)crystalline and amorphous phases, as in this case the solubility should be defined by the most soluble solid, i.e. the amorphous phase. Solubility data obtained for the Th(IV) solid phase aged for 2 months at pHm = 3 is also in good agreement with experimental data reported by Kobayashi and co-workers for a solid phase aged at T = 90°C during 6–8 weeks. Note however that Kobayashi et al. followed a different ageing approach as used in this study–each independent solubility sample was aged at T = 90°C at the target pH (ranging between ≈1.5 and ≈9), whereas in the present work the solid phase used in each solubility series was aged at a single pH. Because of the impact of pH in the ageing process (see next paragraph and Figure 5), the different ageing approach followed in Kobayashi et al. and in this work may lead to differences in the solubility data, especially in the less acidic samples. Recently, Nisbet and co-workers investigated the solubility of ThO2(cr) in the temperature range 150–250°C (Nisbet et al., 2018). The authors did not report solubility constants for the investigated systems, but the measured Th concentrations are clearly lower as those observed in the present study, in line with the crystalline character of their solid phase.
Experimental data in Figure 5 show that the solubility of the Th(IV) hydrous oxide slightly decreases with the ageing time at T = 80°C. Unexpectedly, the pH in which the Th(IV) solid phase was aged has a very significant effect on the solubility measured at T = 22°C. Hence, the solid phases aged at pHm = 3 show up to two orders of magnitude lower solubility than the solid phases aged at pHm = 12.8. This effect is reproduced for the solid phases aged during 1, 2 and 4.5 months. These observations are apparently in contradiction with the minor differences observed by XRD, TG-DTA and XPS for Th(IV) solid phases aged for different contact times and at different pH values. However, these results can be rationalized by a solubility control established by a few monolayers of the ThO2(s, hyd) surface. Such few monolayers have a minor weight in bulk characterization methods (XRD, TG-DTA) but also in “surface-sensitive” methods like XPS, which provides average values of a ≈3 nm layer. This hypothesis is also in line with previous studies by Grambow, Vandenborre and co-workers (Vandenborre et al., 2010; Grambow et al., 2017), who claimed that solubility measurements of ZrO2(s), ThO2(s) and UO2(s) are not representative of the bulk phase, but are rather controlled by surface processes of a few monolayers of the corresponding oxide. Although the starting materials used in these studies were crystalline oxides sintered at very high temperatures (400–1,000°C), the authors claimed that “solubility” of Th(IV) system was controlled by “ThOx (OH)y (H2O)z” present in the grain boundaries.
As already discussed above, the in general higher solubility of Th(IV) at lower pH induces faster recrystallization rates, concomitant with somewhat larger diameters of crystalline units. Even though the characterization methods applied in the present work are not able to resolve variations in the first surface monolayers, we assume that the higher recrystallization rate at low pH may also have an impact on the structure of surfacial ThOx (OH)y (H2O)z species finally leading to a reduced solubility. Moreover, differences in the surface charge of the ThO2(s, hyd) (positive at pHm = 3, negative at pHm = 12.8) can possibly affect to the ageing process, and consequently influence solubility.
3.3 Thermodynamic evaluation of solubility phenomena
Experimental solubility data were modelled with the aim of determining the solubility constants (log *K°s,0) of the investigated Th(IV) hydrous oxides. The model of the system controlling the solubility of Th(IV) includes both monomeric (Th4+, ThOH3+, Th(OH)22+, Th(OH)4 (aq)) and polyatomic (Th2(OH)26+, Th2(OH)35+, Th4(OH)88+, Th4(OH)124+, Th6(OH)159+, Th6(OH)1410+) aqueous species as selected in the NEA-TDB (Rand et al., 2009), as well as the solid phases ThO2(am, hyd, fresh) and ThO2(ncr, hyd, t, pHm), with t = 1, 2, 4.5months and pHm = 3, 12.8. The term “ncr” indicates the nanocrystalline character of the Th(IV) hydrous oxides solid phases obtained after the hydrothermal ageing at T = 80°C. The modelling approach is based on the fit of log *Ks,0°, whereas the values of log *β(n,m)° are kept constant as selected by NEA-TDB. The datasets collected for each solid phase are fitted individually by minimizing the function ∑((log[Th]exp–log[Th]calc)2)1/2. The value [Th]calc is the sum of the species [Th4+] [ThOH3+] [Th(OH)22+] [Th(OH)4 (aq)] [Th2(OH)26+] [Th2(OH)35+] [Th4(OH)88+] [Th4(OH)124+] [Th6(OH)159+] and [Th6(OH)1410+], and can be calculated as:
where the values of *β°(n,m) are know from the NEA-TDB and γH+ is calculated using the SIT formalism (Ciavatta, 1980; Grenthe et al., 2020). The values of hydration water x = 2.9 and x = 1.4 have been considered for the modelling of the freshly precipitated and aged solid phases, respectively, where x = (1.4 ± 0.3) is the average value of the number of hydration waters quantified for all aged solid phases. The impact of x in the current modelling calculations is however very minor in the conditions of this study, because the water activity in 0.1 M NaCl is close to unity, i.e. aw = 0.9966. The outcome of this modelling exercise is shown in Figure 5, whereas Table 5 summarizes the log *K°s,0 values determined for all investigated Th(IV) hydrous oxides as compared to the values selected by NEA-TDB.
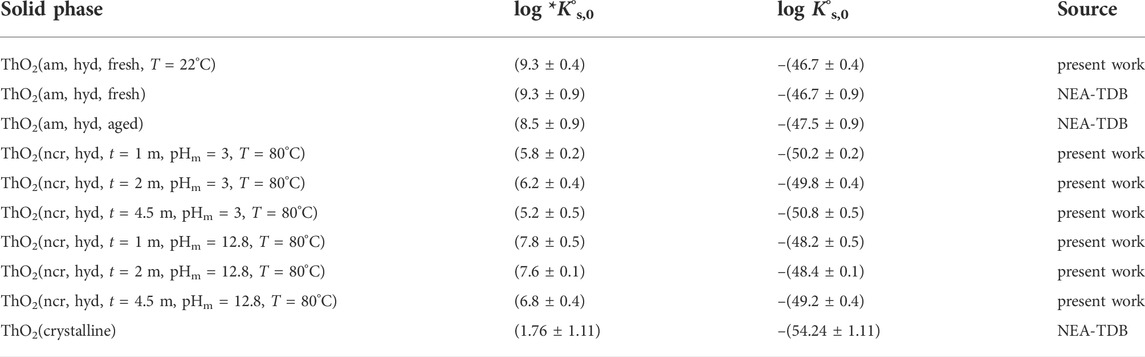
TABLE 5. Solubility constants for ThO2(s, hyd) and ThO2(cr) as determined in this work or selected in the NEA-TDB (Rand et al., 2009). The values of log *K°s,0 and log K°s,0 correspond to the equilibrium reactions ThO2⋅nH2O(s) + 4 H+ ⇔ Th4+ + (2 + n) H2O(l) and ThO2⋅nH2O(s) + (2–n) H2O(l) ⇔ Th4+ + 4 OH−, respectively.
The solubility constant determined in this work for ThO2(am, hyd, fresh) (log K°s,0 = 46.7 ± 0.4) is in excellent agreement with the value selected in the NEA-TDB, log K°s,0 = (46.7 ± 0.9). This supports the validity of the solubility experiments and modelling approach, and represents a sound anchoring point for the quantification of the solubility constants of the aged phases investigated in this work. A very significant decrease in the solubility constant (≥1.5 log10-units) with respect to the freshly precipitated solid phase is observed for the aged solid phases already after 1 month of ageing time. A further decrease in the values of log Ks,0° is observed with the increase of aging period. However, the most significant effect on the values of log K°s,0 is observed as a function of the ageing pH. Hence, in average, the samples aged at pHm = 3 have a solubility constant of ca. 2 log10-units lower than the solid phases aged at pHm = 12.8. This behavior is a strong evidence of the effect of pH on the recrystallization rate, which is higher at pHm = 3 due to the higher solubility of Th(IV) under acidic conditions.
The strong impact of the ageing time and pH on the value of log K°s,0 is however not reflected in differences observed by the different solid phase characterization techniques considered in this work, i.e. XRD, TG-DTA, SEM, XPS and XAFS. As discussed in Section 3.2, this likely reflects that solubility phenomena in the investigated system is mostly controlled by surface processes occurring in the first monolayer of the Th(IV) oxide (0.2–0.4 nm). Indirect evidences pointing to the same hypothesis were reported by Vandenborre and co-workers on the basis of isotopic exchange experiments with 229Th (Vandenborre et al., 2010; Grambow et al., 2017).
Differences in the solubility of ThO2(am, hyd) solid phases have been often attributed to the effect of particle size (and by the extension of the surface area) in the Gibbs energy of formation (ΔfG°m) of the solid (Neck et al., 2007; Kobayashi et al., 2016). The Schindler equation correlates the ΔfG°m of an amorphous/colloidal solid phase with the ΔfG°m of the corresponding crystalline phase considering a term with the surface contribution (Schindler, 1967; Neck et al., 2007):
where ΔfG°m is directly related to the solubility constant through the Gibbs energy of reaction (ΔrG°m), S is the molar surface that depends on the particle size, and γ is the mean free surface energy per unit surface area of solid-liquid interface. The molar surface is defined as
With the known data for ThO2(cr) (ΔfG°m = –1,169.2 kJ mol−1, M = 264 g mol−1, ρ = 10.0 g cm−3,
The Schindler equation acknowledges the key role of the surface on the overall stability of a solid phase, and thus on its solubility constant. Although the assumption of homogeneous spherical particles is not confirmed in the present work, experimental solubility constants determined in this study in combination with particle size determined by the Scherrer equation have been compared with model predictions using the Schindler equation. The molar standard Gibbs energy values for ThO2 solid phases of the present work are calculated according to the following equations:
In order to avoid the bias introduced by the contribution of ΔfG°m (H2O, l) in Th(IV) oxide phases with different number of hydration waters, the following reaction is considered for the calculation of the Gibbs energy of formation of the solid:
which leads to:
where
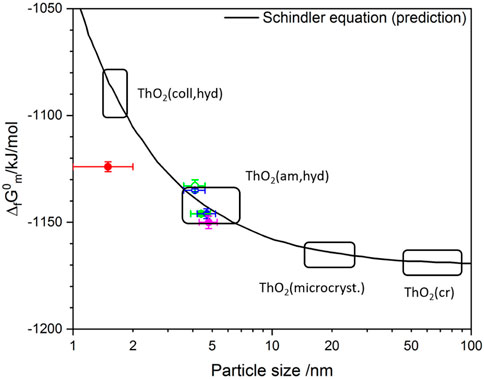
FIGURE 6. Application of Schindler equation to the ThO2(s) system and comparison with experimental data determined in the present work. Figure modified from (Neck et al., 2007).
Figure 6 shows qualitatively a good agreement between ΔfG°m calculated from experimental solubility data and model predictions using the Schindler equation. A somehow large deviation is observed for the freshly precipitated Th(IV) hydrous oxide, whereas ΔfG°m determined for the aged phases agree well with model calculations. Experimental evidences obtained in this work provide an indirect link between solubility phenomena in the ThO2(s) system with surface properties of a (few) monolayer(s) of the solid. Although surface is unequivocally playing a key role in the solubility equilibrium, it is less evident that particle size is the driving force controlling solubility in the ThO2(s) as assumed in the Schindler equation.
4 Summary and conclusion
The influence of temperature on Th(IV) solid phases and solubility was systematically investigated under Ar atmosphere using a freshly precipitated ThO2(am, hyd) solid phase as starting material. The fresh precipitate was aged at T = 80°C for different time periods (t = 1, 2, 4.5, 5.5 m) and at two different pHm values (pHm = 3.0, 12.8). The resulting solid phases were comprehensively characterized using a multimethod approach including XRD, TG-DTA, SEM, XPS and XAFS techniques. Selected solid phases were used for the preparation of solubility experiments at T = 22°C in 0.1 M NaCl solutions with 2.3 ≤ pHm ≤ 7.0. These data, in combination with the hydrolysis constants and SIT coefficients selected in the NEA-TDB, were used to derive the corresponding solubility constants (log K°s,0) and assess the systematic variations caused by the ageing at elevated temperatures.
The ageing of a freshly precipitated ThO2(am, hyd) solid phase at T = 80°C induces a significant increase of the crystallinity and particle size, as confirmed by XRD measurements. TG-DTA and XPS support that this process is accompanied by an important decrease in the number of hydration waters/hydroxide groups in the original amorphous Th(IV) hydrous oxide, ThOx (OH)y⋅zH2O(s). EXAFS data show a decreased number of Th-Th backscatterers at ≈ 3.96 Å in the freshly precipitated solid phase, however in the solid phases aged at T = 80°C the number of Th-Th backscatterers approaches the ThO2(cr) bulk value. Nevertheless, all solid characterization methods used in this work are unable to resolve clear differences between solid phases aged for different time periods or at different pHm values.
Solubility experiments with fresh and aged Th(IV) solid phases conducted at T = 22°C show a clear decrease in the solubility of the solid phases aged at T = 80°C. In contrast to the observations gained by solid phase characterization, the ageing time and (specially) ageing pHm are shown to have a very important impact on the solubility measured at T = 22°C. These apparently discordant observations can be explained in a consistent manner by claiming a solubility control by a few monolayers in the surface of the ThO2(s, hyd) solid (0.2–0.4 nm), which cannot be properly probed by any of the bulk (XRD, TG-DTA, SEM, XAFS) or surface-sensitive (XPS, with a penetration depth of ca. 4 nm) techniques considered in this work.
Data availability statement
The raw data supporting the conclusion of this article will be made available by the authors, without undue reservation.
Author contributions
XG, MA, and HG defined the initial concept of the study. XG, NC, and CK contributed to conception and design of the experimental work. CK and TN performed the experiments and conducted data evaluation. DS conducted the SEM and XPS measurements, and corresponding data evaluation. TV, KD, and JR revised the EXAFS data evaluation. CK, XG, and TN wrote the first draft of the manuscript. All authors contributed to manuscript revision, read, and approved the submitted version.
Acknowledgments
Frank Geyer, Annika Kaufmann, Stephanie Kraft and Melanie Böttle (all KIT–INE) are gratefully acknowledged for the ICP–MS/OES, TG-DTA measurements and technical support. This work was partially funded by the German Federal Ministry for Education and Research under contract 02NUK039A, the ThermAc project. The Institute for Beam Physics and Technology (KIT-IBPT) is acknowledged for the operation of the Karlsruhe Research Accelerator (KARA), and provision of beamtime at the INE-Beamline operated by the Institute for Nuclear Waste Disposal at the KIT Light Source. TN and TV acknowledge funding from the European Research Council (ERC) Consolidator Grant 2020 under the European Union’s Horizon 2020 research and innovation programme (grant agreement no. 101003292). We acknowledge support by the KIT-Publication Fund of the Karlsruhe Institute of Technology.
Conflict of interest
The authors declare that the research was conducted in the absence of any commercial or financial relationships that could be construed as a potential conflict of interest.
Publisher’s note
All claims expressed in this article are solely those of the authors and do not necessarily represent those of their affiliated organizations, or those of the publisher, the editors and the reviewers. Any product that may be evaluated in this article, or claim that may be made by its manufacturer, is not guaranteed or endorsed by the publisher.
Supplementary material
The Supplementary Material for this article can be found online at: https://www.frontiersin.org/articles/10.3389/fchem.2022.1042709/full#supplementary-material
Footnotes
1The most correct definition of the solid refers to ThOx(OH)y⋅zH2O(s), with 2x + y = 4.
References
Altmaier, M., Metz, V., Neck, V., Müller, R., and Fanghänel, T. (2003). Solid-liquid equilibria of Mg(OH)2(cr) and Mg2(OH)3Cl 4H2O(cr) in the system Mg-Na-H-OH-O-Cl-H2O at 25°C. Geochim. Cosmochim. Acta 67, 3595–3601. doi:10.1016/s0016-7037(03)00165-0
Altmaier, M., Neck, V., and Fanghänel, T. (2004). Solubility and colloid formation of Th(IV) in concentrated NaCl and MgCl2 solution. Radiochim. Acta 92, 537–543. doi:10.1524/ract.92.9.537.54983
Amidani, L., Plakhova, T. V., Romanchuk, A. Y., Gerber, E., Weiss, S., Efimenko, A., et al. (2019). Understanding the size effects on the electronic structure of ThO2 nanoparticles. Phys. Chem. Chem. Phys. 21, 10635–10643. doi:10.1039/c9cp01283d
Amidani, L., Vaughan, G. B. M., Plakhova, T. V., Romanchuk, A. Y., Gerber, E., Svetogorov, R., et al. (2021). The application of HEXS and HERFD XANES for accurate structural characterisation of actinide nanomaterials: The case of ThO2. Chem. Eur. J. 27, 252–263. doi:10.1002/chem.202003360
Baes, C. F., Meyer, N. J., and Roberts, C. E. (1965). The hydrolysis of thorium(IV) at 0 and 95°. Inorg. Chem. 4, 518–527. doi:10.1021/ic50026a017
Bonato, L., Virot, M., Dumas, T., Mesbah, A., Dalodiere, E., Dieste Blanco, O., et al. (2020). Probing the local structure of nanoscale actinide oxides: A comparison between PuO2 and ThO2 nanoparticles rules out PuO2+x hypothesis. Nanoscale Adv. 2, 214–224. doi:10.1039/c9na00662a
Briggs, D., and Grant, J. T. (2003). Surface analysis by Auger and X-ray photoelectron spectroscopy. IM Publications and Surface Spectra Ltd.
Cevirim-Papaioannou, N. (2018). Redox chemistry, solubility and hydrolysis of uranium in dilute to concentrated salt systems. PhD thesis. Karlsruhe, Germany: Karlsruhe Institute of Technology.
Ciavatta, L. (1980). The specific interaction theory in evaluating ionic equilibria. Ann. Chim-Rome 70, 551–567.
Downward, L., Booth, C. H., Lukens, W., and Bridges, F. (2007). A variation of the F-test for determining statistical relevance of particular parameters in EXAFS fits. Aip Conf. Proc. 882, 129–131. doi:10.1063/1.2644450
Dzimitrowicz, D. J., Wiseman, P. J., and Cherns, D. (1985). An electron-microscope study of hydrous thorium-dioxide ThO2. nH2O. J. Colloid Interf. Sci. 103, 170–177. doi:10.1016/0021-9797(85)90089-x
Fellhauer, D. (2013). Untersuchungen zur Löslichkeit und Redoxchemie von Plutonium und Neptunium. Heidelberg, Germany: Universität Heidelberg.
Grambow, B., Vandenborre, J., Suzuki-Muresan, T., Philippini, V., Abdelouas, A., Deniard, P., et al. (2017). Solubility equilibrium and surface reactivity at solid/liquid interfaces of relevance to disposal of nuclear waste. J. Chem. Thermodyn. 114, 172–181. doi:10.1016/j.jct.2017.05.038
Grenthe, I., Gaona, X., Plyasunov, A. V., Rao, L., Runde, W. H., Grambow, B., et al. (2020). Second update on the chemical thermodynymics of uranium, neptunium, plutonium, americium and technetium. Boulogne-Billancourt, France: OECD Nuclear Energy Agency 14.
Hietanen, S., Paju, J., Lang, W., and Berndt, W. (1954). Studies on the hydrolysis of metal ions. IX. The hydrolysis of the thorium ion, Th4+. Acta Chem. Scand. (Cph). 8, 1626–1642. doi:10.3891/acta.chem.scand.08-1626
Hietanen, S., Sillen, L. G., Fontell, K., Haug, A., Enzell, C., and Francis, G. (1968). Studies on the hydrolysis of metal ions. 60. Hydrolysis of the thorium(IV) ion in a 3 M (Na)Cl medium. Acta Chem. Scand. 22, 265–280. doi:10.3891/acta.chem.scand.22-0265
Holzwarth, U., and Gibson, N. (2011). The Scherrer equation versus the 'Debye-Scherrer equation. Nat. Nanotechnol. 6, 534. doi:10.1038/nnano.2011.145
JCPDS (2001). Joint committee on powder diffraction standards. Swarthmore, USA: Powder Diffraction Files.
Kobayashi, T., Sasaki, T., Takagi, I., and Moriyama, H. (2016). Effect of solid phase transformation on the solubility product of thorium hydrous oxide at 363 K. J. Nucl. Sci. Technol. 53, 1787–1793. doi:10.1080/00223131.2016.1160004
Kraus, K. A., and Holmberg, R. W. (1954). Hydrolytic behavior of metal ions. III. Hydrolysis of thorium(IV). J. Phys. Chem. 58, 325–330. doi:10.1021/j150514a010
Manaud, J., Maynadie, J., Mesbah, A., Hunault, M. O. J. Y., Martin, P. M., Zunino, M., et al. (2020). Hydrothermal conversion of thorium oxalate into ThO2. nH2O. Inorg. Chem. 59, 14954–14966. doi:10.1021/acs.inorgchem.0c01633
Moulder, J. F., Sickle, W. F., Sobol, P. E., and Bomben, K. D. (1995). Handbook of X-ray photoelectron spectroscopy. ULVAC-PHI, Inc; Physical Electronics, Inc.
Neck, V., Altmaier, M., Seibert, A., Yun, J. I., Marquardt, C. M., and Fanghanel, T. (2007). Solubility and redox reactions of Pu(IV) hydrous oxide: Evidence for the formation of PuO2+x(s, hyd). Radiochim. Acta 95, 193–207. doi:10.1524/ract.2007.95.4.193
Neck, V., and Kim, J. I. (2001). Solubility and hydrolysis of tetravalent actinides. Radiochim. Acta 89, 1–16. doi:10.1524/ract.2001.89.1.001
Neck, V., Müller, R., Bouby, M., Altmaier, M., Rothe, J., Denecke, M. A., et al. (2002). Solubility of amorphous Th(IV) hydroxide - application of LIBD to determine the solubility product and EXAFS for aqueous speciation. Radiochim. Acta 90, 485–494. doi:10.1524/ract.2002.90.9-11_2002.485
Nisbet, H., Migdisov, A., Xu, H., Guo, X., van Hinsberg, V., Williams-Jones, A. E., et al. (2018). An experimental study of the solubility and speciation of thorium in chloride-bearing aqueous solutions at temperatures up to 250°C. Geochim. Cosmochim. Acta 239, 363–373. doi:10.1016/j.gca.2018.08.001
Nishikawa, S., Kobayashi, T., Sasaki, T., and Takagi, I. (2018). Solubilities and solubility products of thorium hydroxide under moderate temperature conditions. Radiochim. Acta 106, 655–667. doi:10.1515/ract-2017-2917
Plakhova, T. V., Romanchuk, A. Y., Likhosherstova, D. V., Baranchikov, A. E., Dorovatovskii, P. V., Svetogorov, R. D., et al. (2019). Size effects in nanocrystalline thoria. J. Phys. Chem. C 123, 23167–23176. doi:10.1021/acs.jpcc.9b04379
Rai, D., Felmy, A. R., Sterner, S. M., Moore, D. A., Mason, M. J., and Novak, C. F. (1997). The solubility of Th(IV) and U(IV) hydrous oxides in concentrated NaCl and MgCl2 solutions. Radiochim. Acta. 79, 239–248. doi:10.1524/ract.1997.79.4.239
Rai, D., Moore, D. A., Oakes, C. S., and Yui, M. (2000). Thermodynamic model for the solubility of thorium dioxide in the Na+-Cl--OH--H2O system at 23°C and 90°C. Radiochim. Acta 88, 297–306. doi:10.1524/ract.2000.88.5.297
Rand, M., Fuger, J., Grenthe, I., Neck, V., and Rai, D. (2009). “Chemical thermodynamics vol. 11,” in Chemical thermodynamics of thorium (North Holland, Amsterdam: Elsevier).
Ravel, B., and Newville, M. (2005). ATHENA, ARTEMIS, HEPHAESTUS: Data analysis for X-ray absorption spectroscopy using IFEFFIT. J. Synchrotron Radiat. 12, 537–541. doi:10.1107/s0909049505012719
Romanchuk, A., Trigub, A., Plakhova, T., Kuzenkova, A., Svetogorov, R., Kvashnina, K., et al. (2022). Effective coordination numbers from EXAFS: General approaches for lanthanide and actinide dioxides. J. Synchrotron Radiat. 29, 288–294. doi:10.1107/s160057752101300x
Rothe, J., Altmaier, M., Dardenne, K., Fellhauer, D., Gaona, X., González-Robles Corrales, E., et al. (2019). Fifteen years of radionuclide research at the KIT synchrotron source in the context of the nuclear waste disposal safety case. Geosciences 9, 91. doi:10.3390/geosciences9020091
Rothe, J., Denecke, M. A., Neck, V., Muller, R., and Kim, J. I. (2002). XAFS investigation of the structure of aqueous thorium(IV) species, colloids, and solid thorium(IV) oxide/hydroxide. Inorg. Chem. 41, 249–258. doi:10.1021/ic010579h
Ryan, J. L., and Rai, D. (1987). Thorium(IV) hydrous oxide solubility. Inorg. Chem. 26, 4140–4142. doi:10.1021/ic00271a038
Schindler, P. W. (1967). Heterogeneous equilibria involving oxides hydroxides carbonates and hydroxide carbonates. Adv. Chem. Ser. 67, 196–221.
Vandenborre, J., Grambow, B., and Abdelouas, A. (2010). Discrepancies in thorium oxide solubility values: Study of attachment/detachment processes at the solid/solution interface. Inorg. Chem. 49, 8736–8748. doi:10.1021/ic100756f
Yalcintas, E., Gaona, X., Altmaier, M., Dardenne, K., Polly, R., and Geckeis, H. (2016). Thermodynamic description of Tc(IV) solubility and hydrolysis in dilute to concentrated NaCl, MgCl2 and CaCl2 solutions. Dalton Trans. 45, 8916–8936. doi:10.1039/c6dt00973e
Keywords: thorium, solubility, surface, temperature, thermodynamics, structure
Citation: Kiefer C, Neill T, Cevirim-Papaioannou N, Schild D, Gaona X, Vitova T, Dardenne K, Rothe J, Altmaier M and Geckeis H (2022) Interlink between solubility, structure, surface and thermodynamics in the ThO2(s, hyd)–H2O(l) system. Front. Chem. 10:1042709. doi: 10.3389/fchem.2022.1042709
Received: 12 September 2022; Accepted: 31 October 2022;
Published: 15 November 2022.
Edited by:
Ping Yang, Los Alamos National Laboratory (DOE), United StatesReviewed by:
Xiaofeng Guo, Washington State University, United StatesBuzuayehu Abebe, Adama Science and Technology University, Ethiopia
Hongwu Xu, Arizona State University, United States
Copyright © 2022 Kiefer, Neill, Cevirim-Papaioannou, Schild, Gaona, Vitova, Dardenne, Rothe, Altmaier and Geckeis. This is an open-access article distributed under the terms of the Creative Commons Attribution License (CC BY). The use, distribution or reproduction in other forums is permitted, provided the original author(s) and the copyright owner(s) are credited and that the original publication in this journal is cited, in accordance with accepted academic practice. No use, distribution or reproduction is permitted which does not comply with these terms.
*Correspondence: Christian Kiefer, a2llZmVyQHN1YmF0ZWNoLmluMnAzLmZy; Xavier Gaona, eGF2aWVyLmdhb25hQGtpdC5lZHU=